-
PDF
- Split View
-
Views
-
Cite
Cite
Fan Zhang, Lauren Jonas, Hanzhi Lin, Russell T Hill, Microbially mediated nutrient cycles in marine sponges, FEMS Microbiology Ecology, Volume 95, Issue 11, November 2019, fiz155, https://doi.org/10.1093/femsec/fiz155
- Share Icon Share
ABSTRACT
Efficient nutrient cycles mediated by symbiotic microorganisms with their hosts are vital to support the high productivity of coral reef ecosystems. In these ecosystems, marine sponges are important habitat-forming organisms in the benthic community and harbor abundant microbial symbionts. However, few studies have reviewed the critical microbially mediated nutrient cycling processes in marine sponges. To bridge this gap, in this review article, we summarize existing knowledge and recent advances in understanding microbially mediated carbon (C), nitrogen (N), phosphorus (P) and sulfur (S) cycles in sponges, propose a conceptual model that describes potential interactions and constraints in the major nutrient cycles, and suggest that shifting redox state induced by animal behavior like sponge pumping can exert great influence on the activities of symbiotic microbial communities. Constraints include the lack of knowledge on spatial and temporal variations and host behavior; more studies are needed in these areas. Sponge microbiomes may have a significant impact on the nutrient cycles in the world’s coral reef ecosystems.
INTRODUCTION
Regarded as the ‘rainforests of the sea,’ coral reef environments cover less than 0.1% of the global ocean but are home to more than 25% of marine organisms and rank among the top ecosystems in primary production on earth (Spalding, Ravilious and Green 2001). These ecosystems are found mostly in the euphotic zone of temperate and tropical regions (Veron 1995), with very low nutrients in the surrounding water column to support one of the most productive and biodiverse ecosystems on the planet. For a long time, researchers have been puzzled by this ecological conundrum of high productivity and biodiversity in very low-nutrient waters in the coral reef environment, commonly referred to as ‘Darwin’s Paradox’ (Darwin 1842). Benthic invertebrates comprise a large proportion of the coral reef biomass and play a central role in benthic-pelagic nutrient coupling. Limited nutrients may favor close microbial symbioses with these invertebrates over evolutionary time scales. Coral is a well-studied example of these symbioses and has been comprehensively reviewed (Rosenberg et al. 2007; Rädecker et al. 2015).
In coral reef ecosystems, marine sponges are important habitat-forming organisms in the benthic community and harbor abundant microbial symbionts. The internal structure of a typical demosponge containing microbial symbionts is shown in Fig. 1. Depending on the density of microbial cells that reside in their mesohyl, sponges can be divided into two categories: high microbial abundance (HMA) sponges host a microbial density 3–4 orders of magnitude greater than the surrounding environment and low microbial abundance (LMA) sponges contain the same order of magnitude as the surrounding seawater (Hentschel et al. 2003; Taylor et al. 2007; Gloeckner et al. 2014). Because of their abundance, high numbers of associated microbes, and ability to filter large volumes of water, sponges have the potential to be key organisms in benthic-pelagic coupling and nutrient cycling in coral reef ecosystems. During the process of filter-feeding, sponges can filter a volume of seawater several thousand-fold their body volume every 24 hours and remove 80%–90% of particles in the size range from 0.2 to 50 μm (Reiswig 1974; Pile, Patterson and Witman 1996), predominantly bacterioplankton and picoplankton (Maldonado et al. 2010). The proposed concept of the ‘sponge loop’ (de Goeij et al. 2013) considers the role of this process in accelerating the remineralization of organic matter, contributing to enhanced elemental turnover and low net export inside coral reefs (Maldonado 2015). With the metabolic capabilities provided by their bacterial partners, sponges can greatly expand their metabolic capacity and gain competitive edges in oligotrophic environments (Taylor et al. 2007; Thomas et al. 2010; Hentschel et al. 2012; Webster and Taylor 2012) where they could face food limitation (Pawlik et al. 2015). This review examines the role of microbial symbionts of marine sponges in cycling of carbon (C), nitrogen (N), phosphorus (P) and sulfur (S).
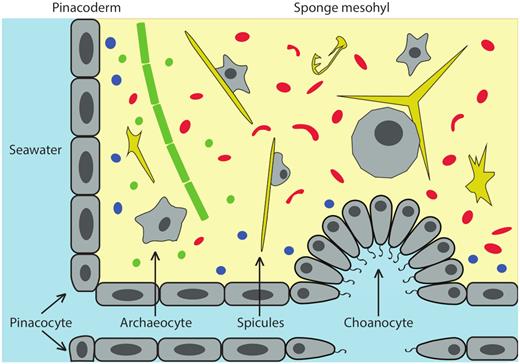
An illustration of the internal structure of a typical demosponge and the microbial symbionts. Major sponge cell (grey) types include pinacocytes, building blocks of the pinacodermal layer; archaeocytes, amoeba-like cells responsible for feeding and cell differentiation; and choanocytes, which form choanocyte chambers to harvest particles in the flow-through seawater. Through the choanocyte chambers, microorganisms from seawater are transported to the sponge mesohyl, where they are either engulfed by archaeocyte cells as food source or evade phagocytosis and become symbionts in their hosts. Diverse symbiotic microorganisms exist in the mesohyl of many demosponges. Representative microbial symbionts are cyanobacteria (green), archaea (blue) and heterotrophic bacteria (red). Siliceous spicules (yellow) act as structural skeleton to support to the morphology of demosponges.
MICROBIALLY MEDIATED C CYCLE IN SPONGES
The major forms of C in marine biogeochemical cycles are inorganic C in the form of bicarbonate and carbon dioxide (CO2) that is utilized in the process of photosynthesis and C fixation, and total organic carbon (TOC). TOC is comprised of three pools: dissolved organic carbon (DOC); living particulate organic carbon (LPOC), consisting of phytoplankton, bacterioplankton, cyanobacteria and zooplankton and detrital particulate organic carbon (detrital POC).
Most work on C cycling of sponges has been done on sponges from coral reef ecosystems, although there are also some studies on deep-water sponges. By heterotrophic feeding, sponges can quickly deplete POC and DOC in the surrounding water column (Reiswig 1971; Vogel 1977). The largest quantities of DOC in coral reefs stem from two primary sources: algae and corals. Marine algae release DOC as a photosynthetic byproduct (Wild et al. 2004, 2010; Haas and Wild 2010; Haas et al.2011, 2013; Mueller et al. 2014b) and corals release DOC in the form of mucus (Ferrier-Pagès et al. 1998; van Duyl and Gast 2001; Wild et al. 2004; Rix et al. 2016). DOC from within the coral reef ecosystem rather than from open ocean phytoplankton and originating primarily from coral mucus and algae was shown to be the primary nutrition source for marine sponges and their symbionts through stable isotope (δ13C, δ15N) and fatty acid biomarkers (van Duyl et al. 2011).
Seminal studies by Reiswig nearly 50 years ago investigated filtration rates of sponges (Reiswig 1974) and their role in C uptake (Reiswig 1971, 1981) by probing expelled water. Many studies have subsequently demonstrated removal of POC by heterotrophic feeding of sponges (Pile et al. 1997; Gili and Coma 1998; Ribes, Coma and Gili 1999; Yahel, Sharp and Marie 2003; Hadas, Shpigel and Ilan 2009; Leys et al. 2011) with bacterial retention rates up to 99% (Pile 1997). Some sponges have been shown to select for LPOC (Yahel, Eerkes-Medrano and Leys 2006; Hanson et al. 2009; Maldonado et al. 2010; McMurray et al. 2016) while conversely, detritus constituted 54% of the POC consumed by the sponge, Negombata magnifica (Hadas, Shpigel and Ilan 2009). Generally, particles approximately 1 µm in diameter are captured most efficiently (Turon, Galera and Uriz 1997; Ribes, Coma and Gili 1999). Within LPOC specifically, sponges preferentially select particle sizes <10 µm (Pile, Patterson and Witman 1996; Turon, Galera and Uriz 1997; Ribes, Coma and Gili 1999; Coma et al. 2001; Yahel et al. 2007). Sponges have also been shown to feed on virus particles (Hadas et al. 2006).
Many studies address the question of whether sponges obtain the majority of their C from POC, DOC or as a result of C fixation by photosynthetic symbionts. POC was shown to account for the vast majority of total C obtained by filtration for the temperate, hard-bottom dwelling sponge, Dysidea avara (Ribes, Coma and Gili 1999), with DOC intake being insignificant. In many reef sponge species, POC accounts for only a small proportion of sponge respiration and growth requirements. Even when POC filtration rates were high, the majority of the C budget was found to be fulfilled by ingestion of DOC (e. g. Pile 1997; Ribes, Coma and Gili 1999; Mueller et al. 2014a; Morganti et al. 2017; reviewed by Pawlik et al. 2015; Pawlik, Loh and McMurray 2018). DOC has been shown to comprise the majority of C in the diets of many sponges that harbor abundant bacterial symbionts. One recent study similarly indicated that DOC is the dominant organic C source (96% of TOC) for Xestospongia muta and can fuel all of the sponge's respiratory needs (Hoer et al. 2018). Additionally, Wooster et al. (2019) found that over 60% of the diet of the giant barrel sponge Xestospongia testudinaria was DOC.
The sponge holobiont differentiates between coral-derived and algal-derived DOC. In a study of three Red Sea reef sponges, algal-derived DOC was found to be incorporated at significantly higher rates overall, primarily into bacteria-specific fatty acids within the sponges whereas coral-derived DOC was primarily incorporated into sponge-specific fatty acids (Rix et al. 2017).
DOC availability and uptake has been shown to be particularly high in reef crevices (de Goeij and van Duyl 2007). DOC removal by three sponges that have been shown to dominate crevice communities accounted for on average more than 90% of the TOC removal; DOC removal rates in these sponges were two orders of magnitude higher than the C obtained from feeding on bacteria (de Goeij et al. 2008a). Further, two boring sponges also relied heavily on DOC: 80% of the TOC filtered in one study was found to be DOC (Mueller et al. 2014a). DOC uptake rates of cryptic reef crevices were shown to potentially exceed gross primary production of the entire reef system (de Goeij and Van Duyl 2007; de Goeij et al. 2008a). Sponges are the primary organisms responsible for this uptake, having removal rates two orders of magnitude higher than bacterioplankton (de Goeij et al. 2008a) and taking up a high proportion of DOM in the surrounding water (de Goeij et al. 2013). Encrusting sponges living in reef cavities tend to have short cell cycles and fast cell shedding, acting as ‘sponge pumps’ that convert ambient dissolved nutrients into particulate forms (primarily by shedding of choanocyte cells) enhancing organic C cycling in the reef environment (de Goeij et al. 2013; Alexander et al. 2014). In several sponge species, there were differing ratios of choanocyte and mesohyl cells in the POM produced by the sponges (Maldonado 2015). In a study of five HMA and four LMA sponges in the Caribbean, none of these nine massive, emergent sponge species produced large amounts of POM; in these sponges, transfer of C to higher trophic levels may be through grazing on the sponges by invertebrate, turtle and fish spongiovores (McMurray et al. 2018). X. testudinaria in the Red Sea was also found not to return C in the form of sponge cells or detritus into the reef environment (Wooster et al. 2019). Therefore, the production of sponge biomass may be more important than production of particulate detritus in this sponge as well (McMurray et al. 2018).
For deep-sea sponges, C availability may be from POC, DOC or inorganic C fixation. On the continental shelf of the northeast Pacific, POC accounted for the entire C budget of Aphrocallistes vastus and Rhabdocalyptus dawsoni (Yahel, Eerkes-Medrano and Leys 2006; Yahel et al. 2007; Kahn et al. 2015). Similarly, Pile and Young showed ultraplankton (<5 µm) made up the diet of Sericolophus hawaiicus (Pile and Young 2006). However, Higginsia thielei and Rosella nodastrella from the deep Atlantic were shown to fix C and assimilate DOC. Bicarbonate may be fixed by chemoautotrophic or mixotrophic nitrifying prokaryotes in these sponges (van Duyl et al. 2008). In the sponge Geodia barretti from 200 m depth in the Norwegian Sea, bacteria were removed by sponge filtering at 99% efficiency; however, bacteria provided only 5% of the sponges’ energy requirements with the rest being supplied by DOC and detritus (Leys et al. 2018). In the deep ocean environment, glass sponges, while having low bacterial densities, are particularly adept at capturing the small amounts of POC available at depth (Leys et al. 2011).
Microbial symbionts can be actively involved in DOC uptake and breakdown, and HMA sponges tend to have higher DOC uptake ability than the LMA sponges, suggesting that bacteria might play an important role in DOC uptake processes (de Goeij et al. 2008b). Stable isotope δ13C tracer studies also showed strong indications of reef origins in the tissue of coral cavity sponges, suggesting an important source for C diet in these sponges (van Duyl et al. 2011). Field measurements showed high sponge respiration rates, often exceeding C ingestion rates (Reiswig 1974; Coma et al. 2002). Measured by increased volume or weight, the growth of sponges is generally slow at 5%–60% per year for tropical and temperate sponges (Reiswig 1973; McMurray, Blum and Pawlik 2008). The rate can drop dramatically for cold water sponges to 0.07% per year (van Duyl et al. 2008).
Sponges can also obtain C from their photosynthetic symbionts (Wilkinson 1987; Freeman and Thacker 2011). Net primary production was detected in sponges hosting cyanobacterial symbionts and not in sponges without cyanobacterial symbionts, providing strong evidence for the importance of microbes in the sponge C cycle in some cases (Wilkinson 1983). Cyanobacterial symbionts, most commonly Synechococcus, are major contributors of C to several different sponges in a range of geographic locations (Erwin and Thacker 2007; Usher 2008 and references therein). In Caribbean sponges associated with various clades of cyanobacteria, nutritional benefits were variable and, in some cases, the cyanobacterial symbionts had a substantial positive effect on host sponge growth (Erwin and Thacker 2008). The identity of both sponges and their symbionts affects rates of C transfer more than the abundance of the photosynthetic symbionts (Freeman et al. 2013). Some sponges can gain C nutrition from zooxanthellae capable of photosynthetically fixing C (Weisz et al. 2010).
Overall, there is a great diversity of processes whereby sponges can obtain C, and microbial symbionts within those sponges can play a variety of roles, including contributing to C fixation through photosynthesis or inorganic C fixation and enhancing uptake of DOC.
MICROBIALLY MEDIATED N CYCLE IN SPONGES
The N cycle within sponges is complex and has been well studied over the past 40 years. In nature, the transformation of different N species by N fixation, nitrification, denitrification and anaerobic ammonia oxidation is conducted exclusively by prokaryotes and every known mechanism of N transformation has been detected in sponges. For example, a recent study examined each N transformation in the microbial symbionts of one sponge, Hymeniacidon heliophila, using a predictive metagenomic approach to show that the H. heliophila holobiont can contribute to a diverse array of N transformations. In addition, this study compared predicted metagenomes to one shotgun-sequenced metagenome and many of the predicted N cycling genes were present in the actual metagenome as well as in the predicted metagenomes (Weigel and Erwin 2017). The core phyla in H. heliophila were shown to be generally affiliated with at least one N-cycling gene, suggesting that taxa with N-cycling potential are dominant members of the microbiome in this sponge. Interestingly, based on these predictions from amplicon data, Proteobacteria may be the only phylum that has the potential to contribute to all N transformations; other major contributors were Planctomycetes, Actinobacteria, Cyanobacteria, Bacteroidetes and Verrucomicrobia (Weigel and Erwin 2017).
N cycling between sponges and their symbionts, and efflux of N compounds to ambient waters exists in a tightly coupled web. Each product ultimately becomes a reactant in a subsequent reaction or is pumped out of the sponge body. Sponges can act as both a source and a sink of bioavailable N to both their symbionts and the surrounding reef ecosystem.
In N fixation, atmospheric nitrogen (N2) can be fixed into ammonia (NH3) by sponge bacterial symbionts. The first evidence of N fixation was the detection of activity of the nitrogenase enzyme complex, responsible for the reduction of N2, in sponges with cyanobacterial symbionts (Wilkinson and Fay 1979). Nitrogenase activity was detected through acetylene reduction assays of both sponges with autotrophic symbionts (Wilkinson and Fay 1979; Wilkinson, Summons and Evans 1999) and heterotrophic sponges (Shieh and Lin 1994). By hosting cyanobacteria and heterotrophic bacteria carrying the nitrogenase gene cluster, the holobiont can acquire reduced N through N2 fixation by its symbionts. Three genes (nifD, nifK, nifH) are expressed to form the nitrogenase complex. The diazotrophic community in sponges was first characterized by detection of nifH gene expression in Ircinia strobilina and Mycale laxissima (Mohamed et al. 2008). The presence and activity of N-fixing bacteria in these and additional sponges has since been confirmed in several studies (e.g. Zhang, Vicente and Hill 2014; Ribes et al. 2015).
N2 fixation activity in sponges can be deduced by N-isotope fractionation measured as δ15N values. The proportion of N obtained by biological N2 fixation has been examined through stable isotope analysis (Wilkinson, Summons and Evans 1999; Weisz et al. 2007; Zhang, Vicente and Hill 2014; Ribes et al. 2015). Caribbean sponges can be clustered into three major groups: HMA sponges with high δ15N, HMA sponges with low δ15N and LMA sponges with high δ15N. No differentiation of stable isotope fractions was observed for δ13C values (Southwell et al. 2008). The low δ15N values found in some HMA sponges suggest that those sponges rely on biological N2 fixation to fulfill their N demand whereas most LMA sponges mainly obtain N by filter feeding and dissolved N uptake (Weisz et al. 2007). A subsequent survey examining both isotope indicators and chlorophyll abundance provides evidence that hosting symbionts expands the ecological niche of the hosts (Freeman, Easson and Baker 2014; Freeman et al. 2015). By hosting cyanobacteria or heterotrophic bacteria carrying the nitrogenase gene cluster, the holobiont can acquire reduced N by symbiotic N2 fixation.
The importance of cyanobacteria in the sponge microbiome is well-documented, from the unicellular cyanobacterium Synechococcus spongiarum in many sponge Xestospongia species (Usher et al. 2004; Burgsdorf et al. 2015) and the species-specific filamentous cyanobacterium Oscillatoria spongeliae, with genetic distinction from their planktonic counterparts in the water column (Thacker and Starnes 2003). The first molecular characterization of a diazotrophic community in sponges found nifH genes were exclusively expressed from cyanobacteria (Mohamed et al. 2008). A subsequent study on the same sponge species indicated that the filamentous cyanobacterium Leptolyngbya dominated the nifH gene transcript data set (Zhang, Vicente and Hill 2014), suggesting that cyanobacteria are key symbionts in the sponges studied.
O2, NH3 and H2S are major factors that affect N fixation. N fixation requires anaerobic conditions (as do anammox and denitrification discussed in later sections), and these conditions can be found in the inner mesohyl region of sponges, where O2 can be depleted by intense cell respiration and limited diffusion from the surrounding water. An elegant in situ study on the sponge Theonella swinhoei showed that temporal microenvironments within this sponge provide niches suitable for anaerobic microbes (Lavy et al. 2016). N fixation must occur in such anoxic regions within the sponge body or in anaerobic compartments within bacteria, such as the specialized heterocyst compartments made by cyanobacteria that limit the diffusion of O2 (Popa et al. 2007; Fiore et al. 2010).
N fixation by bacterial symbionts may not be necessary to provide NH3 to the sponge and its microbial community in all cases. Low N fixation rates or low nifH expression have been found in some sponges, suggesting that N fixation is not ubiquitous in sponges and not all sponges contribute significantly to reef nutrient inputs (Ribes et al. 2015). In these sponges, dissolved inorganic nitrogen (DIN) is most likely predominantly sourced from the remineralization of organic matter. In several cases, sponges that obtain much of their N from fixation and have abundant nifH gene expression also have microbial communities dominated by cyanobacteria, which may be providing a competitive advantage to their sponge hosts, and could also be an significant source of fixed N to reefs (Mohamed et al. 2008; Zhang, Vicente and Hill 2014).
Given the cost of fixing nitrogen when fixed nitrogen is in ample supply, NH3 can suppress N fixation (Kamberger 1977) but only recently has it been discovered that H2S may act as a positive signaling molecule to promote N fixation (Zou et al. 2018). The NH3 produced by diazotrophs within the sponge body serves as a precursor to nitrification.
Nitrification is the aerobic oxidation of NH3 into NO2− and subsequently into NO3−. The NH3 is supplied either by N fixation or the remineralization of POM. The first nitrifying step is the oxidation of NH3 to NO2− and is performed by aerobic ammonia-oxidizing bacteria (AOB) and archaea (AOA). The second step of nitrification is the further oxidation of NO2− to NO3− and is carried out by nitrite oxidizing bacteria (NOB). All known AOB are phylogenetically affiliated with the Beta- and Gammaproteobacteria and were once thought to be the only major NH3 oxidizers. It was later discovered that Crenarcheota were also able to oxidize NH3, and the AOA community within sponges has been well-studied (Rotthauwe, Witzel and Liesack 1997; Karner, Delong and Karl 2001, Francis et al. 2005; Treusch et al. 2005; Hallam et al. 2006a,b; Francis, Beman and Kuypers 2007; Bayer, Schmitt and Hentschel 2008; Steger et al. 2008; Mohamed et al. 2010; Tian et al. 2016). AOA have been hypothesized to be the main drivers of NH3 oxidation due to higher transcription levels of key genes in these microbes (Radax et al. 2012). All known NOB in sponges belong to the genera Nitrobacter (Alphaproteobacteria), Nitrococcus (Gammaproteobacteria), Nitrospina (Deltaproteobacteria), and the phylum Nitrospira (Bayer, Schmitt and Hentschel 2008; Mohamed et al. 2010; Tian et al. 2016).
The first evidence of microbial nitrifiers was discovered by Corredor et al. (1988) after detecting a substantial release of NO3− by a Caribbean reef sponge. Sponges excrete NH3 into ambient water but an efflux of NO3− (and more rarely, NO2−) has also been found in many sponges (Diaz and Ward 1997; Jimenez and Ribes 2007; Southwell, Popp and Martens 2008; Southwell et al. 2008). Release of NO3− is interpreted as evidence for nitrification because no other chemical process could account for the NH3 transformation (Diaz and Ward 1997). This interpretation has been reinforced by the amplification and sequencing of the 16S rRNA gene sequences belonging to known nitrifiers (Hentschel et al. 2002; Diaz, Akob and Cary 2004; Hallam et al. 2006a,b; Taylor et al. 2007; Bayer, Schmitt and Hentschel 2008; Radax et al. 2012; Ribes et al. 2012; Zhang, Vicente and Hill 2014). Further support for nitrification comes from the discovery of the gene responsible for the enzyme that catalyzes NH3 oxidation, amoA, in several sponge species (Bayer, Schmitt and Hentschel 2008; Meyer and Kuever 2008; Southwell et al. 2008; Hoffman et al. 2009; Lopez-Legentil et al. 2010; Mohamed et al. 2010; Fan et al. 2012; Han et al. 2012; Ribes et al. 2012; Yang and Li 2012; Zhang, Vicente and Hill 2014). AOA in sponges tend to have very low diversity and high stability (Bayer, Schmitt and Hentschel 2008; Radax et al. 2012; Zhang, Vicente and Hill 2014), while a different phylotype of AOA was observed in bleached sponges (López-Legentil et al. 2010). NH3 oxidizers, both AOAs and AOBs, have also been discovered to be vertically transmitted to sponge larvae (Sharp et al. 2007; Steger et al. 2008; Webster et al. 2010).
Nitrification has been shown to be nearly ubiquitous within sponge populations regardless of microbial abundance (Schläppy et al. 2010), depth (Li et al. 2016) or water temperature (Radax et al. 2012). Subina, Thorat and Gonsalves (2018) detected differing nitrification rates and different ammonia oxidizers within the distinct sections of a sponge. The nitrification rate in the sponge cortex was found to be twice as high than in the intercellular and extracellular sections. Nitrifying archaea dominated in the intracellular section whereas nitrifying bacteria dominated in the intercellular and extracellular sections and the cortex.
It is important to highlight the various fluxes of DIN out of the sponge body, which are highly variable between sponge species and habitat conditions. DIN efflux is often NO3− (Corredor et al. 1988; Diaz and Ward 1997; Jimenez and Ribes 2007; Southwell, Popp and Martens 2008; Perea-Blázquez, Davy and Bell 2012; Keesing et al. 2013), especially in sponges containing cyanobacterial symbionts (Diaz and Ward 1997). In other cases, DIN efflux is predominantly NH3 (Hatcher 1994; Keesing et al. 2013; Kahn et al. 2015). Interestingly, Fiore, Baker and Lesser (2013) found fluxes of both NO3− and NH3 from X. muta depending on habitat location. Nitrification rates must be considered net rates because NO3− and NH3 are used to fuel denitrification and anammox, respectively (Hoffman et al. 2009), and this coupling of nitrification and denitrification may explain some of the observed variation in nitrification rates (Schläppy et al. 2010; Radax et al. 2012).
Corredor et al. (1988) first reported that Chondrilla nucula could contribute anywhere from 50% to 120% N needed to sustain primary production in a coral reef off Puerto Rico where this sponge is ubiquitous and abundant. Sponge-mediated nitrification rates might be 2 to 4 orders of magnitude higher than sediment processes in certain marine environments with a high density of sponges (Diaz and Ward 1997; Hoffmann et al. 2009; Radax et al. 2012). Because the process sits in the middle of the N biogeochemical cycle, nitrification is tightly coupled with all other N transformations. It is rate limited by the influxes of NH3 from N fixation and remineralization of POM, while subsequently influencing rates of N assimilation by phytoplankton, annamox and denitrification (Jimenez and Ribes 2007). Nitrification acts as the central transformation within the N biogeochemical cycle, so the bacteria and archaea within sponges that perform this process are key to N cycling within marine ecosystems in which sponges are abundant.
The two pathways, denitrification and anaerobic ammonia oxidation (‘anammox’) whereby NH3, NO2− and NO3− are converted to N2 are both anaerobic processes, though denitrification can also occur aerobically (Hayatsu, Tago and Saito 2008). Denitrification converts NO3− to N2, while anammox converts NH3 + NO2− to N2. Within the sponge body, nitrification and N fixation are tightly coupled with denitrification and anammox as the former processes provide the substrates (NH3, NO2− and NO3−) for the latter.
Crenarchaeotes and Euryarchaeotes have been found to be abundant in several sponge species (e.g. Preston et al. 1996; Webster, Watts and Hill 2001a; Jackson et al. 2013). Crenarchaeota have been speculated to be facultative anaerobes and denitrifiers (Sinninghe Damsté et al. 2002). Crenarchaeotes and Euryarchaeotes have also been found in Astrosclera willeyana (Yang and Li 2012) and have been a focus of broad phylogenetic studies (Holmes and Blanch 2007). Anaerobic regions and anammox activity are present within the sponge G. barretti (Hoffman et al. 2009; Brück et al. 2010), and this study provided the first proof of nitrification, denitrification and anammox all occurring within the same sponge host, which would require aerobic and anaerobic regions within the sponge body (Hoffman et al. 2005).
Potential anammox bacteria, Planctomyces, were found in sponges M. laxissima and I. strobilina (Mohamed et al. 2010). A subsequent study on X. muta confirmed the presence of both of the N removal pathways (Fiore, Baker and Lesser 2013) and transcriptomic data showed an exchange of metabolic pathways between symbionts and host, indicating a close symbiotic relationship in X. muta (Fiore et al. 2015).
Denitrification requires four enzymatic reaction steps that are encoded by eight genes: nitrate reductase (napA or narG), nitrite reductase (nirK or nirS), nitric oxide reductase (qnorB or cnorB), nitrous oxide reductase (nosZ) and nitrate/nitrite antiporters (narK) (Fan et al. 2012; Han, Li and Zhang 2013; Zhang et al. 2013). To date, all eight genes have been detected within at least 12 species of sponges, though different species use alternative pathways (Fan et al. 2012; Yang and Li 2012; Han, Li and Zhang 2013; Zhang et al. 2013; Karimi et al. 2018). Weigel and Erwin (2017) found that the shotgun sequenced metagenome of a single sponge, H. heliophila, contained genes for all steps for complete denitrification by the denitrification pathway: nitrate reductases, nitrite reductases, nitric oxide reductases and nitrous oxide reductase. It is also of note that some sponge microbial communities have incomplete pathways of NO3− reduction (Siegl et al. 2011; Fan et al. 2012).
Few studies have directly detected denitrification and anammox processes within the sponge holobiome even though the microbes and genes associated with denitrification and anammox have been confirmed in many sponges. Considering anaerobic ammonia oxidizing bacteria (AnAOB) are estimated to contribute to 30%–50% of N loss from the ocean (Codispoti et al. 2001; Mohamed et al. 2008), this process should be an important future research focus.
Sponges can act as a source of NH3 for their associated prokaryotic community (Davy et al. 2002; Fiore, Baker and Lesser 2013) and as a source of NH3 and NO3− to the surrounding reef environment. Sponges act as sources of DIN more often than as sinks (Southwell et al. 2008; Fiore, Baker and Lesser 2013). In a study in which NH3 and NO3− plus NO2− fluxes were measured from many sponges in a Florida Keys reef system, overall DIN flux rate was estimated to be 640 ± 130 µmol m−2 h−1 (Southwell et al. 2008). DIN can often be eliminated through denitrification and anammox, which can be affected by changes in sponge pumping rates. Changes in O2 concentrations by sponge pumping would disrupt anaerobic microhabitats, which would in turn influence denitrification and anammox rates and N products (Schönberg, Hoffmann and Gatti 2004; Fiore et al. 2010; Schläppy et al. 2010).
Various sponges have been found to host distinct microorganisms that perform equivalent functions in N fixation, nitrification, denitrification and anammox. There is a gap in knowledge of the mechanisms that drive whether a sponge will release NH3 or go through further oxidation steps to release NO3−. Few studies have yet to link the efflux of DIN with the identification of the corresponding microbial phylotypes. A combination of isotope tracer studies, flux measurements and molecular community characterization can provide a more comprehensive view on microbial functionality in N nutrient cycles.
MICROBIALLY MEDIATED P CYCLES IN SPONGES
P is an important building block of several essential biological compounds such as DNA, RNA, ATP and the phospholipid cell bilayer. In the marine environment, P is generally supplied in both dissolved and particulate forms via rivers and runoff (Paytan and McLaughlin 2007). P in the marine environment is transformed between a variety of inorganic and organic forms through microbially mediated mechanisms, comprehensively reviewed by Karl (2014). P is known to be an essential macronutrient that influences primary production, while simultaneously regarded as the ultimate limiting factor within marine systems over geological time (Redfield 1960; Van Cappellen and Ingall 1994; Toggweiler 1999; Tyrell 1999; Benitez-Nelson 2000).
Polyphosphate (polyP) is a multi-chained linear or circular compound comprised of tens to hundreds of phosphate (PO43−) molecules and is found in almost all organisms as a major energy storage compound (Kornberg, Rao and Ault-Riché 1999). The significance of polyP in the microbial and marine world has been well reviewed, especially within nutrient depleted waters (Temperton et al. 2011; Martin et al. 2014). Considering the abundance of sponges in oligotrophic coral reefs, the amount of polyP found within sponges is likely to have an effect on the total biogeochemistry of P (Zhang et al. 2015a). Although microbially mediated P transformations can have significant implications in microbial ecology, it is a vastly understudied process in sponge microbiology (Taylor et al. 2007; Maldonado, Ribes and van Duyl 2012; Karl 2014).
Some studies have measured PO43− (commonly referred to as orthophosphate, Pi) efflux from sponges (Hatcher 1994; Jimenez and Ribes 2007; Yahel et al. 2007), though only a handful have made Pi the main research focus. One study taking efflux measurements from three sponges showed Pi release of 20%, 30% and 60% for D. avara, Chondrosia reniformis and Agelas oroides, respectively, suggesting that sponges may be important recyclers of P in the coral reef communities (Ribes et al. 2012). This release was not correlated with microbial abundance or phylogeny. Another study found that actinomycete sponge endosymbionts are able to solubilize PO43−compounds, potentially providing a bioavailable form of P for the holobiont (Sabarathnam et al. 2010).
The majority of work of the biogeochemical transformations of P has been focused on the role of polyP within sponges and their symbionts. PolyP was found in a freshwater sponge, Ephydatia muelleri, and concentrations were shown to change during development and exposure to polluted water (Imsieke et al. 1996). Sequestration of polyP in the sponge body was found in three marine sponges and between 25% and 40% of total P within these sponges was in the form of polyP (Zhang et al. 2015a). Apatite, a non-labile calcium-phosphate mineral, was also detected in the same three sponges, raising the possibility that sponges may act as P sinks. Additionally, comparative genome analysis found a S-oxidizing sponge symbiont exclusively utilized polyP instead of ATP for glucose phosphorylation (Tian et al. 2014).
Microbes shifting between polyP formation and degradation is well-studied within sewage treatment plants, where redox conditions are controlled by alternating aerobic and anaerobic phases (McMahon and Read 2013). The same mechanisms may be occurring within sponges. Water flowing through the mesohyl facilitates a redox gradient suitable for both polyP accumulation and degradation. During periods of active pumping, the mesohyl is oxygenated and the bacterial symbionts can store ambient phosphate as polyP. Oxygen levels in the mesohyl can be quickly depleted when pumping ceases, which could lead to polyP degradation and Pi release (Zhang et al. 2015a).
P can be present in sponges in unusual forms. For example, two new phosphoiodyns were isolated from a sponge Placospongia sp. found in Korean waters. These compounds are very unusual in that they contain P atoms, which are very seldom found in natural products. Phosphoiodyn A has a C-P bond not previously found in marine sponges (Kim et al. 2013). The form of P which is incorporated in these compounds and the role of the compounds in P storage are unclear.
Sponges may act as either a sink or a source of P, and their primary role in P biogeochemistry is not yet clear. On one hand, sponges have been shown to release bioavailable Pi. By effectively concentrating P from surrounding waters and releasing polyP and/or Pi, sponges may play a key role in providing P to other organisms in the coral reef environment that would otherwise be unable to obtain sufficient P from the low-nutrient waters. On the other hand, sponges may also be playing a role in apatite formation, which would result in P being deposited and lost in sediments (Colman 2015). Cycling of P inside sponges might favor apatite formation over polyP hydrolysis, which would increase burial of P and result in ‘long-term geological sequestration’ (Zhang et al. 2015a). It is unknown what implications this removal of previously bioavailable PO43− might have for the already P-limited reef. P sequestration by sponge symbionts may be important in controlling the P availability in the coral reef water column. The key question is how important this polyP-mediated pathway is in coupling the pelagic-benthic P fluxes. To answer this question, in situ measurements of dissolved and particulate P fluxes are needed to uncover diurnal patterns and seasonal variations (Colman 2015).
There is a clear research gap regarding the form in which sponges release P. Do sponges facilitate the release of P either in (a) the restored available form of Pi (b) as polyP or (c) as recalcitrant apatite lost within sea sediment? The change in concentration of dissolved organic P and particulate P in water entering and leaving sponges needs further study (Colman 2015). One challenge in studying P cycling is that there are no viable stable isotopes of P. Experimental tracing of P must be accomplished using radiolabeled isotopes 32P or 33P. As radioisotopes may not be suitable for in situ experiments, the stable oxygen isotope ratio of phosphate, δ18Op, can be used (Blake et al. 2005; Davies, Surridge and Gooddy 2014). Using δ18Op, in situ measurements can examine the intra- and extracellular reaction mechanisms that control P cycling within the sponge body and uncover correlations in fluxes with diurnal and seasonal variations (Coleman 2015). Coupled with metagenomic and transcriptomic tools, future studies may provide additional information on the diversity and stability of microbes that play roles in polyP production and degradation. If the accumulation of P by sponge symbionts directly benefits the holobionts, this would be one of the first instances of direct nutrient storage by bacteria for their host (Colman 2015).
MICROBIALLY MEDIATED S CYCLES IN SPONGES
Given the high concentrations of sulfate (SO42−) in seawater and the presence of sulfonated polysaccharides and lipids in the sponge extracellular matrix (Zierer and Mourao 2000; Vilanova, Coutinho and Mourão 2009), SO42− is likely an energy source for microbial symbionts of sponges. SO42− reduction is tightly coupled with carbon metabolism as heterotrophs that consume C use SO42− as an electron acceptor for respiration, while sulfate oxidizing bacteria (SOB) use the energy generated from the oxidation of H2S (and other reduced forms of S) to assimilate inorganic C. S oxidation, S reduction and possible coupling between these processes, occurs in sponges and the specialized symbionts that utilize S compounds occur within anoxic spaces of sponges (Hoffman et al. 2005; Mohamed et al. 2008). Interestingly, the genus Spongiobacter, capable of metabolizing dimethylsulfoniopropionate and dimethyl sulfide, is present in corals and has been suggested to be an important player in S cycling in corals (Raina et al. 2009). Spongiobacter has been found associated with I. strobilina and M. laxissima (Mohamed et al. 2008).
Genomic insights have revealed the possible capabilities of S metabolism within the sponge microbiome. For example, genome analyses of six sponges found genes encoding for sulfatases, important for both synthesis and cleavage of SO42− esters (Kamke et al. 2013). Sulfate-reducing bacteria (SRB) have been found in many sponges (Imhoff and Trüper 1976; Schumann-Kindel et al. 1997; Manz et al. 2000; Hoffmann et al. 2005; Hoffmann, Rapp and Reitner 2006; Taylor et al. 2007). Members of the genus Desulfovibrio have been implicated in SO42− reduction in sponges (Ahn, Kerkhof and Häggblom 2009; Zhang et al. 2015b; Lavy et al. 2018). In the deep ocean, SO42− reduction rates of G. barretti was found to be up to 1200 nmol SO42− per cm3 sponge per day (Hoffmann et al. 2005). SO42− reduction generates toxic sulfide (S2−), which can be mitigated by SOB. Sequences of potential sulfide-oxidizing bacteria, Chromatiales, have been found in Aplysina fulva, Sarcotragus spinosulus and T. swinhoei (Hardoim et al. 2009, 2012; Lavy et al. 2018, respectively). Additionally, purple S bacteria have been found in the microbial community of Axinella corrugata (White et al. 2012) while green S bacteria were found in Rhopaloeides odorabile (Webster et al. 2001b). A draft genome of an intercellular SOB (‘HK1’) was assembled from a metagenome of the sponge Haliclona cymaeformis (Tian et al. 2014). Subsequent draft genomes of putative SOBs have been assembled from Lophophysema eversa (Tian et al. 2016), Amphimedon queenslandica (Gauthier, Watson and Degnan 2016), Suberites sp (Tian et al. 2017) and Spongia officinalis (Karimi et al. 2018). Interestingly, AqS1 from A. queenslandica (Gauthier, Watson and Degnan 2016) seems to be closely related to HK1 from H. cymaeformis (Tian et al. 2014). In all cases, genome sequences suggest that these symbionts are capable of S oxidation. A recent genomic analysis (Karimi et al. 2018) revealed the versatile S utilization capacity of Rhodospirillaceae from S. officinalis, including the possibility that Rhodospirillaceae use taurine as an organic S source.
Simultaneous SO42− reduction and S2− oxidation—as found in marine oligochaetes (Dubilier et al. 2001; Blazejak et al. 2005)—is hypothesized to occur in sponges in order to detoxify S2− (Hoffmann et al. 2005; Taylor et al. 2007). Both SOBs and SRBs were present in the deep-sea sponge, Polymastia cf. corticata and contained the gene aprA that encodes a key enzyme in SO42− reduction and S2− oxidation (Meyer and Kuever 2008). Transcription of aprA provided more evidence for SOB, SRB and SRA (sulfate-reducing archaea) functioning together in a sponge-specific endosymbiotic S cycle (Jensen et al. 2017). Lavy et al. (2018) showed by genome analysis that both SO42− reducers (Desulfovibrio) and oxidizers (novel Chromatiales) are present in T. swinhoei. Complete cycling of S is suggested as Desulfovibrio would reduce SO42− to S2− and the Chromatiales would transform S2− back to SO42− (Lavy et al. 2018).
SPATIAL AND TEMPORAL HETEROGENEITY
The conceptual diagram (Fig. 2) illustrates the potential temporal and spatial separation in functional microbial groups. For example, aerobic processes like photosynthesis and aerobic ammonia oxidation are located in the epidermal layer, where the environmental requirements like light penetration and oxic state can be met. Anaerobic processes like N2 fixation, anammox and denitrification are located in the inner mesohyl, where O2 can be depleted by reduced diffusion and intense cell respiration (Hoffman et al. 2009; Lavy et al. 2016). Facultative anaerobes may occupy a niche oscillating between aerobic and anaerobic conditions. Shifting redox state in the sponge mesohyl reflects combinational effects from sponge host pumping, internal respiration and photosynthesis by symbionts. The diurnal cycle can play an important role in regulating the O2 tension in microbiomes with photosymbionts; it has been observed in coral that a distinct change occurs in the O2 concentration in the diffusive boundary layer during the diel cycle (Shashar, Cohen and Loya 1993). While sponge tissues are considered to be oxygenated most of the time, anaerobic conditions can happen during night-time when sponges stop pumping and O2 demand from respiration builds up (Hoffman et al. 2009) (Fig. 2B). Sponge pumping rates can vary greatly by morphology and size, although no consistent temporal rhythm has been reported so far (McMurray, Pawlik and Finelli 2014). Sponges can control O2 supply to their microbial symbionts and adjust functional pathways through pumping. This may change the diurnal redox state created by photosynthesis. Respiration rates in the holobiont can be influenced by temperature, host physiology and the density of microbial symbionts (Reiswig 1974; Osinga, Tramper and Wijffels 1999), adding complexity to the redox dynamic.
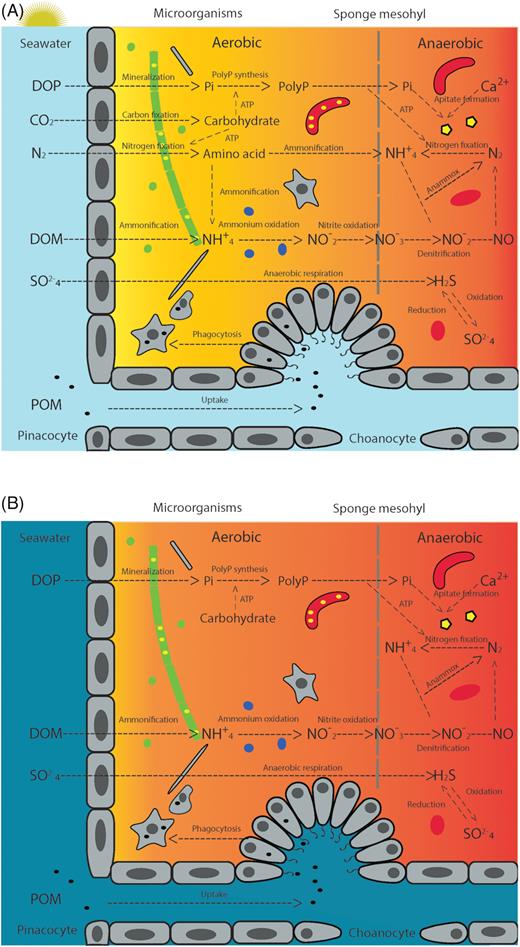
Conceptual diagram of microbially mediated nutrient cycles in sponge mesohyl during daylight (A) and night (B). Color gradient (yellow to red) background indicates O2 availability in sponge mesohyl, with yellow representing the most oxic state and red representing the most anoxic state. Microbial symbionts are divided in three groups: cyanobacteria (green), archaea (blue) and heterotrophic bacteria (red). PolyP granules (yellow) accumulated in microbial cells can contribute to the formation of apitate minerals (yellow pentagon) inside sponges. Sponge cells (grey) are involved in the uptake of DOM and POM from the surrounding seawater. Abbreviations: Dissolved organic phosphorus (DOP); Dissolved organic nitrogen (DON); Particulate organic matter (POM-illustrated as black dots); Phosphate (Pi); Polyphosphate (PolyP).
INTERTWINED NUTRIENT NETWORK
In the sponge holobiont, dynamic environmental gradients and diverse microbial residents would enable numerous nutrient cycling pathways. Several meta-transcriptomic and meta-proteomic studies on sponges have reported detailed gene and protein expression patterns in the associated microbial assemblages, showing complex C, N, and S cycles (Liu et al. 2012; Bayer et al. 2014; Moitinho-Silva et al. 2014a,b). Future omics studies will continue to depict genetic potential and functional activities of the sponge microbiome, although the link between sequence read abundance and the strength of the assigned pathway needs to be further confirmed (Prosser 2015). The possible interactions between nutrient cycles are illustrated in Fig. 2. Many interactions may occur within the N cycle alone (Fig. 2). For example, both AOB and AnAOB were detected in the same sponge (Mohamed et al. 2008). This along with evidence of paired nitrification and denitrification in both HMA and LMA sponges again confirms the notion of separate niches within sponges for different N processes (Schläpy et al. 2010).
The diazotrophic cyanobacteria, with their ability to perform C and N fixations, and P accumulation, might play a central role in shaping the major nutrient flow in the symbiotic microbiome structure. Carbohydrates fixed by cyanobacteria can generate energy for N fixation or stored through polyP synthesis. These P granules can serve as a back-up battery to produce ATP under energy starvation conditions like dark or anaerobic situations, maintaining cellular activities such as biosynthesis or N fixation. Loss of abundant species like cyanobacteria that play a central role in nutrient cycling would collapse existing ties with other microbial members in the network, compromising community homeostasis and host health. To untangle these interactions, sophisticated network analysis and quantitative models are necessary to generate insights with statistical support (Fuhrman, Cram and Needham 2015). There is also evidence demonstrating the interactions between elemental cycles; one cycle can exert constraints on another through specific microbes in the environment that play key roles in nutrient cycling (Hallam et al. 2006a,b; Carini et al. 2014). Future studies can combine molecular markers and elemental isotope signatures to assign microbial identities with metabolic functions and relative strength in nutrient fluxes.
Nano-SIMS can be particularly helpful in linking the microbial community structure with quantitative and spatial information on a nano-scale (Popa et al. 2007). Elements like C, N, O and S all have different stable isotopes, which can be applied as tracers in incubation experiments to understand the element flows in sponges. The deviation of the natural abundance of respective isotopes can reflect microbially mediated nutrient cycling. Although P has only one stable isotope 31P, the bonding with four O in phosphate, which has three stable isotopes (16O, 17O and 18O), theoretically can produce 15 phosphate isotopes, and the biological meaning of these phosphate isotopes is just beginning to be examined in various environments (Elsbury et al. 2009; Stout et al. 2014). These delicate measurements can be very challenging for environmental samples and complex biological tissues, but comparative studies on microbial cultures with PO43−rich granules could be a promising entry point. For example, comparison of C, N, O and S isotope ratios in cyanobacterial filaments with and without polyP granules by nano-SIMS could lead to clues connecting polyP metabolism with other nutrient cycles like C and N fixation with convincing spatial resolution.
Coral reef ecosystems are systems of sustainable high production despite oligotrophic conditions in the surrounding water. Increasing evidence suggests that habitat-forming species like corals and sponges are the leaders in creating this self-sustainable ecosystem. The increasing anthropogenic nutrient loading is a threat to many coral reefs (Koop et al. 2001). With the increasing abundance of sponges in many environments (Bell et al. 2013), research on the nutrient cycles mediated by sponge holobionts is important for untangling the intricacies of the symbioses and for better understanding the broad ecological impact of sponges.
Conflict of interest. None declared.
REFERENCES
Author notes
Shared first authors.
Present Address: Alkek Center for Metagenomics and Microbiome Research, Department of Molecular Virology and Microbiology, Baylor College of Medicine, Houston, TX 77030, USA.