-
PDF
- Split View
-
Views
-
Cite
Cite
Pavel Dutow, Lea Wask, Miriam Bothe, Beate Fehlhaber, Robert Laudeley, Claudia Rheinheimer, Zhangsheng Yang, Guangming Zhong, Silke Glage, Andreas Klos, An optimized, fast-to-perform mouse lung infection model with the human pathogen Chlamydia trachomatis for in vivo screening of antibiotics, vaccine candidates and modified host–pathogen interactions, Pathogens and Disease, Volume 74, Issue 2, March 2016, ftv120, https://doi.org/10.1093/femspd/ftv120
- Share Icon Share
Chlamydia trachomatis causes sexually transmitted diseases with infertility, pelvic inflammatory disease and neonatal pneumonia as complications. The duration of urogenital mouse models with the strict mouse pathogen C. muridarum addressing vaginal shedding, pathological changes of the upper genital tract or infertility is rather long. Moreover, vaginal C. trachomatis application usually does not lead to the complications feared in women. A fast-to-perform mouse model is urgently needed to analyze new antibiotics, vaccine candidates, immune responses (in gene knockout animals) or mutants of C. trachomatis. To complement the valuable urogenital model with a much faster and quantifiable screening method, we established an optimized lung infection model for the human intracellular bacterium C. trachomatis serovar D (and L2) in immunocompetent C57BL/6J mice. We demonstrated its usefulness by sensitive determination of antibiotic effects characterizing advantages and limitations achievable by early or delayed short tetracycline treatment and single-dose azithromycin application. Moreover, we achieved partial acquired protection in reinfection with serovar D indicating usability for vaccine studies, and showed a different course of disease in absence of complement factor C3. Sensitive monitoring parameters were survival rate, body weight, clinical score, bacterial load, histological score, the granulocyte marker myeloperoxidase, IFN-γ, TNF-α, MCP-1 and IL-6.
INTRODUCTION
Chlamydiae are obligate intracellular bacteria. In their unique productive cycle, infectious, metabolically almost inactive elementary bodies (EBs) induce their uptake into mucosal cells. In intracellular inclusions, they differentiate to metabolically active reticulate bodies and reproduce. After redifferentiation, a new generation of EBs is released (Bastidas et al. 2013), and disseminates to neighboring cells and other organs (Laskay, van Zandbergen and Solbach 2003). The pathogen Chlamydia trachomatis is the most common sexually transmitted bacterium worldwide. The World Health Organization estimates ∼105 million new cases of C. trachomatis genital infections caused by D–K and the slightly more aggressive L1–L3 serovars (Lymphogranuloma venereum) in 2008, and the incidence rises each year in developing and industrialized countries (Starnbach and Roan 2008). Urogenital C. trachomatis infections in women can lead to vaginal mucopurulent cervicitis or endometritis. In men, C. trachomatis is a common cause of urethritis, orchitis, proctitis and epididymitis. Urogenital strains can additionally cause conjunctivitis in adults. Infections with C. trachomatis often remain asymptomatic and undetected. However, without effective treatment, severe sequels can occur: C. trachomatis can spread to joints where it remains in its persistent form. Reactive arthritis with chronic synovial inflammation lasting months or years can be the consequence. Most important, in women, difficult-to-treat pelvic inflammatory disease (PID) with chronic pain, or ectopic pregnancy and tubal infertility are severe complications of chronic C. trachomatis infection (Brunham and Rey-Ladino 2005).
The lung can also be one of the target organs of this pathogen. Infants of genitally infected pregnant women can acquire C. trachomatis in the birth canal. The published incidence rates for infants with proven exposure to C. trachomatis at birth reach up to 17% for pneumonia. These neonates show frequently long-lasting nasopharyngeal infection with only few symptoms or moderate illness (Darville 2005). In particular, in premature babies, difficult-to-treat pneumonia can also be observed. In spite of 2–3 weeks application of antibiotics (e.g. erythromycin), 20%–30% of relapses occur, and an additional course of antibiotic treatment is often required. This indicates that the available drugs are not optimally effective in the eradication of these bacteria in patients with an immature immune system (Zar 2005; Miller 2006). PCR screening of pregnant women is the best way to prevent these perinatal chlamydial infections. However, in developing countries, perinatal infections remain a relevant issue.
Anti-chlamydial therapies make use of antibiotics such as macrolides (erythromycin, azithromycin or clarithromycin), tetracyclines (e.g. doxycycline) or quinolones (e.g. levofloxacin) (Hammerschlag and Kohlhoff 2012). Treatment is effective in the majority of uncomplicated cases. However, studies in women not at risk of reinfection show in uncomplicated C. trachomatis urogenital infection ∼8% failure rates of the recommended (2010 CDC STD Treatment Guidelines) single-dose regime with azithromycin (Golden et al. 2005; Batteiger et al. 2010a; Horner 2012). Thus, there is still a need for the development of improved antibiotic regimes or drugs against C. trachomatis.
Vaccine development against this human pathogen and subsequent diseases, in particular, infertility and PID, is presently considered of high importance by many (CBRS meeting, New Orleans, Louisiana, USA, March 29–April 1, 2015). However, vaccines are still only at a preclinical stage (Hafner, Beagley and Timms 2008; Schautteet, De Clercq and Vanrompay 2011; Hafner, Wilson and Timms 2014)—one reason being that it is difficult to screen and identify ideal combinations of antigens, adjuvants and modes of application to obtain mucosal protection using the available animal models for C. trachomatis infection (see below). Similarly, the gene-knockout-based analysis used to identify critical host functions in the defense against C. trachomatis is also hampered by the below described limitations of the urogenital model.
Guinea pigs, non-human primates and mice are the most frequently used host species for experimental chlamydial infections. Mice are often preferred as laboratory animals due to their low cost, small size, fast reproduction, easy handling as well as the availability of inbred and knockout strains (De Clercq, Kalmar and Vanrompay 2013; O'Meara, Andrew and Beagley 2014). Theoretically, a urogenital mouse model for C. trachomatis would be ideal. Unfortunately, intravaginal infection of mice with common C. trachomatis laboratory strains causes only a mild, clinically asymptomatic disease with fast recovery. Only transient vaginal chlamydial discharge can be determined. Furthermore, the upper genital tract is only infected after application of high chlamydial doses directly into the uterus, uterine horns or ovarian bursa (Tuffrey et al. 1986; Carmichael et al. 2013), or after transcervical application (Nogueira et al. 2015). These procedures can lead to hydrosalpinx or infertility of mice. However, they are relatively complicated to perform and differ from the physiological route of infection.
Only the non-human pathogen C. muridarum (formerly: C. trachomatis mouse pneumonitis MoPn biovar) leads reliably to typical pathological changes of the upper genital tract after intravaginal inoculation, i.e. hydrosalpinx, fibrosis and infertility in mice, and thus, to the complications one fears upon human C. trachomatis infections (Barron et al. 1981; de la Maza et al. 1994; Darville et al. 1997; Ramsey, DeWolfe and Salyer 2000; Morrison and Caldwell 2002; De Clercq, Kalmar and Vanrompay 2013; O'Meara, Andrew and Beagley 2014). Moreover, C3H/HeN or BALB/c mice are preferred because C57BL/6 with its variety of genetically modified strains seems to be rather resistant in this model (Bernstein-Hanley et al. 2006; O'Meara, Andrew and Beagley 2014). So far, the genital (and the respiratory) C. muridarum infection model has been applied for antibiotic efficacy studies against ‘C. trachomatis’ (Kramer, Cleeland and Grunberg 1979; Beale et al. 1991; Beale and Upshon 1994; Jupelli et al. 2011; Yeruva et al. 2013). Yet, drugs that are efficient in treatment of C. muridarum infections might not always be as effective against C. trachomatis and vice versa. Additionally, vaccine development is obviously even more hampered by the use of a different chlamydial species. And, in order to identify new virulence factors of the human pathogen, the investigation of C. muridarum mutants generated by TILLING (Kari et al. 2011) or TARGETRON (Johnson and Fisher 2013) techniques can definitely not replace functional analysis of gene deletion mutants of C. trachomatis. Therefore, in addition to the urogenital model, a broadening of the spectrum of available methods addressing the functional analysis of C. trachomatis in the C57BL/6 mouse is urgently needed.
There are excellent murine lung infection models available for C. pneumoniae and C. psittaci, both species causing primary respiratory infections in humans. This type of model provides a number of well-evaluable clinical and laboratory parameters to monitor disease progress and to analyze the outcome (Rothfuchs et al. 2004; Dutow et al. 2013, 2014). Our optimized infection model is related to the work of Graham who demonstrated in 1965 that certain trachoma serovars could infect TO mice and that they are lethal upon inoculation of high amounts into the lungs (Graham 1965). In 1980, a C. trachomatis lung infection model has been described for Swiss Webster mice comparing various serovars with an LGV serovar as the most virulent (Chen and Kuo 1980; Kuo and Chen 1980). Overall, the peak of infection was then observed on day 2, followed by quick elimination of the bacteria from the lungs of surviving mice. The course of disease was relatively short (1–2 weeks) (Chen and Kuo 1980; Kuo and Chen 1980). Pneumonia could be prolonged by high doses of cortisone or cyclophosphamide, resulting in reactivation of the ocular C. trachomatis B strain (Stephens, Chen and Kuo 1982; Yang, Kuo and Chen 1983). A more detailed analysis including determination of cytokines was not performed then, and the model was not at all characterized and optimized in regard of antibiotic effects. To provide a more complete picture, studying effects on atherosclerosis, C. trachomatis serovar E was used in parallel to C. pneumoniae in lung infection of apolipoprotein E-deficient mice as a specificity control (Blessing et al. 2000; Campbell et al. 2000). Additionally, local presence of attachment-preventing glycan could lower the number of these bacteria in the freshly infected lung (Kuo et al. 2007). However, since then, this not yet optimized model—although helpful to address questions such as reactivation under immune suppression—has only sporadically been applied with one very recent exception (Nogueira et al. 2015). Thus, the need for an improved respiratory infection model in mice to screen for and to characterize improved treatment regimes, new antibiotics or vaccine candidates against the human pathogen C. trachomatis is still remaining.
The present study was performed to complement the still indispensable urogenital infection model with its species restrictions, its long duration and its limited clinical monitoring and quantitative laboratory parameters with a rapid-to-perform mouse model that is better suited for screening and first-step characterization of candidates for new antibiotics or vaccines, or of gene-knockout mice (and chlamydial mutants).
MATERIALS AND METHODS
Chlamydial culture
For mouse infection experiments, C. trachomatis serovar D/UW-3/Cx (ATCC; VR-885) and, for a subset of experiments (data not shown), C. trachomatis LGV II strain 434 (L2) (ATCC; VR-902B) were propagated in Baby Hamster Kidney 21 cells (BHK-21). For stock production, cell monolayers were infected with C. trachomatis EBs by centrifugation (55 min; 35°C; 2000 × g) in Panserin 401 medium (Cytogen, Berlin, Germany) supplemented with 1 mg mL−1 cycloheximide (Sigma-Aldrich, St Louis, MO, USA). Infected cells were cultivated at 37°C and 5% CO2 for 2 days. The cells were destroyed by glass beads mechanically, cell debris was removed by centrifugation (15 min; 500 × g), and the EBs in the supernatant were collected by centrifugation at 22 000 × g for 1 h. EBs were washed with transport medium (1× PBS including 6.86% sucrose, 0.04 mg mL−1 gentamicin, 0.002% Phenol red, 2% FCS), and collected again by centrifugation. Inclusion-forming units (IFU) were determined by titration on HeLa T cells (Sommer et al. 2009). PCR confirmed mycoplasma-free preparations.
For mock controls, BHK-21 cells were identically proceeded but without chlamydia and diluted similarly as infected cells.
Animal experiments and experimental design
All animal experiments were approved by the Local District Government (LAVES; permit numbers: 33.14-42502-04-09/1624, −12/0725 and −13/1223), and performed in accordance with the German regulations of the Society for Laboratory Animal Science (GV-SOLAS) for protection of animal life and the European Health Law of the Federation of Laboratory Animal Science Associations (FELASA).
For most ‘C. trachomatis D lung infections’, 10—12-week-old male C57BL/6J mice (Charles River Laboratories, Sulzfeld, Germany) were used. They were anesthetized using 0.1 mL 10 g−1 body weight of the following solution: Ketamine (Gräub, Albrecht, Aulendorf, Germany) 0.5 mL [100 mg mL−1]; Xylazine (Bayer, Leverkusen, Germany) 0.1 mL [2%]; NaCl (Braun, Melsungen, Germany) 4.4 mL [0.9%]. Then, 30 μL of 0.9% NaCl solution containing in most experiments 4 × 106 chlamydial IFU of the first stock preparation, or mock material were intranasally applied. For some additional experiments, it became necessary to use a second chlamydial stock preparation, as indicated in the corresponding figure legends (dose–response relationship with tetracycline, azithromycin treatment, reinfection experiment). Based on titration experiments of this preparation, 8 × 106 chlamydial IFU were intranasally applied per mouse and infection.
In the ‘first experimental setup of the antibiotic treatment’, mice were intranasally infected with C. trachomatis D (CtrD) followed by tetracycline (Tet) treatment using a high dosage of 20 mg kg−1 body weight intraperitonially (i.p.) once a day either on days 1–3 (early = E) or 3–5 (late = L) post infectionem (p.i.) (Fig. 1A). This experimental setup consisted of ‘12 differentially treated groups’ representing different regimes. In six of them, the mice were sacrificed on day 6 p.i.: CtrD/Buffer (early), CtrD/Tet (early), Mock/Buffer (late), Mock/Tet (late), CtrD/Buffer (late), CtrD/Tet (late). In order to analyze the kinetics of early Tet treatment in more detail, there were six additional groups consisting of CtrD/Buffer (early) and CtrD/Tet (early) mice that were sacrificed on days 4, 8 or 20 p.i. Of note, no differences were to be expected between a Mock/Buffer (late) and a potential Mock/Buffer (early) or Mock/Tet (late) and a potential Mock/Tet (early) group, respectively. Therefore, only both ‘late’ groups were used as general negative controls. For easier understanding, these groups were designated only as ‘Mock/Buffer’ or ‘Mock/Tet’ in the text. Tetracycline hydrochloride (Sigma-Aldrich) was applied i.p. (in a volume of 0.1 mL 10 g−1 body weight) at the indicated dosages once a day; control groups received NaCl 0.9% (‘buffer’) i.p..
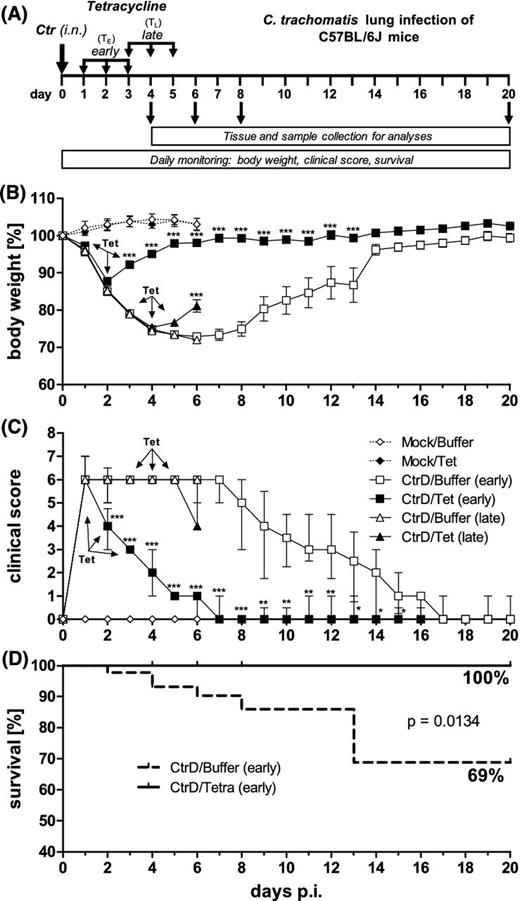
Experimental design, body weight, clinical score and survival rate of C57BL/6J mice after lung infection with C. trachomatis serovar D. (A) Mice were intranasally (i.n.) infected with 4 × 106 IFU of C. trachomatis (Ctr) or mock material and subsequently monitored for up to 20 days. The early tetracycline (Tet) treatment (TE) was applied once a day on days 1–3 after infection. The late tetracycline treatment (TL) was applied on days 3–5 after infection. In this experiment 20 mg kg−1 tetracycline i.p. were used. Control groups received buffer solution instead (NaCl). Infected early-treated mice and corresponding control animals were euthanized on days 4, 6, 8 and 20 p.i. Infected late-treated and corresponding infected control mice as well as the mock-treated mice were euthanized on day 6 p.i. (B) Body weight loss of the different groups is depicted as means ± standard errors of the means. (C) Clinical score, composed of parameters such as pilo-erection, body posture, locomotion, breathing, dehydration, attention/curiosity and secretion from nose and eyes. Score values are depicted as medians ± interquartile ranges. (D) Comparison of the survival rate of the CtrD/Buffer (early) and CtrD/Tet (early) groups. Asterisks indicate statistical significances: *P = 0.05; **P = 0.01; ***P = 0.001.
The ‘second experimental setup of antibiotic treatment’ was intended to demonstrate a dose–response relationship, but only with tetracyclinei.p. for the early treatment (days 1–3). Here, all mice were sacrificed on day 6 p.i. This second setup consisted of ‘five differentially treated groups’: CtrD-infected mice treated once a day with 20 (CtrD/TetE High), 5 (CtrD/TetE Medium) or 1.25 (CtrD/TetE Low) mg kg−1 body weight of tetracycline i.p. or buffer (CtrD/Buffer). The mock-infected control mice in the fifth group received 20 mg kg−1 body weight of this antibiotic (Mock//TetE High).
In the third experimental setup of antibiotic treatment azithromycin (Azi; Hexal AG, Holzkirchen, Germany; frozen aliquots of 200 mg 5 ml−1 suspension as stock solution) at a dosage of 80 mg kg−1 body weight (CtrD/Azi), or buffer (CtrD/buffer) was applied orally (gavage; in a volume of 0.1 mL 10 g−1 body weight) on 1 day p.i. This experiment consisted of ‘three groups’. 1% sucrose served here as ‘buffer’. Mock-infected mice of the third group received azithromycin (Mock/Azi). Again, the mice were sacrificed on day 6 p.i.
In all experiments, mice were monitored daily and the body weight was determined. Clinical parameters (pilo-erection, body posture, locomotion, breathing, dehydration, attention/curiosity and secretion from nose and eyes) were recorded until the end of the experiment (until day 4, 6, 8 or 20, respectively). Dysfunction in each parameter was rated gradually as 0, 1 or 2 points (rising depending on the severity of their appearance). The overall condition of mice was determined by adding all points, resulting in a clinical score of untroubled (0 points) to moribund (>10 points; in this case, mice were euthanized). In contrast to Streptococcus pneumoniae (or other extracellular bacteria), intracellular chlamydiae lead to a severe but delayed and protracted course of disease. Therefore, (taken into account in our permit) chlamydia-infected mice can adapt, tolerate weight loss much better, and usually recover even if they lose >20% of their body weight.
For the ‘reinfection experiment’, 21 days after primary ‘infection’ with C. trachomatis D or mock material, respectively, all mice were infected (again) with the identical amount of this strain. ‘Both experimental groups’ were euthanized on day 7 after the second infection to collect tissues and samples for further analyses.
Some C. trachomatis D lung infection experiments were also performed in complement factor C3−/− mice (B6.129S4-C3tm1Crr/J) applying 2 × 106 chlamydial IFU of the D serovar. Here, 10—12-week-old ‘female’ mice were used comparing them to their C57BL/6J wild-type counter parts.
Determination of the bacterial load in mouse lungs
Homogenates from right lung lobes were obtained as described elsewhere (Bode et al. 2012). For titration of bacterial burden, thawed, diluted tissue homogenates were centrifuged onto monolayers of HeLa T cells growing on cover slides without cycloheximide. After incubation for 24 h at 37°C, the slides were washed and fixed with ice-cold methanol before being stained with chlamydia-specific antibody (Pathfinder Chlamydia Culture Confirmation System; Bio-Rad, Redmond, WA, USA). IFU mL−1 were determined by immunofluorescence microscopy (Zeiss, Jena, Germany).
Lung histology
Left lung lobes were fixed in formaldehyde solution (4%). Sectioning and Hematoxylin and Eosin (Merck, Darmstadt, Germany) staining was performed as described elsewhere (Bode et al. 2012) and analyzed by light microscopy (Zeiss Axioskop 40). For determination of pneumonia typical pathology, the degree of inflammation was scored according to the following parameters: inflammatory cells, edema, hemorrhaging, peribronchiolar infiltrates, affected area, luminal exudates and overall disease pathology. The first three parameters were rated with a value between 0 and 2 points; the other parameters were rated with a value between 0 and 5 points. The score was determined by adding all points, resulting in a score of 0 (not affected) to 26 (highly affected) points. All preparations were evaluated and graded in a blinded manner by a veterinary expert.
Determination of myeloperoxidase and of cytokine levels in lung homogenates
For the quantification of the granulocyte marker enzyme myeloperoxidase (MPO) in lung homogenates, the ‘Mouse MPO Enzyme-linked Immunosorbent Assay Kit’ (HyCult Biotechnology, Unden, the Netherlands) was used according to the manufacturer's instructions. Absorbance at 450 nm was measured on a Titertek Multiskan MCC/340 plate reader (Labsystems, Vantaa, Finland).
For the experiments depicted in Fig. 4A–D (with one high dosage of tetracycline) and Fig. 7 (reinfection study) determination of the cytokine concentrations (IFN-γ, TNF-α, MCP-1, IL-6, IL-10, IL-12p70) in lung homogenates was performed according to the manufacturer's instructions using the ‘Mouse Inflammation Cytometric Bead Array’ (Becton Dickinson, Heidelberg, Germany). Samples were analyzed by flow cytometry (FACSCalibur, Becton Dickinson) and data analysis was performed using the FCAP array software (Becton Dickinson). The data depicted in Fig. 6 (azithromycin treatment) and Fig. S1 (Supporting Information; treatment with three different dosages of tetracycline) were determined by Mouse INF-γ and TNF-α ELISA Kits (Biolegend, San Diego, CA, USA).
IgG ELISA
Whole blood was collected by heart puncture, mixed with 100 μl of 200 mM EDTA pH 8.3 (Sigma-Aldrich). After a centrifugation step (10 000 × g, 10 min, 4°C), the supernatant was taken and frozen at –80°C. Determination of chlamydia-specific IgG levels was performed as described elsewhere (Bode et al. 2012). Briefly, heat-inactivated and ultrasound-treated C. trachomatis homogenate (0.25 μg mL−1) or mock material was used as coating antigen and 10% fetal calf serum in phosphate buffered saline as blocking reagent. Depending on experimental group, 1:50- to 1:2500-diluted plasma was added. A F(ab')2 goat anti-mouse IgG horseradish peroxidase-linked antibody (115-036-062; Dianova, Hamburg, Germany) was used to detect the IgG bound to chlamydial antigen. Standard curves defining arbitrary units were obtained using pooled plasma from C. trachomatis-infected mice.
Statistics and group sizes
Usually, the Mock/Buffer (late) group and the CtrD/Buffer (late) group of the mice were compared first using an unpaired t-test. As exception, in data of IgG levels, the Mock/Buffer (late) group was compared with the CtrD/Buffer (early) group that was sacrificed on day 20 p.i. because no IgG response can be expected during the first week of infection. In all performed analyses, significant differences were found in this first comparison indicating an effect due to chlamydial infection.
Next, ‘only groups of infected mice were statistically compared’. Most data achieved Gaussian distribution. The exceptions were data for cytokines and bacterial load. They were depicted as log-transformed values and analyzed as indicated below.
If only two groups were compared within 1 day, unpaired t-test (MPO, IgG) or Mann–Whitney test (clinical score, histological score, cytokines and bacterial load with the exception of day 6 in the first experimental setup) were used. This was the case for the data sets obtained on days 4, 8 and 20 in the first and on day 6 in the third experimental setup (i.e. with one dosage of tetracycline or azithromycin, respectively), and for day 7 after ‘reinfection’.
For the comparison of the four CtrD-infected groups sacrificed on day 6 ‘within the first experimental setup’ [CtrD/Buffer (early), CtrD/Tet (early), CtrD/Buffer (late), CtrD/Tet (late)], as well as ‘within the second setup’ [(CtrD/TetE High), (CtrD/TetE Medium), (CtrD/TetE Low), (CtrD/Buffer)] a 1-way ANOVA followed by Tukey multiple comparison test (MPO, IgG) or the Kruskal–Wallis test followed by Dunn's multiple comparison test (histological score, cytokines and bacterial load in first experimental setup for day 6) was applied.
The survival of mice was statistically analyzed with the Log-rank (Mantel–Cox) test and the body weight with a two-way ANOVA followed by a Bonferroni posttest.
In the reinfection experiment, the comparison of the body weight after the first seven days of primary with the first seven days of (re)infection was performed for four groups: CtrD/CtrD and Mock/CtrD, days 0–7 and days 21–28, each. Here, the clinical score comparing CtrD/CtrD days 0–7 and the corresponding days 21–28, as well as Mock/CtrD days 21–28, was analyzed by one-way ANOVA followed by Kruskal–Wallis test combined with Dunn's multiple comparison test.
Medians ± interquartile ranges are depicted for the non-parametric data of the clinical as well as histological scores. Body weight is represented by the means ± standard errors of the means. For all other data sets, the means ± standard deviations are shown.
The following ‘group sizes’ were used in the ‘first antibiotic treatment’ study with tetracycline in a single dosage (i.e. 20 mg kg−1 body weight): for body weight, clinical score and survival: Mock/Buffer (late) and Mock/Tet (late) n = 6; CtrD/Buffer (late) and CtrD/Tet (late) n = 9; CtrD/Buffer (early) n = 44 in the beginning and n = 8 in the end, because some mice were euthanized on days 4, 6, 8; CtrD/Tet (early) n = 36 in the beginning and n = 9 in the end, because some mice were euthanized again on days 4, 6, 8. For other experiments: Mock/Buffer (late) n = 6; Mock/Tet (late) n = 5–6; CtrD/Buffer (late) n = 9; CtrD/Tet (late) n = 8; CtrD/Buffer (early) n = 8–11, depending on the experimental group; CtrD/Tet (early) n = 9 for each experimental group. In the ‘second treatment setup’ applying three different dosages of tetracycline and using an observation period of 6 days, the group sizes of all four CtrD-infected and statistically compared groups were n = 10. The Mock/TetE High group consisted of n = 6 animals. In the ‘third setup’ applying azithromycin and observing 6 days, the group size of the Ctr/Azi and Ctr/buffer groups were n = 6. The Mock/Azi group contained n = 5 mice.
The reinfection experiment was started with n = 9 mice of the CtrD/CtrD group and n = 8 animals of the Mock/CtrD control. On day 28, bacterial load and cytokine levels were compared for n = 7 and n = 8 survivors, respectively.
GraphPad Prism V5 (Graph-Pad Software Inc. San Diego, CA, USA) was used for creating graphs and for statistical evaluation.
RESULTS
This study aimed to characterize in detail the course of lung infection with a urogenital laboratory strain of C. trachomatis in an optimized mouse model. The intention was to demonstrate the suitability of this relatively fast-to-perform and highly quantitative analysis not only for the identification of new antibiotic drugs in efficacy studies, but also for screening of vaccine candidates (as suggested by a different outcome in reinfection), and for the rapid phenotypical and functional in vivo characterization of ‘modified’ host–pathogen interactions.
Figure 1A illustrates the first experimental design: Mice were intranasally infected with a dose of 4 × 106 IFU of chlamydia followed by 20 days of observation. On days 4, 6, 8 and 20, different groups of animals were euthanized in order to obtain material to characterize the infection kinetics. To identify conditions that are optimally suited to demonstrate antibiotic effects, groups of mice were treated once a day with 20 mg kg−1 body weight of tetracycline (or buffer as control in the ‘untreated’ groups) either from days 1–3 (early) or from days 3–5 (late) p.i.
Clinical parameters and survival of infected mice with and without tetracycline treatment
Upon infection, untreated CtrD/Buffer (early or late) mice lost continuously weight until day 6 p.i. (>25%), before the remaining animals regained weight and recovered almost completely until day 20 (Fig. 1B). Early-treated CtrD/Tet animals started to regain weight on day 3 p.i.—i.e. already 2 days after the first tetracycline application, and kept a higher body weight than untreated mice until day 13. Late-treated CtrD/Tet mice started to regain weight on day 5 p.i.—i.e. also 2 days after the first antibiotic application, and their body weight was ∼10% higher than that of the non-treated mice on day 6.
Already 1 day p.i., C. trachomatis lung infection resulted in a clinical score of six points indicating severe pneumonia characterized by dyspnea, unnatural body posture, pilo-erection and slight dehydration (Fig. 1C). The score of the untreated CtrD/Buffer mice remained at that high level until day 7. From that time point on, clinical symptoms decreased continuously and disappeared completely on day 17 in this group. Early-treated CtrD/Tet animals showed already on the second day of treatment a reduction of their elevated clinical score down to four points, and their clinical symptoms disappeared completely until day 7. Consistent with the course of the body weight, CtrD/Tet (late) mice had only a slightly decreased score on day 6 p.i. compared to untreated mice—exhibiting a tendency to recover from infection. Mock-infected animals did not show any weight loss or increase in clinical score (Fig. 1B and C). The survival rate (essentially defined by reaching a critical value in the clinical score) of infected untreated CtrD/Buffer animals was only 69%, whereas none of the early tetracycline-treated CtrD/Tet mice died from chlamydial infection (Fig. 1D).
Bacterial load in lung with and without tetracycline treatment
Infectious chlamydia could be recovered from the lungs of all infected groups (Fig. 2). In CtrD/Buffer mice, the highest bacterial load was detected on day 4 p.i. and the number of bacteria decreased continuously throughout the whole experiment. Finally, on day 20 p.i., there were only two out of nine mice chlamydia-positive; the bacterial burden of the other mice from this group was below the limit of detection. Of all analyzed time points, the effect of early antibiotic treatment was most prominent on day 4 p.i., when C. trachomatis could only be found in the lung of one single animal. However, on day 6, all analyzed early-treated mice turned out to be slightly chlamydia-positive again. Yet, the bacterial load remained significantly smaller than that in the lungs of corresponding untreated mice. From day 6 on, the number of bacteria in the early-treated group decreased again, and on day 20 p.i., only two mice out of eight were still tested positive, similar as in the non-treated group.
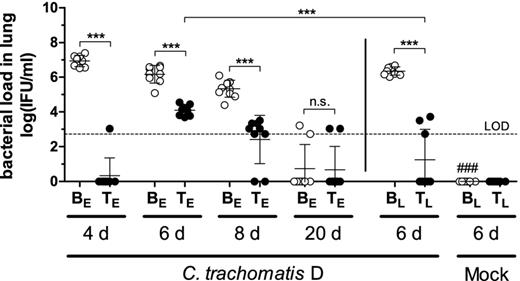
IFU recovered from lung homogenates in HeLa T cell culture determined by immune-fluorescence microscopy. The limit of detection (LOD) is indicated by the dashed line. Per definition, it is based on one single chlamydial inclusion in the HeLa cell monolayer in 40 fields of vision. In the mock-infected control, no chlamydia could be detected (data not shown). Abbreviations: BE: Buffer (early treatment); BL: Buffer (late treatment); TE: Tetracycline (early treatment): TL: Tetracycline (late treatment); groups were analyzed at different time points: 4, 6, 8 or 20 days p.i. A dosage of 20 mg kg−1 body weight of tetracycline was applied i.p.. Means ± standard deviations are depicted. Asterisks and hashtags indicate statistical significances: ***P = 0.001; n.s. = not significant; ###P = 0.001 (difference Mock/Buffer (late) versus CtrD/Buffer (late)).
Late treatment of CtrD-infected mice also led to a decrease of infectious bacteria on day 6 p.i. At that time point, only three out of eight mice of the late-treated group were tested positive, whereas all early-treated mice had chlamydia in the lung.
In summary, the (in untreated mice continuously decreasing) bacterial loads on days 4, 6 and, 8 p.i. were significantly reduced by tetracycline treatment. No chlamydiae were detected in mock-infected control mice.
Lung histopathology and MPO levels in the lung with and without tetracycline treatment
Mouse lung tissue obtained from all 12 different groups of animals was H&E-stained. There was no histological sign of infection and inflammation in mock-infected mice (Fig. 3A), independent of whether they had been treated with buffer (Mock/Buffer—depicted) or tetracycline (Mock/Tet—not depicted). In contrast, the histology of untreated chlamydia-infected mice (CtrD/Buffer) showed severe inflammatory reactions in the lung tissue at days 4, 6 and 8 after infection, including severe edema of the stroma, obstruction of bronchioli with plugs of mucus and neutrophils, and frequently, areas with hemorrhage indicating the destructive aspect of the infection. Peribronchial cuffs and alveolar infiltrates were dominated by neutrophils, and more than 75% of investigated lung tissue was affected. At day 20 p.i., this group showed a marked decrease of the inflammatory process, indicating the natural healing process of the disease.
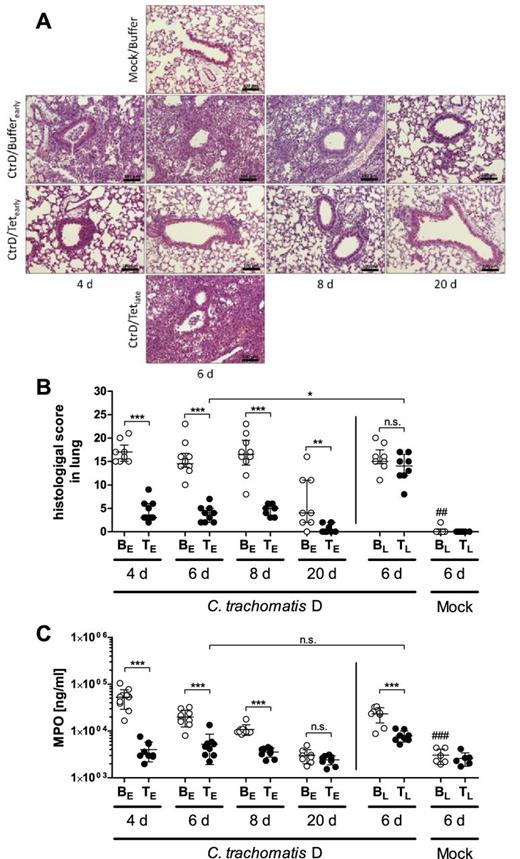
Histopathology of the lungs and granulocyte marker enzyme MPO in lung homogenates. (A) Representative images of lung sections from different experimental groups of C57BL/6J mice at the dedicated time points. Above: Mock/Buffer group at day 6 p.i.: unaltered morphology of alveolar tissue and bronchiole. Second line: CtrD/Buffer (early) group at days 4, 6, 8 p.i.: bronchiolitis with luminal neutrophils, peribronchial and stromal infiltration with neutrophils, alveoli containing edema and inflammatory cells; day 20 p.i.: mild edema, only very few stromal inflammatory cells left. Third line: CtrD/Tet (early) group at days 4, 6, 8 p.i.: mild inflammatory reaction without signs of bronchiolitis; day 20 p.i.: almost recovered morphology of alveolar tissue. Bottom line: CtrD/Tet (late) group at day 6 p.i.: findings similar to the CtrD/Buffer (early) group; H&E staining; bar = 100 μm. (B) Histopathological score of lung inflammation composed of different parameters such as inflammatory cells, edema, hemorrhaging, peribronchiolar infiltrates, affected area and overall disease pathology (for details see M&M section). Medians ± interquartile ranges are depicted. (C) Concentration of granulocyte marker enzyme MPO determined by ELISA. Means ± standard deviations are depicted. Abbreviations: BE: Buffer (early treatment); BL: Buffer (late treatment); TE: Tetracycline (early treatment): TL: Tetracycline (late treatment); groups were analyzed at different time points: 4, 6, 8, or 20 days p.i. A dosage of 20 mg kg−1 body weight of tetracycline was applied i.p.. Asterisks and hashtags indicate statistical significances: *P = 0.05; **P = 0.01; ***P = 0.001; n.s. = not significant; ##P = 0.01 and ###P = 0.001 (difference Mock/Buffer (late) versus CtrD/Buffer (late).
Animals with an early onset of treatment (CtrD/Tet (early)) showed a milder progression of disease, with only a few locations of peribronchial and alveolar infiltration at the investigated time points. No hemorrhage and only in some cases, mild edema could be detected.
In contrast to this group, the late onset of treatment (CtrD/Tet (late)) resulted in a severe inflammation of the lung tissue, comparable to the untreated group at day 6 (CtrD/Buffer).
For quantification and statistical analysis, histological scoring addressing signs of inflammation was additionally performed (Fig. 3B). Lung histology of the infected but untreated groups (CtrD/Buffer (early)) was scored with ∼14–17 points for the medians on days 4, 6 and 8 p.i. On day 20, the median of the histological score was diminished to ∼4 points, which was consistent with the simultaneous and spontaneous clinical recovery of the mice. In early-treated mice, the histological score was significantly lower than that of the corresponding untreated groups at each respective time point. On day 20 p.i., lung histology was almost back to normal level in the early-treated infected animals.
The scores of both late-treated groups (CtrD/Buffer and CtrD/Tet) were nearly identical (∼15 points) on day 6, indicating that the late tetracycline treatment had no effect on the—until the start of treatment—most likely already advanced lung histopathology. Consequently, the late tetracycline-treated group exhibited a much higher inflammatory score on day 6 than the early tetracycline-treated mice at the same time point.
In summary, lung histopathology was much lower in all early-treated groups than in the corresponding untreated groups. However, when treatment was applied later in infection, i.e. from days 3 to 5, no rapid general positive effect on the ongoing inflammation became detectable. Mock-infected animals did not show an elevated histopathological score.
In order to assess granulocytes in the mouse lungs in more detail, the levels of their marker enzyme MPO were determined (Fig. 3C). On days 4, 6 and 8 p.i., MPO levels were elevated in the chlamydia-infected untreated groups and became normal on day 20—similar to the levels of mock-infected mice. When early treated, the enzyme levels in the lungs remained low on all four analyzed time points, similar as observed in mock-infected mice. Analyzed on day 6 p.i., late treatment of infected mice had a decreasing effect on the MPO levels; and focusing on day 6, no difference was found between the early- and the late-treated chlamydia-infected groups.
Cytokine levels in the lung with and without tetracycline treatment
In order to determine the concentration of several cytokines that are known to be elevated and important in chlamydial lung infection, the Mouse Inflammation Cytometric Bead Array for IFN-γ, TNF-α, MCP-1 (Monocyte Chemoattractant Protein-1), IL-6, IL-10 and IL-12p70 was performed. The levels of the two latter cytokines remained below the limit of detection (data not shown).
Overall, levels of IFN-γ, TNF-α, MCP-1 and IL-6 were drastically elevated on days 4, 6 and 8 upon C. trachomatis infection. (Fig. 4A–D). Consistent with the good clinical appearance of mice on day 20, inflammatory cytokines could then no longer be detected in the lungs. Both, early and late treatment clearly led to a reduction of elevated levels of the four cytokines. Only for IFN-γ, the tetracycline-dependent decrease did not reach significance comparing the CtrD/Buffer (late) and the CtrD/Tet (late) groups—though, even in this case, there was a clear tendency (Fig. 4A).
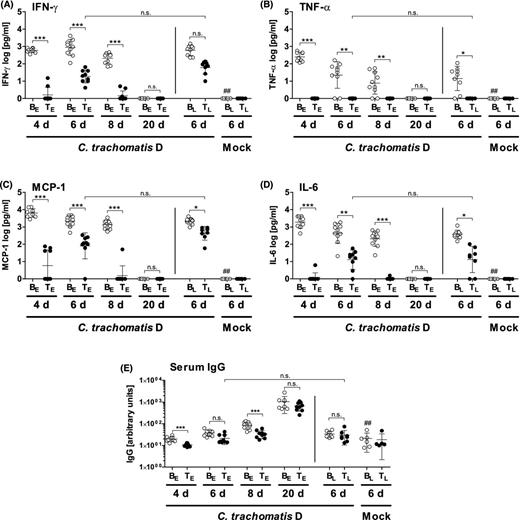
Concentrations of the cytokines IFN-γ (A), TNF-α (B), MCP-1 (C) and IL-6 (D) in lung homogenates and C. trachomatis-specific IgG titer in serum (E). A dosage of 20 mg kg−1 body weight of tetracycline was applied i.p.. The cytokines were determined by the Mouse Inflammation Cytometric Bead Array. Levels of IL-10 and IL-12 p70 were below the LOD (data not shown). Means ± standard deviations are depicted. Abbreviations: BE: Buffer (early treatment); BL: Buffer (late treatment); TE: Tetracycline (early treatment): TL: Tetracycline (late treatment); groups were analyzed at different time points: 4, 6, 8 or 20 days p.i. Asterisks and hashtags indicate statistical significances: (A–D) *P = 0.05; **P = 0.01; ***P = 0.001; n.s. = not significant; ##P = 0.01 (difference Mock/Buffer (late) versus CtrD/Buffer (late). (E) In order to show an effect due to chlamydial infection, the Mock/Buffer (late) group was compared with the CtrD/Buffer (early) group from day 20 and not, as in other analyses, with the CtrD/Buffer (late) group. The latter group was already sacrificed on day 6 p.i. and at this time point, almost no IgG response can be expected, yet.
In the early treated mice, there was a slight increase of IFN-γ, MCP-1 and IL-6 levels from days 4 to 6 p.i., in parallel to the observed transient increase of the bacterial load (see also Fig. 2). Of note, the TNF-α response was not only reduced but completely absent in all tetracycline-treated infected mice (Fig. 4B).
Chlamydia-specific IgG levels in serum with and without tetracycline treatment
In order to get insight into the humoral response against C. trachomatis with and without antibiotic treatment, the chlamydia-specific IgG levels were determined in serum (Fig. 4E). As expected, during the first eight days of infection, only a slight increase of the IgG levels could be observed upon infection in both, untreated and treated animals. In mice receiving the antibiotic, the increase was slightly delayed. However, 20 days p.i., the achieved anti-chlamydial IgG concentration was finally high in all infected groups, independent of the tetracycline treatment.
Dose–response dependency of the early treatment with tetracycline i.p.
The first experimental setup of the antibiotic treatment was modified to demonstrate dose dependency of the course of the disease. Thus, tetracycline was not only applied (i.p.) on days 1–3 p.i. at 20 mg kg−1 body weight (high) and 0 mg kg−1 (i.e. buffer), but also at a ‘medium’ and ‘low’ concentration of 5 and 1.25 mg kg−1 body weight, respectively (Fig. 5 and Fig. S1, Supporting Information). Significant differences between all four groups of C. trachomatis D-infected mice became apparent in the body weight on day 4. On days 3 and 5, and partially also on day 6 p.i., there were also significant differences between the body weights of several CtrD-infected groups (Fig. 5A, Table S1, Supporting Information). For the clinical score (Fig. 5B), the bacterial load, the concentrations of MPO and the key cytokines IFN-γ and TNF-α, a trend for a dose-dependency was obvious. However, these differences reached only significance comparing the buffer-treated group with the three tetracycline-treated groups (Fig. S1, Supporting Information).
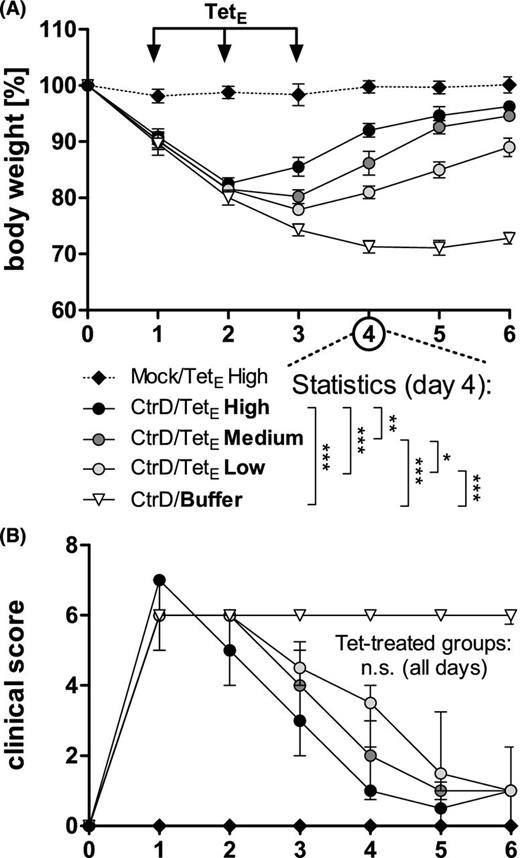
Dose–response in early treatment with tetracycline i.p. of body weight and clinical score of C57BL/6J mice after lung infection with C. trachomatis serovar D and early treatment with different dosages of tetracycline i.p. (TetE). Mice were intranasally (i.n.) infected with 8 × 106 IFU (of a new preparation) of C. trachomatis (CtrD) or mock material (Mock) and subsequently monitored until they were euthanized on day 6 p.i. Tetracycline was applied once a day on days 1–3. In this experiment 20 (TetE High), 5 (TetE Medium), and 1.25 (TetE Low) mg kg−1 body weight tetracycline or buffer (NaCl) were used. (A) Body weight is depicted as means ± standard errors of the means. (B) Clinical score values are depicted as medians ± interquartile ranges. Asterisks indicate (for day 4 p.i. as example) significant differences between the infected groups: *P = 0.05; **P = 0.01; ***P = 0.001; n.s. = not significant. On days 3 and 5, and partially also on day 6 p.i., depending on the varying dosages of tetracycline there were also significant differences between the body weights of several CtrD-infected groups (see also Table S1, Supporting Information).
Azithromycin treatment of C. trachomatis D lung infection
In addition to the 3 days of i.p. treatment with tetracycline, in a further experiment, azithromycin was applied orally (gavage) as a single dose on day 1 p.i. Compared to buffer-treated C. trachomatis D-infected mice, the body weight of the azithromycin-treated animals became significantly higher on day 3 p.i. almost reaching on day 6 p.i. the weight of non-infected control mice (Fig. 6A). The clinical score improved even earlier (Fig. 6B). Azithromycin reduced the bacterial load by ∼3 log 10 levels on day 6 p.i. (Fig. 6C). Corresponding to that, the elevated granulocyte marker MPO was then almost back to normal (Fig. 6D), and the levels of the key cytokines IFN-γ and TNF-α (determined by ELISA) were significantly diminished (Fig. 6E and F).
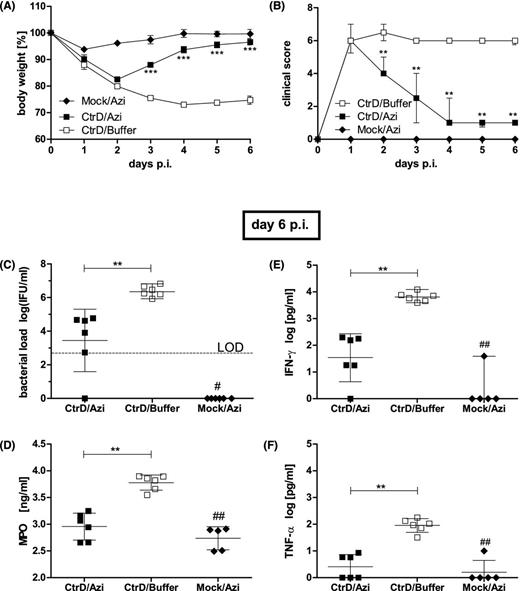
Oral treatment with a single dose of azithromycin. C57BL/6J mice were intranasally (i.n.) infected with 8 × 106 IFU of C. trachomatis D or mock material (Mock) and subsequently monitored until they were euthanized on day 6 p.i. Azithromycin (Azi; 80 mg kg−1 body weight) or buffer (1% sucrose solution) were applied as a single dose on day 1 p.i. (A) Body weight of the different groups is depicted as means ± standard errors of the means. (B) Clinical score values are depicted as medians ± interquartile ranges. (C) The number of IFU recovered from lung homogenates on day 6 p.i. is depicted as means ± standard deviation. The LOD is indicated by the dashed line. (D) Concentration of the granulocyte marker enzyme MPO determined by ELISA. Means ± standard deviations are depicted. (F) Concentrations of the key cytokines IFN-γ, and (E) TNF-α in lung homogenates determined by ELISA (means ± standard deviation). Asterisks and hashtags indicate statistical significances: */#P = 0.05; **/##P = 0.01; ***/###P = 0.001.
Reinfection experiment (without antibiotics)
We wanted to see how far repetitive C. trachomatis lung infection with its assumed effects on the specific immune response modifies the course and outcome of secondary infection. Therefore, intranasal application with the identical strain (of a new preparation) of C. trachomatis D was repeated in mice that had just recovered from primary infection on day 21. Mice were observed for seven additional days before they were sacrificed for analysis (Fig. 7). Mice that were reinfected, (CtrD/CtrD) showed higher body weight compared to mice that were infected for the first time on day 21 (Mock/CtrD) (Fig. 7A). Significant differences in weight were observed from days 25 to 28. Moreover, the relative loss of body weight comparing days 4–7 after primary infection with the same days after reinfection (i.e. days 25–28) within the CtrD/CtrD group was less dramatic after the reinfection. Similarly, reinfected mice (CtrD/CtrD) showed on days 22–23 and 25–28 less clinical symptoms compared to mice infected for the first time on day 21 (Mock/CtrD): (Fig. 7B). The clinical score was also diminished after reinfection (i.e. days 2–7 versus days 23–28) within the CtrD/CtrD group. On day 28 of this experiment, the bacterial load in the lungs of reinfected mice was diminished by ∼3 log 10 levels compared to mice that were infected for the first time on day 21 (Fig. 7C). In accordance to that, the levels of IFN-γ, TNF-α, MCP-1 and IL-6 on day 28 were smaller in the CtrD/CtrD compared to the Mock/CtrD group indicating a diminished increase after reinfection (Fig. 7D). The significant smaller histopathological score indicated reduced inflammation during reinfection (Fig. 5E). These data confirmed acquired partial protection against C. trachomatis.
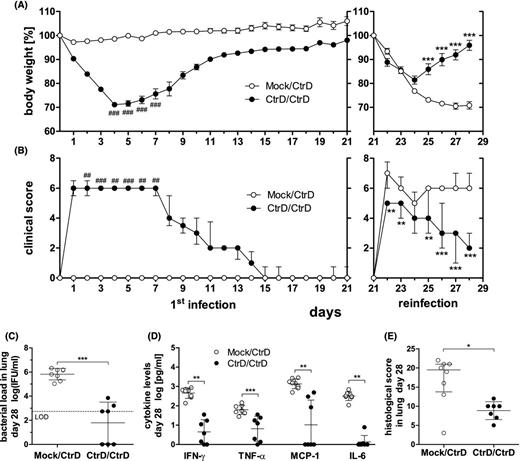
Lung reinfection of C57BL/6J mice with C. trachomatis serovar D. Mice were infected with 8 × 106 IFU of a new preparation of chlamydia (CtrD) or mock material (Mock)—left panels in (A) and (B). On day 21, all mice were (re)infected with the identical amount of chlamydia and monitored for additional 7 days until day 28—right panels in (A) and (B). (A) Body weight of the two groups (CtrD/CtrD and Mock/CtrD) was calculated for each animal as percentage in relation to its weight on the day of first infection (day 0), or reinfection (day 21), respectively. The weight is depicted as means ± standard errors of the means. (B) Clinical score values are depicted as medians ± interquartile ranges. Asterisks indicate statistical significances comparing the CtrD/CtrD with the Mock/CtrD group from day 21 to 28: *P = 0.05; **P = 0.01; ***P = 0.001. Hashtags indicate statistical significances comparing the first seven days after primary infection with the first seven days after reinfection within the CtrD/CtrD group, only: #P = 0.05; ##P = 0.01; ###P = 0.001. (C) Chlamydial load in the lung of the CtrD/CtrD and Mock/CtrD mice on day 28. The LOD is indicated by the dashed line. In the mock-infected control, no chlamydia could be detected (data not shown). (D) The cytokines IFN-γ, TNF-α, MCP-1 and IL-6 in lung homogenates on day 28 determined by Mouse Inflammation Cytometric Bead Array. (E) Histopathological score of lung inflammation. Medians ± interquartile ranges are depicted. Asterisks indicate statistical significances: **P = 0.01; ***P = 0.001.
C. trachomatis D lung infection with complement factor C3−/− mice (without antibiotics)
Guided by the knowledge that the complement system is critical in the development of a protective immune response against C. psittaci DC 15, we compared C. trachomatis lung infection in wild-type versus complement factor C3−/− mice. In these mice lacking all main effector functions of the complement system (as well as in C3a receptor−/− mice where one of these functions is missing) complement-dependent protection, presumably based on optimal induction of specific T cells, plays an important role during the second week of C. psittaci lung infection (Bode et al. 2012; Dutow et al. 2014). Performing similar lung infection experiments with C. trachomatis D in (female) mice, the differences in body weight of C3−/− and wild-type mice were rather discrete (Fig. 8A). However, during the observation period of 21 days, clinical score and survival differed significantly during the second and third week (Fig. 8B and C)—indicating that the complement system participates also in the defense against the here used chlamydial species.
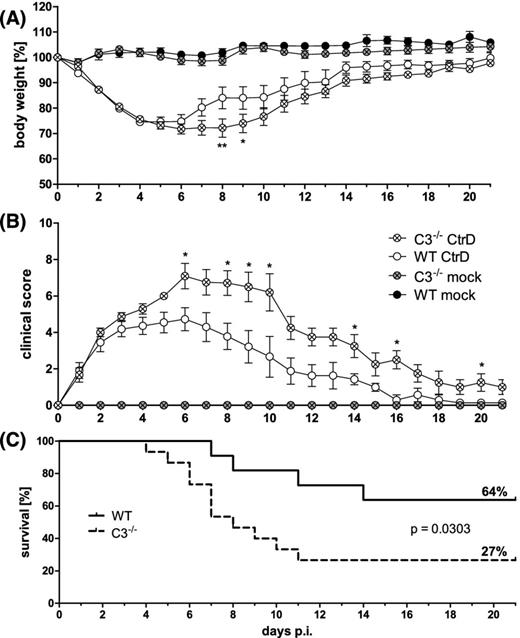
Lung infection of complement factor C3−/− and C57BL/6J wild-type mice with C. trachomatis serovar D. Female mice were infected with 2 × 106 IFU of C. trachomatis D (CtrD) or mock material (Mock), and observed for 21 days. (A) Body weight is depicted as means ± standard errors of the means. (B) Clinical score values are depicted as medians ± interquartile ranges. (C) Comparison of the survival rate of C3−/− and C57BL/6J mice. Asterisks indicate statistical significances between the infected groups: *P = 0.05; **P = 0.01.
DISCUSSION
In this study, we established an optimized lung infection model for the human pathogen C. trachomatis serovar D (and L2—data not shown) in C57BL6/J wild-type mice. In our model, the effects of chlamydial infection and antibiotic treatment (as one example for its usefulness) are relatively easy to quantify and to compare.
In an almost identical experimental setting, the infectious dose to achieve comparable severity of disease in mice was similar for C. trachomatis D and various other laboratory strains of C. pneumoniae (Sommer et al. 2009). In contrast, approximately a hundred times less bacteria of C. psittaci strain DC15 were already equally pathogenic (Bode et al. 2012; Dutow et al. 2014). Severe symptoms of pneumonia caused by C. trachomatis could already be observed on the first day after infection. Remarkably, this onset of disease was even slightly faster than that caused by the two other chlamydial species used by our group.
After one week, mice started to recover continuously until they reached normal weight and clinical score around day 17 p.i. Almost one-third died during that period (i.e. were prematurely removed from the experiment after reaching a critical value in the clinical score). Infectious chlamydia could be recovered from lung homogenate in high amounts during the first week p.i. followed by their elimination, illustrated by the fact that less than one-quarter of the animals was still tested chlamydia-positive until day 20. Consistent with the time course of the bacterial load in the lungs, the levels of inflammatory cytokines and of granulocyte marker enzyme MPO were elevated in lung homogenate. The presence of chlamydia was accompanied by histopathological changes reflected in an inflammatory score that stayed highly elevated until day 8 of infection. Although the majority of mice was chlamydia-negative and cytokines were no longer elevated 20 days p.i., most mice were then still slightly histologically affected. Thus, C. trachomatis lung infection seems to have relatively long-lasting effects on lung tissue. Additionally, as demonstrated by high chlamydia-specific IgG levels in serum, a humoral immune response developed within 20 days.
In order to characterize the influence of the timing of antibiotic treatment in immunocompetent individuals, different regimes, i.e. early and late i.p. applications of a high dosage (20 mg kg−1) of tetracycline for 3 days were compared. After treatment starting on day 1, all infected mice survived, whereas ∼1/3 of untreated mice died. This early application could drastically diminish the clinical symptoms within 2 days, and led within one week to complete clinical recovery with normal score and body weight. One day after the last early treatment, the number of infective IFU was reduced by at least 3–4 log 10 levels, with eight out of nine tetracycline-treated mice being below the level of chlamydial detection. Remarkably, the chlamydial load increased within the following 2 days again ∼10–100fold, proving 3 days of tetracycline treatment as insufficient to eliminate all chlamydia. Nevertheless, in regard of remaining infectious bacteria, there was still a significant advantage 4 days after the end of early treatment, i.e. on day 8 p.i. Intriguingly, our therapeutic mouse model was able to reflect in vivo the relapse that has recently been described for 3 days of doxycycline application in cell culture (Padberg, Janssen and Meyer 2013). Applying tetracycline from days 1 to 5 p.i., in nine of nine additionally investigated mice the chlamydial load in the lung remained below the limit of detection (data not shown), thereby confirming that longer treatment with this drug is needed. The positive effect of early tetracycline application (for 3 days) became even more visible in the continuously rather low histological score, including day 6 p.i. when the ‘relapse’ of bacteria occurred. However, this transient increase in the chlamydial load was reflected by the levels of IFN-γ, MCP-1 and pro-inflammatory IL-6. Only the TNF-α response (determined by Cytometric Bead Array) was completely abrogated in the lung homogenate of early treated mice. However, this might partially be due to assay sensitivity because—using later an ELISA with other antibodies—a slight elevation of TNF-α (compared to mock-infected control mice) was apparent in the tetracycline dose-dependency experiment and also after treatment with azithromycin. Although application of the antibiotic delayed the specific humoral response, the anti-chlamydial IgG levels of treated and untreated mice were comparably high ∼3 weeks after infection.
The early treatment with tetracycline with an observation period of 6 days was additionally performed with decreasing concentrations of this antibiotic (original and 1:4 or 1:16 diluted). Regarding the order of the medians or means, a dose dependency is obvious. However, early applied tetracycline seems to interfere rather potently in chlamydial growth resulting in rather small differences between the three tetracycline-treated groups. Nevertheless, these dose-dependent differences reached significance in the body weight on days 4 and 5 (and partially also 3 and 6) p.i.
The effect of late tetracycline treatment from days 3 to 5 p.i. was less prominent. Nevertheless, there was still an effect on body weight and (as a trend) on clinical score. In addition, the bacterial load in the lung decreased by at least ∼3 log 10 levels compared to untreated animals; only three out of eight mice of the late-treated group were still tested C. trachomatis positive 6 days p.i. There was also a discrete improvement in lung histology, reflected by a smaller number of granulocytes (i.e. their marker enzyme MPO) 1 day after the end of late treatment. Application of tetracycline had again the strongest effect on TNF-α. There was also a significant tetracycline-dependent decrease in the levels of MCP-1 and IL-6. However, for IFN-γ the difference between late treated and untreated mice did not reach significance.
Taken together, 3 days of treatment with tetracycline in a high dosage was able to drastically diminish but not to completely kill C. trachomatis in the mouse lung. Treatment starting on day 1 p.i. caused an extreme improvement of almost all parameters. Delayed treatment starting on day 4 p.i. could only shorten the course of disease. Efficiency appeared smaller, mainly because immunocompetent C57BL/6J mice in the control group were able to clear C. trachomatis from the second week p.i. on, without any help of antibiotics. Our results suggest that the here presented antibiotic treatment against C. trachomatis might mainly serve to gain time for the development of an effective specific (T cell) immune response. This might explain why newborns, the main risk group for lung infection in humans, need a relatively long treatment of up to 3 weeks, and why many relapses occur after antibiotic treatment of pneumonia and conjunctivitis with C. trachomatis in these young patients with their immature immune system. It also suggests that treatment of C. trachomatis-infected immunocompromised adults might be much more challenging. Differences in the effectiveness of the immune response against intracellular bacteria might also play a role in the 8% treatment failure rate of azithromycin in uncomplicated C. trachomatis urogenital infection (Golden et al. 2005; Batteiger et al. 2010a; Horner 2012).
The oral treatment with a single dose of azithromycin on day 1 p.i. led to similar results as the early i.p. treatment with tetracycline over 3 days confirming the practical advantage of the macrolide with its long biological half-life. Additionally, it corroborates the usability of our optimized mouse lung infection model for the characterization of C. trachomatis-directed antibiotics.
Lung infection experiments with the complement factor C3 knockout mouse resulted in significant differences in clinical score and survival compared to wild-type mice, thereby demonstrating the usability of the lung infection model for analysis of components of the immune system directed against C. trachomatis. Intriguingly, the complement-dependent differences were smaller than those observed in our former study with C. psittaci. Thus, there might be chlamydia-species specific differences in their ‘sensitivity’ to complement. An in-depth analysis is needed to clarify this issue in the future.
In immunocompromised animals, the therapeutic effect of a drug depends much stronger on its bactericidal potency and avoidance of persistence induction. Studies in the respiratory C. trachomatis infection model with antibiotic treatment of IFN-γ (or complement C3 or C3a-receptor) deficient C57BL/6 mice (Dutow et al. 2014; Nogueira et al. 2015) will help to clarify this important point.
The acquired protective immune response against related chlamydial species is dominated by an effective T-cell response with IFN-γ and TNF-α as key cytokines, only supported by chlamydia-specific antibodies (Rothfuchs et al. 2004; Hafner, Wilson and Timms 2014). Only recently and partially parallel to our study, the Starnbach laboratory investigated in detail the adaptive immunity obtained by reinfection with C. trachomatis L2 (Nogueira et al. 2015). Obviously, viable C. trachomatis cannot be regarded as an ideal vaccine. Nevertheless, reinfection with the same chlamydial strain is well suited to give—as a proof of principal—an impression about the usability and potential of the lung infection model for future vaccine development. Intriguingly, focusing in their investigation on the bacterial load, intranasal immunization with C. trachomatis L2 confers not only protection in the lung, but leads also to cross-mucosal protection against secondary challenge in the genital tract. Remarkably, specific CD4+ and CD8+ T cells are raised during lung infection and each of these T-cell subpopulations together with IFN-γ secretion is sufficient to provide protection during secondary challenge with the L2 serovar (Nogueira et al. 2015).
We can confirm this promising data for the different C. trachomatis serovar D, i.e. the decrease of the bacterial load in the lung by at least 3 log 10 levels upon secondary infection. Additionally, parameters whose increase characterizes the severity and course of lung disease, such as the levels of IFN-γ, TNF-α, MCP-1 and IL-6, were significantly reduced due to previous C. trachomatis immunization—complementing the recent study with the L2 serovar that focused on cellular immunity.
Thus, considering the results of the two reinfection studies, the mouse lung infection seems to be at least well suited as a screening procedure—much easier and faster than in urogenital infection—to determine protective effects of vaccine candidates. Intranasal application might even be the route of choice to later provide simultaneously protection against chlamydial infections at other, clinically more important sites.
However, considering clinical score and body weight during the first week of reinfection (and the histological score) the degree of achieved protection against serovar D was limited suggesting mainly a shortening of disease—although all antigens must have been present at the ideal location during primary infection with the identical chlamydial strain. This is in accordance with the only partial protection achieved in natural human genital infection (Batteiger et al. 2010b). These results indicate that the development of a regular vaccine against C. trachomatis will not be trivial, besides additional problems caused by the various serovars. In particular, it might be necessary to boost the immune response (or to overcome induced tolerance) against antigens of C. trachomatis by their optimal combination with adjuvants.
Overall, genital C. trachomatis infection in mice does only weakly resemble infection of the human genital tract (Darville et al. 1997; Ramsey, DeWolfe and Salyer 2000; De Clercq, Kalmar and Vanrompay 2013), and the observation period in urogenital infection with C. muridarum to observe pathological changes of the upper genital tract or infertility is rather long (weeks to months). Moreover, the urogenital model does not exhibit any easily and continuously detectable clinical parameters such as loss of body weight or dyspnea. Additionally, the determination of bacterial load in vaginal swabs is only a semi-quantitative method.
Most of these limitations do not apply for the optimized mouse lung infection model with C. trachomatis. The here described pulmonary model, that does not represent the most typical but at least one of the possible target sites of the human pathogen, is well suited for fast comparative analysis of a relative high number of varying conditions. After intranasal application of the bacteria, this model leads within a few days to a plethora of easily quantifiable laboratory parameters. In almost all settings, body weight, bacterial load, IFN-γ, TNF-α and MPO levels belong to the most sensitive and valuable ones. These advantages underline the usefulness of the C. trachomatis lung infection as in vivo screening method to study general features of chlamydia or the host cell interaction. Of course, this model has also its limitations—and should thus be combined with the urogenital model—in regard of questions that address specific features of the urogenital tract, such as virulence factors that are only important in this tissue, or the achievable concentration of an antibiotic at this location.
Taken together, we established a practicable, quantifiable, relatively fast-to-perform respiratory infection model for the medically relevant human pathogens C. trachomatis serovar D (and L2—data not shown) in not immunocompromised infected C57BL/6 mice. Our results indicate that this lung infection model (in combination with immunocompromised gene knockout mice) will not only be helpful to complement the urogenital mouse infection for the screening of new antibiotics and vaccine candidates. Moreover, it seems to be well suited for the elucidation of certain aspects of the immune response against the human pathogen, and, most likely, also for a functional analysis of upcoming C. trachomatis mutants.
SUPPLEMENTARY DATA
We cordially thank the head of the Institute, Prof. S. Suerbaum, for his support.
AUTHOR CONTRIBUTIONS
PD and AK conceived the experiments, analyzed the data and wrote the manuscript; LW analyzed part of the data and designed several figures; PD, LW, MB, BF, RL, CR performed experiments and SG, histology; ZY and GZ participated in the experiment with the complement deficient mouse.
FUNDING
This work was supported by the German Federal Ministry of Economic Affairs and Energy [AiF-BMWi grant KF 3309801LW4] (AK, PD, RL, LW). First preliminary experiments for the setup of the C. trachomatis mouse lung infection model were supported by the German Federal Ministry of Education and Research [BMBF Grant No. 01Kl10011F ‘Zoonotic Chlamydiae’ IX] (AK, PD, RL). Funding for MB was provided by the German Research Foundation DFG [grant of the International Research Training Group 1273].
Conflict of interest. None declared.
REFERENCES