-
PDF
- Split View
-
Views
-
Cite
Cite
Andrea Sánchez-Vallet, Jeroen R. Mesters, Bart P.H.J. Thomma, The battle for chitin recognition in plant-microbe interactions, FEMS Microbiology Reviews, Volume 39, Issue 2, March 2015, Pages 171–183, https://doi.org/10.1093/femsre/fuu003
- Share Icon Share
Fungal cell walls play dynamic functions in interaction of fungi with their surroundings. In pathogenic fungi, the cell wall is the first structure to make physical contact with host cells. An important structural component of fungal cell walls is chitin, a well-known elicitor of immune responses in plants. Research into chitin perception has sparked since the chitin receptor from rice was cloned nearly a decade ago. Considering the widespread nature of chitin perception in plants, pathogens evidently evolved strategies to overcome detection, including alterations in the composition of cell walls, modification of their carbohydrate chains and secretion of effectors to provide cell wall protection or target host immune responses. Also non-pathogenic fungi contain chitin in their cell walls and are recipients of immune responses. Intriguingly, various mutualists employ chitin-derived signaling molecules to prepare their hosts for the mutualistic relationship. Research on the various types of interactions has revealed different molecular components that play crucial roles and, moreover, that various chitin-binding proteins contain dissimilar chitin-binding domains across species that differ in affinity and specificity. Considering the various strategies from microbes and hosts focused on chitin recognition, it is evident that this carbohydrate plays a central role in plant–fungus interactions.
INTRODUCTION
The fungal cell wall plays a critical role in physiology as, in addition to its structural function in defining cell shape and integrity, it has a dynamic function in interaction of fungi with their surroundings. In pathogenic fungi, the cell wall plays an important role during host invasion. It is the first structure of the pathogen to make physical contact with host cells, which may recognize several of its components as microbe-associated molecular patterns (MAMPs) in order to activate host immune responses (Latgé 2010; Thomma, Nürnberger and Joosten 2011; Latgé and Beauvais 2014). Many potential hosts secrete hydrolytic enzymes, such as chitinases and glucanases, to target fungal cell wall constituents and thus affect fungal cell wall integrity. This may have a dual function for the host as the hydrolytic activity may release MAMP molecules that further stimulate immune responses, but may also lead to fungal cell collapse resulting in arrest of pathogen ingress (Kombrink, Sánchez-Vallet and Thomma 2011). Consequently, fungal pathogens evolved various strategies to protect their cell walls and prevent the elicitation of cell wall-triggered immune responses in their hosts. For instance, modification of the composition and structure of cell walls to mask substrates for host hydrolytic enzymes and potential MAMPs is a successful strategy that is employed by some pathogens (Fujikawa et al., 2012; Latgé and Beauvais 2014). Furthermore, pathogens evolved to secrete effector molecules, many of which have been characterized to deregulate host physiology in order to support infection (de Jonge, Bolton and Thomma 2011; Rovenich, Boshoven and Thomma 2014). In pathogenic fungi, various types of effectors have been characterized that either protect their cell walls or prevent or perturb the elicitation of cell wall-triggered host immune responses (Kombrink et al., 2011; Rovenich et al., 2014).
Especially the role of chitin in interactions of pathogenic fungi and host plants has received considerable attention as it has been known for decades that plant hosts respond to chitin application by inducing immune responses (Felix, Regenass and Boller 1993; Shibuya et al., 1993). Research into the molecular mechanism of chitin perception and chitin-triggered immunity in plants has really sparked since the cloning of the first plant chitin receptor from rice nearly a decade ago (Kaku et al., 2006).
CHITIN BIOSYNTHESIS
Chitin is a linear homopolymer of β-(1,4)-linked N-acetyl-D-glucosamine (GlcNAc) monomers and, after cellulose, the second most abundant polysaccharide in nature. In addition to the exoskeleton of arthropods and the egg-shell of nematodes, chitin occurs as an important structural component in the cell walls of fungi. In these cell walls, chains of chitin associate in microfibrils that are covalently bound to the major component of fungal cell walls, β-(1,3)-glucan, and form a network together with glycoproteins to constitute the structural basis that confers stiffness to the cell wall. Additional components of fungal cell walls are other proteins and carbohydrates such as mannans, β-(1,6)-glucan and α-(1,3)-glucan that form part of the soluble matrix (Free 2013; Latgé and Beauvais 2014).
Fungal cell wall synthesis requires considerable energy and is therefore strictly regulated. Chitin is synthesized by plasma membrane-localized chitin synthases (CHSs), which are β-glycosyltransferases that use cytoplasmic UDP-N-acetylglucosamine as a substrate (Bracker, Ruiz-Herrera and Bartnicki-Garcia 1976; Leal-Morales, Bracker and Bartnicki-Garcia 1994; Sietsma et al., 1996). During chitin synthesis, linear chitin polymers are elongated towards the extracellular space by the addition of N-acetylglucosamine units to the non-reducing end of the chain. Antiparallel chitin polymers are subsequently connected through hydrogen bonds, and thus assemble into microfibrils that have high tensile strength to confer cell wall rigidity. In spite of its relatively simple structure, chitin biosynthesis is an intricate process, most probably due to the need of chitin at several stages of fungal development and the complexity of its regulation (Lenardon, Munro and Gow 2010). Consequently, the synthesis of chitin is still not completely understood and has only been characterized for a few fungal species, including the yeasts Saccharomyces cerevisiae and Schizosaccharomyces pombe (Cabib et al., 2001; Matsuo et al., 2004). Saccharomyces cerevisiae contains three CHSs that have cell cycle-specific functions. While CHS1 has a repair function, CHS2 is responsible for the chitin formation in the septum that is formed in between daughter cells during cytokinesis (Silverman et al., 1988). CHS3 is also involved in septum formation and, in addition, is responsible for the synthesis of cell wall chitin (Kollár et al., 1995; Cabib et al., 2001).
Filamentous fungi generally contain a higher number of CHSs (up to 10) than yeasts, which can be attributed to their multicellular organization, the higher complexity of their life cycle and the higher chitin content in their cell walls (Lenardon et al., 2010). Based on their amino acid sequence, CHSs are grouped in two families, and furthermore in five or six classes (Chigira et al., 2002; Roncero 2002; Choquer et al., 2004). However, this classification is somewhat controversial because the function of a CHS cannot be inferred from the class to which it belongs (Lenardon et al., 2010). For example, class I CHSs of Aspergillus nidulans and of Neurospora crassa have major functions in hyphal growth and conidiation (Yarden and Yanofsky 1991; Borgia et al., 1996), while a mutant in the homologous CHS of the rice blast pathogen Magnaporthe oryzae is only impaired in the formation of conidia (Kong et al. 2012). In contrast, a mutant in the homologous CHS of the maize smut pathogen Ustilago maydis has no obvious phenotype at all, and therefore seems to play a minor role in chitin synthesis during development or to have a redundant function with other CHSs (Weber et al. 2006). Thus, despite the classification system, CHSs need to be individually studied to be assigned a particular function.
In N. crassa, there are seven CHSs, three of which were shown to play a major role in cell wall biogenesis at the tip of growing hyphae and in septa (Riquelme et al., 2007; Sánchez-León et al., 2011). Aspergillus nidulans has eight CHSs that differentially contribute to chitin synthesis during the different phases of hyphal development. While some of them have major functions in chitin formation during hyphal growth, others are involved in the synthesis of chitin during conidiation or stress responses (Ichinomiya et al., 2005; Fukuda et al., 2009). Finally, CHSs of A. fumigatus are functionally redundant and collaborate to synthesize chitin (Muszkieta et al., 2014).
The role of CHSs in pathogenicity has been analyzed for a number of plant pathogenic fungi, and CHSs often play roles in the infection. However, their contribution varies depending on the fungal species. In the gray mold fungus Botrytis cinerea, eight CHSs have been identified, of which four were implicated in virulence (Soulie et al., 2003, 2006; Morcx et al., 2013). In Colletotrichum graminicola, the causal agent of cereal anthracnose, treatment with the CHS inhibitor nikkomycin Z disrupted the formation of appressoria and, consequently, progress of the infection. In accordance with this observation, a mutant in one of the ten CHSs of C. graminicola showed cell wall defects and appressorium distortion, leading to reduced pathogenicity (Werner et al., 2007). A deletion strain in one of the seven CHS genes of M. oryzae is similarly defective in appressorial cell wall synthesis, leading to reduced host penetration. Furthermore, mutants in two additional M. oryzae CHS genes were impaired in virulence (Kong et al., 2012). The vascular wilt fungus Fusarium oxysporum encodes seven CHSs, the deletion of one of which was impaired in virulence most probably because of its enhanced hypersensitivity to antimicrobials and the elicitation of plant resistance (Madrid, Di Pietro and Roncero 2003; Pareja-Jaime et al., 2010). Deletion of all of the remaining CHSs but one also compromised virulence (Martin-Udiroz, Madrid and Roncero 2004, 2008). The genome of the dimorphic fungus U. maydis encodes eight CHSs, which are localized in septa, and three of them were additionally localized in growing hyphal tips and yeast buds. Interestingly, deletion of each CHS gene compromised pathogenesis, of which three were shown to be required for tumor formation (Weber et al., 2006; Treitschke et al., 2010).
In conclusion, fungal cell walls are dynamic and vary in composition and structure between as well as within species, during different phases of development (Gow and Hube 2012; Free 2013). The correct assembly of chitin polymers in cell walls in filamentous fungi therefore requires several CHSs with tightly regulated expression and localization patterns. Although functional redundancy may occur to some extent, different CHSs act differentially in cell wall integrity, fungal growth, protection against environmental stress and, in pathogenic species, to pathogenicity. However, cooperativity is generally required for cell wall synthesis.
CHITIN PERCEPTION SYSTEMS IN PLANT HOSTS
Like other organisms, also plants evolved to recognize MAMPs by cell surface localized pattern-recognition receptors (PRRs) to mount an immune response. The best-studied plant PRRs are the sensors for bacterial flagellin (FLS2) and elongation factor Tu (EFR), respectively (Gómez-Gómez, Bauer and Boller 2001; Zipfel et al., 2006). These are transmembrane proteins that carry extracellular leucine-rich repeats (LRRs) and a cytoplasmic kinase domain. However, although LRR-type PRRs are the most studied, PRRs may also carry other extracellular domains than LRRs to perceive microbial ligands (Antolín-Llovera et al., 2012). For instance, plant receptors for fungal chitin and bacterial peptidoglycan contain extracellular lysin motifs (LysMs) that were initially discovered in peptidoglycan-hydrolyzing enzymes (Bateman and Bycroft 2000; Kaku et al., 2006; Miya et al., 2007; Wan et al., 2008; Willmann et al., 2011).
The first chitin immune receptor gene that was cloned was the gene encoding the rice chitin elicitor-binding protein (CEBiP), a cell surface localized receptor with extracellular LysMs and that lacks a cytoplasmic signaling domain such as a kinase (Kaku et al., 2006). CEBiP is essential for chitin recognition and indispensable for the induction of chitin-triggered immunity (Kaku et al., 2006). More recently, it was confirmed that CEBiP directly binds chitin and acts as a genuine chitin receptor (Hayafune et al., 2014; Kouzai et al., 2014b). It was demonstrated that CEBiP preferentially binds longer chain chitin oligosaccharides, such as heptamer-octamer, and it was proposed that a single chitin molecule will establish CEBiP homodimerization as the ectodomain of two CEBiP monomers bind to the same chitin oligosaccharides from the opposite side (Hayafune et al., 2014) (Fig. 1). The CEBiP dimer likely recruits the LysM domain containing receptor kinase OsCERK1 (Oryza sativa chitin elicitor receptor kinase1) that is subsequently phosphorylated and thus activates a chitin-triggered immune response (Shimizu et al., 2010; Hayafune et al., 2014). In this process, OsCERK1 phosphorylates the guanine nucleotide exchange factor OsRacGEF1 that interacts with, and activates, the small GTPase OsRAC1 (Akamatsu et al., 2013; Fig. 1). In turn, OsRAC1 interacts with several downstream signaling proteins and regulates the final steps of the signaling pathway, including the production of reactive oxygen species, phytoalexins, lignins, and the activation of MAPK cascades and expression of pathogenesis-related proteins (Wong et al., 2007; Akamatsu et al., 2013). Other PRRs, such as OsFLS2, also interact with OsRacGEF1, suggesting that these components act in a common signaling pathway downstream of different MAMP receptors in rice (Akamatsu et al., 2013).
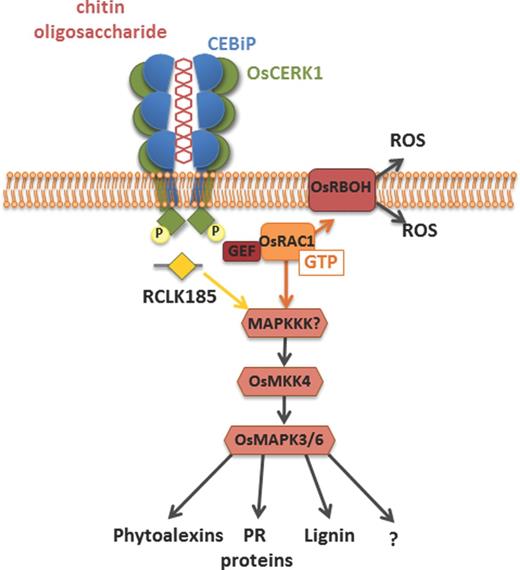
Schematic representation of the chitin receptor complex at the plasma membrane and components involved in the cellular signaling pathway in rice. The LysM receptor CEBiP directly binds chitin, resulting in CEBiP homodimerization as the ectodomain of two CEBiP monomers bind the same chitin oligosaccharide from opposite sides. CEBiP furthermore heterodimerizes with OsCERK1 whose kinase domain is subsequently phosphorylated. In turn, OsCERK1 phosphorylates the downstream signaling component OsRacGEF1, which activates OsRAC1 that then activates a MAPK cascade, which culminates in the expression of defense executers. OsCERK1 can also phosphorylate RCLK185 that activates the same MAPK cascade.
In addition, other components of chitin-triggered immunity in rice have been described. The plasma membrane-localized receptor-like kinase (RLK) OsRLCK185 associates with, and is phosphorylated by, OsCERK1 in response to chitin (Yamaguchi et al., 2013). Similarly, the RLK OsRLCK176 was also shown to heterodimerize with OsCERK1 to mediate chitin-induced signaling (Ao et al., 2014). Potentially, these RLKs constitute additional links between the PRR and the MAPK cascades (Yamaguchi et al., 2013; Ao et al., 2014). Finally, two additional homologs of CEBiP, OsLYP4 and OsLYP6, were reported to bind chitin, heterodimerize with OsCERK1 and act in chitin-triggered immune responses and disease resistance (Liu et al., 2012a; Ao et al., 2014). Interestingly, OsRLCK176, OsLYP4, OsLYP6 and OsCERK1 do not exclusively respond to chitin, but have also been implicated in peptidoglycan responses. Therefore, OsCERK1 most probably acts as an adaptor for signal transduction of multiple PRRs and is not solely involved in chitin-triggered immunity (Ao et al., 2014; Kouzai et al., 2014a).
Although chitin perception is probably most intensively characterized in rice, chitin detection has also been intensively studied in Arabidopsis. Five LysM RLKs are encoded by the Arabidopsis genome, of which only a single one was implicated in chitin detection and named AtCERK1 for chitin elicitor receptor kinase 1 or LysM RLK1 (Miya et al., 2007; Wan et al., 2008). Subsequent analysis has shown that AtCERK1 can bind chitin, although its affinity for chitin binding appears to be rather low, up to 68 μM for chitin oligomers of eight units of GlcNAc (Iizasa, Mitsutomi and Nagano 2010; Petutschnig et al., 2010; Liu et al., 2012b). It was suggested that chitin oligomers are bound by the LysM domains of two CERK1 monomers, resulting in receptor dimerization and transphosphorylation of their cytoplasmic kinase domains (Liu et al., 2012b). This dimerization is established by relatively long (oligomers containing eight GlcNAc monomers) and not by shorter (oligomers containing four or five GlcNAc monomers) oligomers, despite the finding that these were previously shown to also induce chitin-triggered responses in tomato (Felix et al., 1993; Liu et al., 2012b). Furthermore, it has been reported that the LysM RLK AtLYK4 is required for chitin-triggered immunity and may form part of the chitin receptor complex (Wan et al., 2012). Additional components of the chitin receptor complex include LIK1 (LysM RLK1-interacting kinase 1) that interacts with CERK1 and regulates the triggered innate immunity (Le et al., 2014). CERK1 phosphorylates downstream signaling proteins, such as the mitogen-activated protein kinases MPK3 and MPK6 (Miya et al., 2007).
Despite the allocation of considerable research efforts, the chitin perception complex of Arabidopsis is still associated with considerable controversy, and it has been claimed that chitin perception systems differ between rice and Arabidopsis (Shinya et al., 2012). Only one of three CEBiP homologs of Arabidopsis, AtCEBiP (LYM2), has been reported to display high-affinity binding of chitin oligosaccharides. However surprisingly, LYM2 was not found to contribute to chitin-triggered immune signaling, as single and triple knock-outs of Arabidopsis CEBiP homologs and a line overexpressing LYM2 responded to chitin in similar way as wild-type plants (Shinya et al., 2012). However, it was subsequently demonstrated that LYM2 mediates a reduction in the flux of small molecules via plasmodesmata that is triggered upon chitin recognition, resulting in defense against B. cinerea (Faulkner et al., 2013). The observation that chitin-induced plasmodesmatal flux changes operate in a CERK1-independent manner suggests the operation of CERK1-dependent and -independent chitin-triggered defense responses (Faulkner et al., 2013).
Recently, it was proposed that AtLYK5, a LysM-containing cell surface receptor that carries an inactive kinase domain, rather than AtCERK1, is the primary chitin receptor of Arabidopsis. This hypothesis is supported by the respective affinities for chitin binding. Whereas the affinity of AtCERK1 for chitooctaose binding is quite low (kd = 45 μM), AtLYK5 binds chitooctaose with a significantly higher affinity (kd = 1.71 μM). AtLYK5 forms a chitin-inducible complex with AtCERK1 leading to AtCERK1 phosphorylation and induction of chitin-triggered immune responses (Cao et al., 2014). However, whereas AtLYK5 mutation results in significantly compromised chitin signaling, complete loss of chitin-triggered immunity could only be obtained upon simultaneous mutation of AtLYK4 and AtLYK5 (Cao et al., 2014). This finding argues for a role of AtCERK1 as an adaptor for cellular signal transduction rather than as major chitin receptor. Such a role as adaptor for PRRs, as similarly proposed for OsCERK1, also accommodates several reports that have demonstrated functions of AtCERK1 in processes other than chitin signaling, including bacterial immunity and peptidoglycan perception (Gimenez-Ibanez et al., 2009, Gimenez-Ibanez, Ntoukakis and Rathjen 2009; Willmann et al., 2011; Petutschnig et al., 2014). These recent findings would argue that chitin perception systems of rice and Arabidopsis are not so different after all.
PATHOGEN STRATEGIES TO PERTURB CHITIN HYDROLYSIS AND DETECTION
Many pathogens establish their first contact with plant cells in the apoplast, the extracellular space in plant tissue that constitutes a source of nutrients and shelter for many microbial inhabitants. At the same time the apoplast is a hostile environment that contains hydrolytic enzymes and toxins that may challenge microbial growth. Furthermore, host hydrolytic activities establish decomposition of microbial matrices to generate soluble PRR ligands (Liu et al., 2014). For instance, apoplastic glucanases and chitinases disrupt the integrity of fungal walls and release chitin and glucan MAMPs. In response, several strategies evolved in plant pathogens in order to prevent recognition and MAMP-triggered activation of immune responses, including alterations in the composition and structure of cell walls, modification of carbohydrate chains and secretion of effectors to provide protection to the cell wall or target host immune responses (Fig. 2). Conversion of chitin to chitosan by deacetylation during host invasion may protect hyphae of pathogenic fungi from being hydrolyzed by extracellular plant chitinases, as chitosan is a poor substrate for chitinases, and consequently will reduce the release of elicitors (Ride and Barber 1990). Moreover, chitosan is a weak inducer of immune responses in many plant species (Baureithel, Felix and Boller 1994; Vander et al., 1998), although it has been reported as a strong inducer of immunity in others (Rabea et al., 2003; Iriti and Faoro 2009). Indeed, it has been demonstrated that chitin in the invasive hyphae of the rust fungi Puccinia graminis f.sp. tritici and Uromyces fabae and of the anthracnose fungus C. graminicola is not accessible to the chitin-binding probe wheat germ agglutinin, but is labeled by a chitosan-specific antibody (El Gueddari et al., 2002). In addition to chitin deacetylation, also other changes in cell wall composition may occur during fungal infection. For instance, the rice blast pathogen M. oryzae specifically accumulates α-1,3-glucan on the surface of the cell wall of infectious hyphae during plant invasion. The accumulation of α-1,3-glucan on the cell wall of M. oryzae is indispensable for successful invasion of rice, as mutants with reduced levels of α-1,3-glucan or treatment with α-1,3-glucanase led to enhanced susceptibility towards chitinases, triggered a faster immune response in plants and, consequently, were less infectious. Thus, α-1,3-glucan protects the fungal cell wall from antimicrobials, including chitinases, delays the release of MAMPs and, consequently, host defense responses (Fujikawa et al., 2009, 2012). Similar phenomena have been observed in mammalian pathogens, as deletion of α-1,3-glucan synthase genes in A. fumigatus resulted in reduction of fungal virulence despite normal growth and development in vitro, which was attributed to increased exposure of polysaccharide MAMPs such as chitin and β-1,3-glucan (Beauvais et al., 2013). Likewise, the lack of α-1,3-glucan in Histoplasma capsulatum resulted in exposure of MAMPs and reduced pathogenicity (Rappleye, Eissenberg and Goldman 2007).
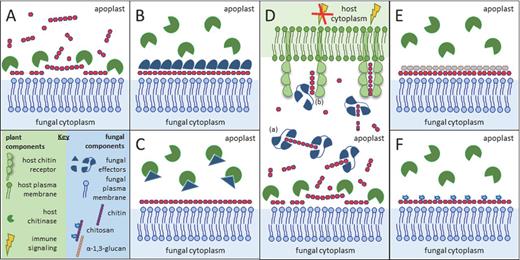
Simplified illustration of potential pathogen strategies to perturb hydrolysis of cell wall chitin and detection of chitin oligomers. (A) Chitin in fungal cell walls is targeted by host-secreted chitinases that liberate chitin fragments that can further activate the host immune system, and that can hydrolyze fungal cells together with other host-secreted hydrolases. (B) Several fungal pathogens are known to secrete effectors that can shield fungal hyphae and thus prevent access of chitinases to the chitin in the cell wall. (C) Fungal pathogens may secrete effectors that directly target chitinases to inhibit their activity. (D) Fungal LysM effectors prevent host recognition of released chitin oligomers, which can be attributed to two mechanisms. Due to their ultrahigh affinity chitin binding, these effectors may either scavenge chitin fragments such that they cannot activate host chitin receptors. Alternatively, they may prevent activation of the chitin receptor complex by preventing chitin-induced receptor dimerization. (E) Cell wall remodeling may lead to reduced access of chitinases to the chitin in the cell wall. Several fungi accumulate α-1,3-glucan at the surface of the cell wall to prevent degradation of chitin by chitinases. (F) Alternatively, during host colonization some fungi convert cell wall chitin into chitosan, which is a poor substrate for chitinases and a weak inducer of host immune responses.
Masking of cell wall polysaccharides may also be established by pathogen-secreted proteinaceous components known as effectors (Fig. 2). For instance, the tomato leaf mold fungus Cladosporium fulvum secretes the Avr4 effector during infection of host plants. Avr4 contains an invertebrate chitin-binding domain (ChBD) (carbohydrate-binding module family 14, CBM14) and binds fungal cell wall chitin to reduce its accessibility to host chitinases, thus preventing cell wall hydrolysis (van den Burg et al., 2006; van Esse et al., 2007). Avr4 homologs occur in a number of relatives of C. fulvum, and the homolog of Mycosphaerella fijiensis that causes black Sigatoka disease of banana was shown to similarly protect fungal cell walls against hydrolysis by plant chitinases (Stergiopoulos et al., 2010). A more broadly distributed group of chitin-binding effector proteins in the fungal kingdom contains LysM domains and are termed LysM effectors. Like Avr4, these LysM effectors were originally identified in C. fulvum, but have been thoroughly characterized in several fungal species, including M. oryzae and the wheat blotch pathogen Zymoseptoria tritici (formerly M. graminicola) (Bolton et al., 2008; Kombrink and Thomma 2013). In all these pathogens, LysM effectors are highly expressed during infection, accumulate in the apoplastic space of infected plants, and were demonstrated to be essential for pathogen virulence through suppression of chitin-triggered immunity (de Jonge et al., 2010; Marshall et al., 2011; Mentlak et al., 2012; Chen et al., 2014). One of the molecular mechanisms by which the C. fulvum LysM effector Ecp6 (extracellular protein 6) can suppress chitin-triggered immunity was revealed by its crystal structure (Fig. 3; Sánchez-Vallet et al., 2013). Two of the three LysM domains of Ecp6 have the capacity to undergo ligand-induced dimerization, resulting in a deeply buried groove that binds chitin oligosaccharides with ultrahigh (pM) affinity that is sufficient to outcompete host immune receptors for chitin binding (Sánchez-Vallet et al., 2013). In this manner, chitin-triggered immunity is not induced and the fungus can colonize the apoplast (Sánchez-Vallet et al., 2013). However, the second, singular, LysM of Ecp6 that has no possibility to undergo intramolecular dimerization, and thus likely lacks the ability to outcompete host receptors for chitin binding, similarly has the capacity to suppress chitin-triggered immunity (Sánchez-Vallet et al., 2013). This observation strongly suggests that MAMP sequestration in order to deprive host immune receptors of their ligand is not the main function of this effector (Sánchez-Vallet et al., 2013). Potentially, Ecp6 interferes in the activation of chitin immune receptor complexes through preventing receptor dimerization (Kombrink and Thomma 2013; Sánchez-Vallet et al., 2013).

3D structure of the C. fulvum LysM effector Ecp6. (A) In the crystal structure, two LysM domains (LysM1 in green and LysM3 in light blue) of Ecp6 were found to cooperatively bind a chitin oligomer (chitin tetramer represented with red sticks) with ultrahigh affinity. (B) Representation of the crystal structure of Ecp6 rotated 90 degrees around the horizontal axis when compared with panel A to show the chitin-binding site of LysM2 (in dark blue). The singular LysM domain has the capacity to bind chitin, albeit with lower affinity than the composite LysM1–LysM3 binding groove. Since chitin bound to LysM2 was not observed in the crystal structure, a chitin tetramer is represented by transparent red sticks.
Intriguingly, two LysM effectors of Z. tritici, Mg1LysM and Mg3LysM that have one and three LysM domains, respectively, display an additional molecular function. Despite being related to C. fulvum and M. fijiensis, Z. tritici pathogen does not contain an Avr4 homolog that can protect its hyphae against hydrolysis by host chitinases. It is therefore perhaps not surprising that its LysM effectors evolved to exert this activity (Marshall et al., 2011). The molecular mechanism by which Mg1LysM and Mg3LysM are able to protect fungal cell walls against chitinases presently remains elusive. Potentially, they oligomerize in the presence of chitin to cover fungal hyphae and prevent access of chitinases to the chitin in the cell wall. However, direct inhibition of chitinases cannot be discarded as well, as Mg1LysM and Mg3LysM might interact with chitinases either directly or indirectly through binding to chitinase-bound chitin molecules to prevent hydrolysis. In fact, it has been proposed that direct inhibition of host chitinases is a common strategy of microbial pathogens to overcome host immune responses. In several dicots, class I chitinases are subject to positive selection and rapid evolution, specifically in the active site cleft. This finding suggests that fungi directly defend themselves against chitinolytic activity through enzymatic inhibition or other forms of chemical resistance against the class I chitinases (Bishop, Dean and Mitchell-Olds 1999).
Considering the importance of tackling host immune responses centered on cell wall chitin, it is conceivable that several strategies evolved in fungi. Thus far, LysM effectors have not been identified in obligate biotrophic fungal plant pathogens, such as rusts and powdery mildews. However, the rust transfer protein 1 from U. fabae and U. striatus is capable of forming filamentous structures that were suggested to have a potential role in protection of fungal hyphae from degradation (Kemen et al., 2013). In addition, interference with degradation of cell wall components other than chitin has been described in several fungal pathogens. Fusarium verticillioides secretes Fumonisin B1, a toxin that inhibits the activity of maize β-1,3-glucanase (Sánchez-Rangel, Sánchez-Nieto and Plasencia 2012). Several effectors have been described to inhibit host hydrolytic enzymes, such as Avr2 and Pit2 from C. fulvum and U. maydis, respectively, that inhibit host proteases (Rooney et al., 2005; van Esse et al., 2008; van der Linde et al., 2012; Mueller et al., 2013). In a similar way, some fungal effectors may inhibit host enzymes that degrade cell wall components, such as chitinases.
CHITIN-TRIGGERED IMMUNITY AND NON-PATHOGENIC MICROBES
Similar to pathogens, commensalistic endophytes and mutualists develop intimate host plant associations. During initiation of such symbiotic interactions, immune receptors continue to perceive MAMPs to intercept potential pathogens (Jacobs et al., 2011). Consequently, similar to pathogens, endophytes and mutualists are recipients of immune responses. However, the precise role of host immunity in the establishment of symbiosis has long remained enigmatic. An increasing body of evidence suggests that also these microbes employ effectors to suppress host immune responses rather than avoid MAMP detection (Rovenich et al., 2014). The overlap in the transcription profile of plants invaded by pathogens or symbionts indicates common host mechanisms to interact with these invaders (Güimil et al., 2005). In Medicago truncatula roots, chitinases are expressed during the interaction of mycorrhiza and fungal pathogens (Salzer et al., 2000). Consequently, the virulence factors secreted by the fungi, and the mechanism by which plants tolerate them, will define the outcome of their interactions. Several studies have provided evidence for the role of effectors in symbiotic interactions. The MiSSP7 effector of the ectomycorrhizal fungus Laccaria bicolor interferes with jasmonic acid-mediated immune signaling (Plett et al., 2014), while the SP7 effectors of the arbuscular mycorrhiza Rhizophagus irregularis interacts with ERF19 and attenuates ethylene-mediated immune responses (Kloppholz, Kuhn and Requena 2011). The genome of the endophytic fungus Piriformospora indica expresses a high number of effector-like small secreted proteins upon interaction with host plants (Zuccaro et al., 2011). Interestingly, carbohydrate-binding domain-containing proteins, and more specifically LysMs, are overrepresented (Zuccaro et al., 2011). These LysM-containing proteins could be involved in chitin metabolism, or could even act as LysM effectors that interfere with chitin-triggered immunity. The R. irregularis genome similarly encodes effector-like proteins, including LysM proteins, putative chitinases and putative CHSs that are considerably upregulated in mycorrhizal roots (Salzer et al., 2000; Tisserant et al., 2013). Altogether, it seems like mutualistic fungi undergo cell wall remodeling during intracellular colonization (Balestrini and Bonfante 2014) in order to adapt to the new growing conditions and protect themselves against the host immune system.
The molecular mechanisms by which the symbiotic interactions are established get even more intriguing by the fact that chitin-derived molecules are microbial signatures that not only trigger defense responses but also symbiotic responses in plants. Arbuscular mycorrhiza secrete Myc factors which are essential for their recognition by the host plant. In R. irregularis, these molecular signals have been recently characterized as a mixture of sulfated and non-sulfated simple lipochitooligosaccharides (LCOs), which stimulate formation of mycorrhizal arbuscules in host cells of diverse plant species (Maillet et al., 2011). Interestingly, chitin-containing symbiosis signaling molecules are not only produced by mycorrhiza, as also Nodulation (Nod) factors produced by nitrogen-fixing rhizobacteria are LCOs that are recognized by leguminous host plants (Dénarié, Debellé and Promé 1996). Additionally, short chitin oligomers of four or five units that are not immunogenically active and are produced by mycorrhizal fungi activate Ca2+ spiking, a typical symbiosis response in root epidermal cells (Walker, Viprey and Downie 2000). Therefore, LCOs and short chitin oligomers are part of the signaling molecule repertoire that activates the common symbiotic signaling pathway, although the precise role of the different molecules is not yet clear (Walker et al., 2000; Genre et al., 2013). Modification of chitin oligomers or expression of specific chitinases that will degrade chitin molecules during symbiosis could lead to different host responses, namely the activation of either symbiosis or immunity (Genre et al., 2013). Consequently, the capacity to identify chitin decorations and the length of the oligomer is the basis for plant hosts to discriminate symbionts from pathogens (Maillet et al., 2011; Broghammer et al., 2012). However, the molecular mechanism by which this is achieved remains obscure. It might be that this recognition capacity is broadly occurring in the plant kingdom, as non-legume plants were shown to respond to Nod factors and suppress innate immune responses (Liang et al., 2013). Intriguingly, rice OsCERK1 was recently shown to be essential not only for chitin-triggered immunity but also for arbuscular mycorrhizal symbiosis, as no internal hyphae or arbuscules were formed by R. irregularis in the mutant oscerk1 (Miyata et al., 2014). Thus, OsCERK1 has a dual function in symbiosis and immunity, which again indicates that this protein acts as an adaptor in multiple receptor complexes, rather than as a receptor for a specific ligand.
Like chitin receptors, Nod factor receptors (NFRs) of leguminous plants are LysM receptors that directly bind their substrates; i.e. chitin-derivative Nod factors (Limpens et al., 2003; Madsen et al., 2003; Radutoiu et al., 2003; Broghammer et al., 2012). As described for LysM-containing immune receptors, also NFRs are organized in receptor complexes. In Lotus japonicus, this complex comprises NFR1, which is autophosphorylated in vivo and heterodimerizes with NFR5 in order to activate downstream signaling (Radutoiu et al., 2003; Madsen et al., 2011). Expression of L. japonicus NFR1 and NFR5 in M. truncatula and L. filicaulis extended the host range of these plants to include bacterial strains that normally infect L. japonicus, and domain swaps between L. japonicus and L. filicaulis NFR1 and NFR5 revealed that the LysM domains of these receptors mediate perception of bacterial Nod-factor signals depending on their structure (Radutoiu et al., 2003). Moreover, discrimination of two rhizobial strains that produce different Nod factors could be attributed to a single amino acid in LysM2 of NFR5 (Radutoiu et al., 2003). Interestingly, Arabidopsisatcerk1 mutants expressing chimeric receptors composed of the LysM domain-containing ectodomain of L. japonicus NFRs and the kinase domain of AtCERK1 induced an immune response and pathogen resistance upon treatment with Nod factors and not with chitin oligomers (Wang, Xie and Staehelin 2014). Similarly, the ectodomains of NFRs were replaced by those of OsCERK1 and OsCEBiP and expressed in L. japonicus NFR mutants, leading to nodulation signaling upon chitin treatment. Hence, the ectodomains of LysM domain receptors provide a way to trigger specific downstream signaling (Wang et al., 2014). It has been shown that L. japonicus LysM receptors LjNFR1 (NFR1) and LjNFR5 directly bind Nod factors with nanomolar affinity, while they have a much lower affinity for chitin oligomers (Broghammer et al., 2012). Furthermore, a single LysM domain of LjNFR5 binds stronger to the Nod factor from Mesorhizobium loti in vitro than to chitin oligosaccharides (Sørensen et al., 2014). Thus, LysM domains of leguminous plant receptors have evolved to specifically recognize differences in decorated chitin ligands.
Thus far, it seems that LysMs generally have at least some affinity for chitin and/or peptidoglycan derivatives. This finding argues for the careful determination dissociation constants (kd) values in order to determine genuine LysM ligands (Broghammer et al., 2012; Sørensen et al., 2014). Further to this consideration, care should be taken with the interpretation of especially in vivo experiments where ligand concentrations typically cannot be controlled.
STRUCTURAL BASES FOR CHITIN BINDING
In principle, chitin binding by proteins serves various purposes such as its biosynthesis, modification, hydrolysis, detection, preservation and sequestration. Associated with these processes, different 3D folds are observed as documented in the CAZy carbohydrate-active enzymes database (Lombard et al., 2014) and the PBD protein database (Berman et al., 2000), likely exhibiting different affinities that fit the different purposes. Consequently, from both a structural and functional viewpoint, there is not one ubiquitous but rather several dissimilar ChBD found across living species and viruses. Searching the SUPERFAMILY database (Wilson et al., 2009) for the keyword ‘chitin-binding domain’ exposes three different protein folds; the small invertebrate chitin-binding protein fold (such as tachycitin; Suetake et al., 2000), the all beta immunoglobulin-like beta-sandwich protein fold (CBP21; Vaaje-Kolstad et al., 2005) and finally the WW domain-like protein fold (ChBD of chitinase A1; Ikegami et al., 2000). Using the keyword ‘chitin binding’ adds the alpha and beta class (a/b class) 7-stranded beta/alpha barrel protein fold (chitin deacetylase; Blair et al., 2006) to the three previous folds. In the results of both queries, omitted are the LysM domain fold (Ecp6; Sánchez-Vallet et al., 2013) belonging to the alpha and beta class of protein folds (a+b class, as opposed to the a/b class of chitin deacetylase) and the knottin fold (such as hevein; Aboitiz et al., 2004) comprising small inhibitors, toxins and lectins. Hevein is a member of the CAZy database (Lombard et al., 2014) carbohydrate-binding module family 18 (CBM18). These cystin-rich modules are found attached to a number of chitinase catalytic domains and in non-catalytic proteins, where they are found either in isolation or as multiple repeats. The NMR structure of a truncated hevein in complex with the chitooligosaccharide triacetylchitotriose (PDB ID 1T0M; Aboitiz et al., 2004) is shown in Fig. 4, panel (A). The most prominent feature of the interaction between triacetylchitotriose (millimolar affinity range; micromolar affinity range for hexaacetylchitotriose) (Aboitiz et al., 2004) and the polypeptide are two face-to-face interactions formed by the faces of two tryptophan side chains (W21 and W23) on the one hand and the faces two adjacent GlcNAc monomers on the other hand. The knottin fold is not only observed in plant proteins such as hevein (CBM18) but also found, for example, in the antifungal protein tachycitin (CBM14) from the horseshoe crab Tachypleus tridentatus or the C. fulvum avirulence protein Avr4 (CBM14) and thus recognized as a common ChBD fold shared amongst bacteria, eukaryote and viruses (Suetake et al., 2000; van den Burg et al., 2006). The main feature, the face-to-face stacking interactions as delineated above, is not exclusively restricted to tryptophan side chains as tyrosine and phenylalanine side chains are equivalently functionalized in chitin-binding proteins (as discussed below). No apparent parallels, neither fold- nor interaction-wise (face-to-face stacking), are present between the above-mentioned ChBDs and the so-called chitinase insertion domain CID (FKBP-like fold; Li and Greene 2010), as included in certain TIM barrel chitinases of the family 18 glycoside hydrolases. The CID domain is proposed to both help orient and bind chitin by forming several hydrogen bonds with the substrate. Compared to hevein, the three LysM modules (LysM1, 2 and 3) of C. fulvum Ecp6 are equally compact and contain at least one disulphide bond but feature a different fold, and hence constitute an independent carbohydrate-binding module family, CBM50. LysM chitin affinity is not reliant on face-to-face type of stacking interactions with aromatic side chains but, remarkably, chitin affinity dependents on LysM domain cooperativity (Figs 3 and 4, panels B and C) (PDB ID 4B8V; Sánchez-Vallet et al., 2013). The groove of one LysM domain (Fig. 4, panel B) is able to bury one half of a bound saccharide monomer of short soluble chitin fragments, leaving the possibility for another LysM domain to approach from the other side to bind to the other half of the bound (i.e. half sandwiched) saccharide monomer. Indeed, Ecp6 LysM1 and LysM3 cooperate to form a dimer with a deeply buried chitin-binding groove (Figs 3 and 4, panel C), thereby fully covering the substrate and achieving chitin binding with picomolar affinity (Sánchez-Vallet et al., 2013). This type of cooperativity (i.e. fully sandwiched substrate) is different from the one described for the bacterial Bacillus subtilis peptidoglycan-hydrolyzing NlpC/P60 endopeptidase (Wong et al., 2014). Here, the four LysM domains of the endopeptidase act additively to increase the binding affinity through side-by-side binding to one half of bound saccharide monomers (half sandwiched) and, consequently, the enzyme is unable to discriminate between chitin and peptidoglycan (alternating units of GlcNAc and N-acetylmuramic acid; see also Mesnage et al., 2014) fragments. Besides the latter two MAMPs, certain receptor LysM domains are responsible for the high affinity binding of Nod factors (that have a GlcNAc backbone) as recently shown for the L. japonicus NFR 5 LysM2 domain (Sørensen et al., 2014). LysM2 of Ecp6 displays a low micromolar affinity for chitin fragments, strongly suggesting that LysM2 domains of Ecp6 molecules do not cooperate with one another in homodimer formation. Nonetheless, we cannot exclude dimerization between Ecp6 LysM2 and a LysM module of another protein such as a plant cell surface receptor. In CBP21, a family 33 carbohydrate-binding module (CBM33, now renamed to AA10 as explained below) displaying a so-called budded β-sandwich fibronectin type-III fold (Fig. 4, panel D), six surface-exposed polar side chains (Tyr54, Glu55, Glu60, His114, Asp182 and Asn185) are involved in chitin binding (PDB ID 2BEM; Vaaje-Kolstad et al., 2005). CBP21 actually is an oxidative enzyme that introduces chain breaks in insoluble crystalline β-chitin leading to increased substrate accessibility for chitinases and thus strongly promotes chitin hydrolysis (reviewed in Levasseur et al., 2013). Merely two amino acid residues of the WW domain-like ChBD of chitinase A1 (PDB ID 1ED7; Ikegami et al., 2000), a family 12 carbohydrate-binding module (CBM12), have been shown to be involved in substrate binding and the mutation of either, Gln679 or Trp687 (Fig. 4, panel E), significantly decreases the binding activity to colloidal chitin (Hara et al., 2013). Besides the CBM families 12, 14, 18, 33 (AA10) and 50 discussed above, also CBM19 is a ChBD but no structures have been reported yet. Not discussed here are CBM families 1–3, 5, 37, 54 and 55, of which one or more members have been shown to bind chitin besides binding another substrate more characteristic for the family group (see CAZY database). Overall, from a structural viewpoint, it appears as if the ChBMs can be roughly divided in two main groups, one group of folds is designated to bind to insoluble/colloidal/crystalline chitin (CBMs 12 and 33 (AA10)) and the other group is able to bind to soluble/short chitin fragments (CBMs 14, 18, 19 and 50), each displaying different affinities that fit the purposes detailed. For completeness, one example of chitin binding by enzymes is presented here to demonstrate that face-to-face packing is not only utilized by certain members of the CBMs but also enzymes such as the 7-stranded beta/alpha barrel protein chitin deacetylase from the fungal pathogen C. lindemuthianum that possesses four subsites that are involved in chitin binding, −2, −1, 0 (active site) and +1 subsite. The enzyme is a carbohydrate esterase family 4 (CE4) member (CAZy database). In subsite 0 (Fig. 4, panel F) (PDB ID code 2IWO; Blair et al., 2005), one GlcNac monomer of a modeled triacetylchitotriose is sandwiched in between Tyr145 (edge-to-face) and Tyr173 (face-to-face) (see Blair et al., 2005). In the structurally related Streptococcus pneumoniae peptidoglycan deacetylase, a tryptophan residue is present at the position equivalent to Tyr173 of the C. lindemuthianum deacetylase (Blair et al., 2006).
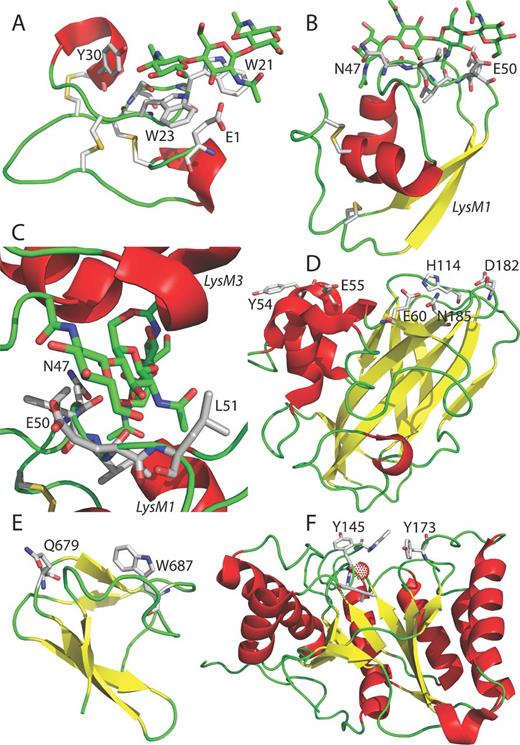
Structural view of several chitin-binding motifs. Protein structures are shown as ribbon models; alpha helices are colored in red, loops in green and beta strands in yellow. Disulfide bridges (sticks representation, sulfurs yellow) and chitooligosaccharides (sticks with carbons in green) are depicted in panels A–C. (A) Truncated hevein in complex with (GlcNAc)3 (PDB ID 1T0M; Aboitiz et al., 2004). (B) Ecp6 LysM1 of Ecp6 with (GlcNAc)4. (C) Chitin-binding groove constituted by LysM1 and LysM3 in close-up with bound (GlcNAc)4 (PDB ID 4B8V; Sánchez-Vallet et al., 2013). (D) CBP21 (PDB ID 2BEM; Vaaje-Kolstad et al., 2005). (E) chitin-binding domain of chitinase A1 (PDB ID 1ED7; Ikegami et al., 2000). (F) Chitin deacetylase (PDB ID code 2IWO; Blair et al., 2005) with a bound zinc atom shown as a red dotted sphere. Amino acid residues involved in chitin binding such as 47NLIEL51 of Ecp6 (panels B and C) are shown in sticks with carbons colored in gray. Figures were prepared with an old version (DeLano Scientific, LLC) of the PyMOL Molecular Graphics System (Schrödinger, LLC).
CONCLUDING REMARKS AND OUTSTANDING QUESTIONS
Chitin is a well-known inducer of immune responses in plants (Felix et al., 1993; Shibuya et al., 1993) and large advances in knowledge of the molecular mechanisms of chitin perception and chitin-triggered immunity in plants have been achieved since the cloning of the first plant chitin receptor from rice (Kaku et al., 2006; Miya et al., 2007; Wan et al.2008; Lee et al., 2014). However, these processes are still not completely understood, as the different molecular components that constitute receptor complexes at the plasma membrane, the dynamics of these complexes that leads to immune activation, as well as downstream signaling components, remain largely unknown. Additionally, although it is generally assumed that chitin oligomers are released during pathogen ingress and are recognized by plants, the actual length and concentration of the oligosaccharides that are released at infection sites, as well as the amount that is needed to induce an actual immune response in planta remains unknown. All this information is critical to understand the effort that is required from a pathogen to prevent the release of chitin oligomers or otherwise inhibit the induction of chitin-triggered immune responses. Furthermore, little is known about the composition and architecture of fungal cell walls in the actual interaction with plant hosts. Fungi adapt to new environments or stress conditions by modifying their cell wall structure and composition. The cell wall is not a static structure, but is continuously remodeled during host colonization. Consequently, not always the same fungal cell wall components may get in contact with host cells, and the study of the role of putative cell wall MAMPs in innate immunity remains a challenge (Latgé and Beauvais 2014). Moreover, cell wall compositions may evolve in order to provide adaptation of the pathogen to the host immune system and increase its virulence. In the animal pathogen Candida glabrata, the continuous interaction with macrophages in a serial passage approach triggered a single nucleotide mutation in chitin synthase2 (CHS2) gene that resulted in modifications in the fungal cell wall architecture. As a consequence of this changed cell wall architecture, striking alterations in the cellular morphology occurred, from spherical yeast to filamentous-like, allowing the pathogen to escape from macrophages and, consequently, increase its virulence in mice (Brunke et al., 2014).
Although plant–fungus interactions are typically studied as a one-to-one interaction, it is important to realize that these interactions take place in the presence of a wealth of other microorganisms that may impact the nature and outcome of the interaction (Rovenich et al., 2014). Plants may attract microbiome partners that help them in defense against fungal pathogens, and likely some of these microbiome partners target (chitin in the) fungal cell walls, triggering the evolution of fungal defense against such attacks. Conversely, fungi may recruit microbiome partners to invade host plants. An elegant, far-reaching, example is provided by the rice seedling blight fungus, Rhizopus microspores, that exploits the endosymbiotic bacterium Burkholderia rhizoxinica that produces the precursor of the toxin that represents the causative agent of rice seedling blight and that allows the fungus to take up nutrients from the host. So far, it has remained elusive how the bacteria that reside in the cytoplasm of the fungus are able to enter these fungal cells. Recently, it was demonstrated that B. rhizoxinica uses its type II machinery to secrete chytinolytic enzymes, encompassing chitinases and chytosanases, as well as chitin-binding proteins. Collectively, these proteins are essential for traceless bacterial intrusion and entry into the fungal cells (Moebius et al., 2014). Therefore, chitin and chitin-mediated responses of fungal organisms do not only play a direct role in innate immune responses of their hosts but also in the interaction with other organisms that may indirectly influence the outcome of colonization processes.
Intriguingly, chitin-derived molecules not only induce immune responses but they are also symbiotic signaling compounds. The mechanisms by which plants are able to discriminate between beneficial and pathogenic fungi still remain enigmatic, although a crucial step appears to be the differentiation among symbiotic signals in order to trigger the most appropriate signaling pathway to approximate friends and foes. The key to this phenomenon is in how various LysM domains are able to recognize differences in their substrates. Potentially, posttranslational modifications such as glycosylation of the LysM receptors will lead to specificity in substrate recognition (Mulder et al., 2006; Chen et al., 2014). The fact that more and more LysM domains that bind various substrates have been characterized emphasizes the need to make accurate measurements of affinity-binding values to understand how these domains discriminate between substrates. In this respect, it is furthermore relevant that during fungal colonization not just a single inducer gets in contact with the host cells, but various polysaccharides and other cell wall components may be recognized, likely leading to a different overall response than when a single inducer is detected.
BPHJT acknowledges support by the Research Council Earth and Life Sciences (ALW) of the Netherlands Organization of Scientific Research (NWO).
Conflict of interest statement. None declared.
REFERENCES