-
PDF
- Split View
-
Views
-
Cite
Cite
Liu Tian-Tian, Huang Ping, Liu Jia-Xing, Ke Zhi-Xin, Tan Ye-Hui, Utilization of different dissolved organic phosphorus sources by Symbiodinium voratum in vitro, FEMS Microbiology Ecology, Volume 95, Issue 11, November 2019, fiz150, https://doi.org/10.1093/femsec/fiz150
- Share Icon Share
ABSTRACT
This study examines the physiological responses of the Symbiodiniumvoratum (clade E) to two types of phosphates having different chemical bonds—phosphoesters (C-O-P bonds) and phosphonates (C-P bonds) to explore Symbiodinium cell growth and the molecular perspective of the P utilization process. Alkaline phosphatase (AP), PhnX, PhoA and PhoX expression was profiled for different P conditions using the RT-qPCR method. In a sterile system, Symbiodinium could decompose phosphoesters, such as ATP and glucose 6-phosphate (G-6-P), into dissolved inorganic P (DIP) to supplement inorganic phosphorus but could not directly use phosphoesters for growth. The growth rate and photosynthetic efficiency of zooxanthellae in phosphoester-containing media did not significantly differ from those in the DIP group but were significantly inhibited in medium containing phosphonates such as N-(phosphonomethyl)glycine (glyphosate) and 2-aminoethylphosphonic acid (2-AEP), as well as in DIP-poor medium. The phosphonate group DIP concentration did not change remarkably, indicating that phosphonates can neither be directly used by zooxanthellae nor decomposed into DIP. Our RT-qPCR results support our views that the phosphoesters (C-O-P) had been hydrolyzed outside the cell before being absorbed into the Symbiodinium cell, and implies that PhnX, PhoA and PhoX are perhaps responsible for transporting DIP from medium into cells and for storage of DIP.
INTRODUCTION
The coral reef ecosystem is one of the most biologically diverse and highly productive marine ecosystems (Staley et al. 2017; Ranjbar Jafarabadi et al. 2018). The diversity of coral reef ecosystems is maintained by the complex coral reef habitats created by reef-building corals, which secrete calcium carbonate to provide important habitats for many other species (Rosic and Dove 2011; Staley et al. 2017). At the same time, the coral reef ecosystem also relies on the high nutrient productivity provided by Symbiodinium (Shore-Maggio et al. 2015). It has been proposed that coral reef ecosystems have continued to maintain high nutrient productivity under low nutrient conditions since the Darwin era; however, there is no reasonable explanation for this yet. The photosynthesis of symbiotic algae requires biogenic factors such as C, N and P, and the survival strategies of most reef symbioses in oligotrophic waters are still unknown. Increasing research is focusing on the efficient nutrient cycling between symbiotic algae and symbionts such as Tridacnidae and corals. The symbiont absorbs the metabolic waste from the host directly or absorbs dissolved organic matter from the surrounding seawater, but the importance of the absorption of dissolved organic matter for the symbiont is not well understood. P is often known as the ultimate limiting macronutrient in marine ecosystems, because unlike N, which has a biogenic source through N2 fixation, dissolved inorganic phosphorus (DIP) recycling from the remineralization of sinking organic matter and rock weathering is rather slow (Karl 2014).
Phytoplankton can respond to the limitation of inorganic P by utilizing dissolved organic P (DOP) in the aquatic ecosystem. DOP becomes an important P source for marine phytoplankton in parts of the sea where DIP is scarce. The symbiotic algae that maintain the health of the coral reef need to continuously absorb DIP from the surrounding water for photosynthesis. DOP in coral reef areas is mainly derived from the metabolites released by living organisms. Phosphate ions, phosphoesters and phosphonates are found in tissues of the scleractinian coral Stylophora pistillata (Godinot et al. 2016). In corals, phospholipids account for 25–40% of total lipids (Crossland, Barnes and Borowitzka 1980; Imbs 2013). Moreover, coral host tissues and symbiotic algae contain several types of phosphonates (Steen 1986; Godinot et al. 2016). They represent the major forms of phosphorus in marine organisms, especially dinoflagellates, accounting for 10–50% of cellular P (Steen 1986). Other P-containing compounds such as monophosphates and polyphosphates [adenosine-5′-triphosphate (ATP), ADP, adenylate and reductants] are found in corals and symbionts (Steen 1986; Jackson, Miller and Yellowlees 1989; Rands et al. 1992). The dinoflagellate Prorocentrum donghaiense can directly use DOP such as ATP effectively (Li et al. 2015). Such utilization might occur via enzymes such as alkaline phosphatase (AP), which has commonly been used as an indicator of DIP depletion and DOP utilization in phytoplankton in the past decades (Azam and Hondson 1977; Riemann 1979; Luo et al. 2017). Previous studies have indicated that glucose-6-phsphate (G-6-P) can support the growth and yield of Karenia mikinotoi as efficiently as DOP (Zhang et al. 2017), the growth of Karenia mikinotoi may be caused by both extracellular DOP hydrolysis and uptake of DOP or its hydrolysis products occur (Luo et al. 2017). If so, can symbiotic zooxanthellae absorb DOP directly or use it after extracellular hydrolysis? Furthermore, what effect does the concentration of DIP in the surroundings have on the cell growth rate and photosynthetic physiology of zooxanthellae? To address these questions, we chose two classes of DOP, those containing stable C-P bonds and those with C-O-P bonds and compared them with DIP and P deficiency groups to investigate the method of DOP utilization in Symbiodinium and explain the mechanism whereby coral reefs maintain high nutrient productivity in oligotrophic seawaters.
Phosphoesters (C-O-P bond) and phosphonates (C-P bond) are the two dominant classes of DOP in the ocean and contribute roughly 75 and 25% of the high-molecular-weight DOP reservoir in the ocean respectively (Kolowith, Ingall and Benner 2001; Young and Ingall 2010). ATP, an important form of DOP, occurs stably in seawater in significant concentrations (Azam and Hondson 1977). ATP utilization as a P source has rarely been investigated (Li et al. 2015) and the utilization mechanism remains unclear. The biochemical composition of DOP in the oceans is hydrolyzed by a diverse group of APs (Luo et al. 2011). There are three different types of APs (PhoX, PhoD and PhoA), which operate in heterotrophic bacteria and are often found in different cell compartments (cytoplasm, periplasm, outer membrane and extra cellular space) of marine prokaryotes (such as heterotrophic bacteria, synechococcus, photosynthetic protozoa etc.) (Dyhrman et al. 2006; Sebastian and Ammerman 2009; Kathuria and Martiny 2011; Luo et al. 2017). The PhnX-based phosphonatase pathway in bacteria is known to specifically catalyze the cleavage of the 2-AEP in Nature (Cui et al. 2016). Algal species can contain multiple AP genes coding for different proteins. For example, at least four different putative APs have been identified in the diatom Thalassiosira pseudonana, and all four were up-regulated under P-deficiency (Dyhrman et al. 2012). Some findings indicate that PhoA was abundant and played a significant role in a simulated eutrophic system to hydrolyze phosphate esters (Sebastian and Ammerman 2009). PhoX encodes an alkaline phosphatase and a member of the Pho regulon (von Krüger et al. 2006), but shares no homology with PhoA. To date, only one sequence of AP from Symbiodinium sp. CCMA192 has been published in NCBI. AP, PhnC and PhnX were reported as important functional genes in the DOP pathway of Symbiodinium kawagutii (Lin et al. 2015). PhnX which is related to phosphonate metabolism was detected from available transcriptomes in Symbiodinium (Cui et al. 2016).
It has remained unexplored whether Symbiodiniumare able to utilize DOP as sources of P. To address the gap of research, we chose to study ATP and G-6-P as C-P bonds DOP utilization in the Symbiodinium. Despite the toxic effects on weeds, glyphosate can be utilized by microbial communities as an alternative source of C, N or P (Krzysko-Lupicka and Sudol 2008), which are essential to all living organisms. As many studies have indicated that some bacteria, actinomycetes, fungi and unidentified microbes can degrade glyphosate (Forlani et al. 2008; Duke 2011), the effect of glyphosate on Symbiodinium should be determined. For the remaining 25% of DOP, phosphonates, to which 2-AEP and glyphosate belong, the potential to be utilized as a P-source by phytoplankton is investigated.
The utilization mechanisms underlying the physiological responses to different P conditions in Symbiodinium and other dinoflagellates are still poorly studied. We attempt to obtain some insights into whether putative AP, PhoA, PhoX and PhnX of Symbiodinium are functional in utilizing phosphoesters or phosphonates in the growth medium.
In this study, we investigated the effects of DOP on Symbiodinium, one important dinoflagellate, to assess whether DOP can support the growth of Symbiodinium, and then examined the expression patterns of four DOP-related genes (AP, PhoA, PhoX, PhnX; full sequences in Table S1, see online supplementary material) under different P conditions. We also explore whether these genes operate a similar mechanism to that of marine prokaryotes and algae in S. voratum, and illustrate the bioavailability of DOP to Symbiodinium and molecular responses to different P conditions.
MATERIALS AND METHODS
Algal culture and phosphorus treatments
The experimental zooxanthella Symbiodinium voratum (clade E) was provided by the Key Laboratory of Tropical Marine Bio-resources and Ecology, South China Sea Institute of Oceanology, Chinese Academy of Sciences, where 28S rRNA gene amplicon sequencing has been carried out in 2017. Zooxanthellae were cultured at 23°C ± 1 °C under a 12 h/12 h light/dark cycle with a photon flux of 100 µmol m−2 s−1 in batch cultures on autoclaved (120 °C, 20 min) f/2 medium (Guillard and Ryther 1962). The medium was prepared using filtered (0.22 µm) artificial seawater, in which DIP was below the detection limit (0.01 µM) of the phosphomolybdenum blue spectrophotometric method (Butler 1984). To prevent the carryover of P stored in zooxanthellae cells to the DIP-depleted experiment, the culture was acclimated in modified L1 medium with a P concentration of 10 µM until the P concentration reduced to about 1 µM by consumption when the experiment started. Although the culture flasks and media were sterilized by autoclaving, the DOP reagent will be decomposed under high temperature and pressure, so antibiotics were used in the axenic groups to eliminate bacterial contamination during batch culture; the non-axenic groups are without antibiotics. Bacterial abundance was checked at regular intervals using light microscopy and samples were plated on marine agar plates. Experimental cultures with six varying P conditions were set up in L1 medium (without silicate) as follows. For the DIP-replete treatment (+P), NaH2PO4 was added at a final concentration of 36 µM. The P-deprived treatment (−P) was set up without adding P. In the DOP treatment, DIP in L1 medium was replaced with ATP, G-6-P, glyphosate or 2-AEP at the same molar concentration (36 µM); each treatment was performed in triplicate. In the axenic experiments, KAS antibiotics (1X, final concentrations: 100 µg L−1 ampicillin, 50 µg L−1 kanamycin and 50 µg L−1 streptomycin) were added. The axenic condition was confirmed by 4’6-diamidino-2-phenylindole (DAPI) DNA staining when epifluorescence microscopy and samples plated on marine agar plates showed no bacteria.
Measurement of cell concentration, cell size, DIP concentration and population growth rate
During incubation, all flasks were shaken thrice a day to prevent cells from adhering to flask walls. All experiments were conducted in batches. The culture flasks and media were sterilized by autoclaving at 121°C and 150 kPa for 30 min before use to prevent bacterial contamination. All solutions were prepared using ultrapure water (resistivity >18 MΩ cm) from a Milli-Q system (EMD Millipore, Billerica, MA, USA) and chemicals of analytical grade (Sigma-Aldrich, St. Louis, MO, USA).
The initial culture volume was 500 mL, and 20mL samples were collected from each culture daily for monitoring population growth, cell size and DIP concentration in the cultures. Cell concentration was determined microscopically using a Sedgewick Rafter counting chamber (Lin et al. 2011). The average cell size was determined as the equivalent spherical diameter using a FlowCAM® 8000 series imager (Fluid Imaging Technologies, Yarmouth, ME USA) equipped with a high-resolution camera (1920 × 1200 pixels, magnification of 10X), with a particle size range of 5–20 µm. In addition, cell morphology was observed under fluorescence microscope (Zeiss Axio Scope A1, Carl Zeiss, Gottingen, Germany, 100X). For DIP measurement, 10 mL samples from each culture were filtered through a 0.22 µm mixed cellulose ester membrane. DIP concentrations of the filtrates were measured following the phosphomolybdenum blue spectrophotometric method (Butler 1984).
After collection, algal cells were fixed in a 2% formaldehyde solution and counted in a blood cell counting chamber using a light microscope (Zeiss, Germany) at 400X magnification. Each sample was counted thrice, with a maximum deviation of approximately 10%. The growth rate of algae was calculated as follows: µ = ln(N2/N1)/(t2− t1), where N1 and N2 are the cell densities on days t1 and t2, respectively. The maximum µ during the incubation period was defined as µmax.
Total daily release of DIP in DOP cultures estimated from changes in DIP in +P cultures and ATP and G-6-P cultures.
Time . | DIP consumption in P culture (µM) . | DIP consumption in ATP culture (µM)a . | Increment of DIP in ATP culture (µM)b . | Total released DIP in ATP culture (µM)c . | DIP consumption in G-6-P culture (µM) . | Increment of DIP in G-6-P culture (µM)b . | Total released DIP in G-6-P culture (µM)c . |
---|---|---|---|---|---|---|---|
Day1–Day2 | 5.2047 | 2.959 | >2.9591 | 1.964 | >1.9649 | ||
Day2–Day3 | 2.690 | 3.568 | 11.637 | 15.205 | 3.579 | 10.760 | 14.339 |
Day3–Day4 | 1.286 | 1.374 | 4.444 | 5.819 | 1.417 | 14.561 | 15.978 |
Day4–Day5 | 1.286 | 1.259 | 11.695 | 12.955 | 0.994 | 1.111 | 2.108 |
Day5–Day6 | 6.666 | 6.042 | 31.052 | 37.095 | 5.13 | 4.619 | 9.749 |
Day6–Day7 | 1.461 | 1.318 | 16.374 | 17.692 | 1.55 | 2.690 | 4.245 |
Total | >78.16 | >35.7 |
Time . | DIP consumption in P culture (µM) . | DIP consumption in ATP culture (µM)a . | Increment of DIP in ATP culture (µM)b . | Total released DIP in ATP culture (µM)c . | DIP consumption in G-6-P culture (µM) . | Increment of DIP in G-6-P culture (µM)b . | Total released DIP in G-6-P culture (µM)c . |
---|---|---|---|---|---|---|---|
Day1–Day2 | 5.2047 | 2.959 | >2.9591 | 1.964 | >1.9649 | ||
Day2–Day3 | 2.690 | 3.568 | 11.637 | 15.205 | 3.579 | 10.760 | 14.339 |
Day3–Day4 | 1.286 | 1.374 | 4.444 | 5.819 | 1.417 | 14.561 | 15.978 |
Day4–Day5 | 1.286 | 1.259 | 11.695 | 12.955 | 0.994 | 1.111 | 2.108 |
Day5–Day6 | 6.666 | 6.042 | 31.052 | 37.095 | 5.13 | 4.619 | 9.749 |
Day6–Day7 | 1.461 | 1.318 | 16.374 | 17.692 | 1.55 | 2.690 | 4.245 |
Total | >78.16 | >35.7 |
Daily consumption of DIP from ATP or G-6-P in the ATP or G-6-P cultures calculated from consumption in the P cultures following formula 1 shown in the text.
Daily increment (i.e., release) of DIP in ATP or G-6-P culture.
Calculated as a+b.
Total daily release of DIP in DOP cultures estimated from changes in DIP in +P cultures and ATP and G-6-P cultures.
Time . | DIP consumption in P culture (µM) . | DIP consumption in ATP culture (µM)a . | Increment of DIP in ATP culture (µM)b . | Total released DIP in ATP culture (µM)c . | DIP consumption in G-6-P culture (µM) . | Increment of DIP in G-6-P culture (µM)b . | Total released DIP in G-6-P culture (µM)c . |
---|---|---|---|---|---|---|---|
Day1–Day2 | 5.2047 | 2.959 | >2.9591 | 1.964 | >1.9649 | ||
Day2–Day3 | 2.690 | 3.568 | 11.637 | 15.205 | 3.579 | 10.760 | 14.339 |
Day3–Day4 | 1.286 | 1.374 | 4.444 | 5.819 | 1.417 | 14.561 | 15.978 |
Day4–Day5 | 1.286 | 1.259 | 11.695 | 12.955 | 0.994 | 1.111 | 2.108 |
Day5–Day6 | 6.666 | 6.042 | 31.052 | 37.095 | 5.13 | 4.619 | 9.749 |
Day6–Day7 | 1.461 | 1.318 | 16.374 | 17.692 | 1.55 | 2.690 | 4.245 |
Total | >78.16 | >35.7 |
Time . | DIP consumption in P culture (µM) . | DIP consumption in ATP culture (µM)a . | Increment of DIP in ATP culture (µM)b . | Total released DIP in ATP culture (µM)c . | DIP consumption in G-6-P culture (µM) . | Increment of DIP in G-6-P culture (µM)b . | Total released DIP in G-6-P culture (µM)c . |
---|---|---|---|---|---|---|---|
Day1–Day2 | 5.2047 | 2.959 | >2.9591 | 1.964 | >1.9649 | ||
Day2–Day3 | 2.690 | 3.568 | 11.637 | 15.205 | 3.579 | 10.760 | 14.339 |
Day3–Day4 | 1.286 | 1.374 | 4.444 | 5.819 | 1.417 | 14.561 | 15.978 |
Day4–Day5 | 1.286 | 1.259 | 11.695 | 12.955 | 0.994 | 1.111 | 2.108 |
Day5–Day6 | 6.666 | 6.042 | 31.052 | 37.095 | 5.13 | 4.619 | 9.749 |
Day6–Day7 | 1.461 | 1.318 | 16.374 | 17.692 | 1.55 | 2.690 | 4.245 |
Total | >78.16 | >35.7 |
Daily consumption of DIP from ATP or G-6-P in the ATP or G-6-P cultures calculated from consumption in the P cultures following formula 1 shown in the text.
Daily increment (i.e., release) of DIP in ATP or G-6-P culture.
Calculated as a+b.
Determination of alkaline phosphatase activity
The cellular alkaline phosphatase activity (APA) of zooxanthellae cells was measured daily at the same time during incubation. The APA was measured using 4-methylumbelliferyl phosphate (MUP) as the substrate (Hoppe HG 2003; Jacobson Meyers, Sylvan and Edwards 2014). MUP with a final concentration of 25 µM was added to each sample (2 mL) in the dark and incubated at 37°C for 2 h. At the end of the incubation, HgCl2 with a final concentration of 16 mM was added to stop the enzyme reaction. Fluorescence measurements were performed using a PerkinElmer LS-45 spectrofluorometer, with the excitation wavelength set at 365 nm and emission wavelength at 445 nm. The concentration of 4-methylumbelliferone (MUF) was calculated from a standard curve using MUF at concentrations from 0 to 10 µM. Potential APA rates (Vmax) were determined from Michaelis–Menten kinetics by using GraphPad Prism 5 (GraphPad Software) (Zhang et al. 2018).
Examination of bacterial involvement in different kinds of DOP utilization
In the second round of the experiment, the cultures were not axenic (without antibiotics). The other conditions were the same as those in the first round of the experiments, and the initial algal concentration was also the same. The growth of zooxanthellae in different DOP cultures was observed, and the concentration of DIP in the medium was measured. To further confirm that the utilization of 2-AEP was contributed by bacteria, we chose ATP, G-6-P and 2-AEP groups to perform a new experiment. Media from each of the treatments were gently filtered through autoclaved 3 µm polycarbonate membranes (vacuum, <10 psi) to collect the filtrate, which contained the bacteria from the culture and but excluded the algae. The filtrates containing the bacteria were added into the new cultures containing ATP, G-6-P or 2-AEP. Simultaneously, the three antibiotic-treated cultures without algae were used as controls.
RNA isolation and cDNA cloning of selected genes from zooxanthella
We performed RNA sequencing at Shanghai Majorbio Bio-pharm Biotechnology Co., Ltd. (Shanghai, China). Four selected genes related to DOP metabolism were detected from available transcriptomes data (full sequences in Table S1, see online supplementary material). To determine the response to different P conditions based on the selected genes, cultures were set up in triplicate and samples were collected in the exponential growth stage (day 4). Algal cells were harvested by centrifugation at 5450 × g for 15 min, and then cell pellets were resuspended in 1 mL of f/2 culture media, transferred to 2 mL screw cap tubes and centrifuged for an additional 10 min at 10 000 × g. The resulting pellet was snap-frozen in liquid nitrogen and stored at −80°C prior to RNA extraction. Total RNA isolation was performed using the RNeasy Mini Kit (Qiagen, Australia) combined with the Trizol method (Invitrogen, Australia), according to the manufacturer's instructions. The RNA concentration and quality were determined using NanoDrop spectrophotometer (Thermo, Germany) (Rosic and Hoegh-Guldberg 2010).
Fist-strand cDNA was synthesized from 1 μg of total RNA using the PrimeScriptTMRT reagent Kit (TaKaRa, Tokyo, Japan). To eliminate possible residual genomic DNA, the RNA sample was incubated with 2 μL 5 × gDNA Eraser Buffer, 1 uL gDNA Eraser at 42°C for 2 min. Next 1 μL of PrimerScript Buffer 2 was added. Reverse transcription reactions were incubated at 37°C for 45 min, then at 85°C for 5 s to deactivate the reverse transcriptase. All cDNA samples were stored at –20°C for subsequent experiments.
RT-qPCR-based expression analysis of selected genes
To identify the relative expression of selected genes, cDNA samples were analysed by Reverse transcriptase quantitative polymerase chain reaction (RT-qPCR). Specific primers for RT-qPCR (Table 2) were designed using Primer Premier 5.0 software to explore the differential expression of AP, PhoA, PhnX and PhoX under different P conditions. These primers were located in the conserved region shared by all the cDNA types obtained to ensure the amplification of all forms. Symbiodinium sp. type E2 was used as the reference (NCBI); this was tested in this study and showed a relatively stable level of expression. Keyword searches were also carried out to obtain all possible related sequences from transcriptome data. Sequences of PhnX, PhoA, PhoX, as well as AP from our Symbiodinium transcriptome data (Table S1), were used as queries to perform blastn against available Symbiodinium genomic and transcriptomic data sets, including genomes of S. kawagutii (Lin et al. 2015) (http://web.malab.cn/symka_new/index.jsp) and Symbiodinium sp. Clade B1 (http://marinegenomics.oits.jp/genomes/gallery/) and the transcriptomic datasets in CAMERA (http://camera.crbs.ucsd.edu/ddc/). All the sequences acquired from those datasets were further confirmed by performing blastn against the NCBI GenBank database.
Gene . | Directiona . | Sequence (5′-3′) . | Amplicon (bp) . |
---|---|---|---|
5.8s Symbiodinium E2 | F-primer | GGAGAAGCCTTGAGCCTCTT | 94 |
R-primer | GCGACAAATCAGTCCAGCTT | ||
Alkaline phosphatase (AP) | F-primer | GAAAGACCAAGCCTGCACTC | 176 |
R-primer | ACTCCACCACGTCCTTCAAC | ||
PhnX | F-primer | TGAGAAGAAGGAGGCCTTGA | 101 |
R-primer | GCTCCACCTTGAAGATCTCG | ||
PhoX | F-primer | TGCGGATCTATGTTCTGCTG | 84 |
R-primer | GATGCTTCAGCTCCATCTCC | ||
PhoA | F-primer | ATGGCAAGAAGGTGGTGTTC | 101 |
R-primer | GTTCATGCCATCCAGAACCT |
Gene . | Directiona . | Sequence (5′-3′) . | Amplicon (bp) . |
---|---|---|---|
5.8s Symbiodinium E2 | F-primer | GGAGAAGCCTTGAGCCTCTT | 94 |
R-primer | GCGACAAATCAGTCCAGCTT | ||
Alkaline phosphatase (AP) | F-primer | GAAAGACCAAGCCTGCACTC | 176 |
R-primer | ACTCCACCACGTCCTTCAAC | ||
PhnX | F-primer | TGAGAAGAAGGAGGCCTTGA | 101 |
R-primer | GCTCCACCTTGAAGATCTCG | ||
PhoX | F-primer | TGCGGATCTATGTTCTGCTG | 84 |
R-primer | GATGCTTCAGCTCCATCTCC | ||
PhoA | F-primer | ATGGCAAGAAGGTGGTGTTC | 101 |
R-primer | GTTCATGCCATCCAGAACCT |
F: forward primer; R:reverse primer
Gene . | Directiona . | Sequence (5′-3′) . | Amplicon (bp) . |
---|---|---|---|
5.8s Symbiodinium E2 | F-primer | GGAGAAGCCTTGAGCCTCTT | 94 |
R-primer | GCGACAAATCAGTCCAGCTT | ||
Alkaline phosphatase (AP) | F-primer | GAAAGACCAAGCCTGCACTC | 176 |
R-primer | ACTCCACCACGTCCTTCAAC | ||
PhnX | F-primer | TGAGAAGAAGGAGGCCTTGA | 101 |
R-primer | GCTCCACCTTGAAGATCTCG | ||
PhoX | F-primer | TGCGGATCTATGTTCTGCTG | 84 |
R-primer | GATGCTTCAGCTCCATCTCC | ||
PhoA | F-primer | ATGGCAAGAAGGTGGTGTTC | 101 |
R-primer | GTTCATGCCATCCAGAACCT |
Gene . | Directiona . | Sequence (5′-3′) . | Amplicon (bp) . |
---|---|---|---|
5.8s Symbiodinium E2 | F-primer | GGAGAAGCCTTGAGCCTCTT | 94 |
R-primer | GCGACAAATCAGTCCAGCTT | ||
Alkaline phosphatase (AP) | F-primer | GAAAGACCAAGCCTGCACTC | 176 |
R-primer | ACTCCACCACGTCCTTCAAC | ||
PhnX | F-primer | TGAGAAGAAGGAGGCCTTGA | 101 |
R-primer | GCTCCACCTTGAAGATCTCG | ||
PhoX | F-primer | TGCGGATCTATGTTCTGCTG | 84 |
R-primer | GATGCTTCAGCTCCATCTCC | ||
PhoA | F-primer | ATGGCAAGAAGGTGGTGTTC | 101 |
R-primer | GTTCATGCCATCCAGAACCT |
F: forward primer; R:reverse primer
RT-qPCR was performed using a Bio-Rad iQ SYBR Green Supermix kit (Bio-Rad Laboratories, Hercules, CA, USA) following the manufacturer's instructions, with all reactions set up in triplicate for each gene. Non-template control reactions were performed in triplicate for each pair of primers, and three biological repeats were performed. PCR conditions were as follows: initial denaturation step at 95°C for 2 min, followed by 45 cycles of denaturation at 95°C for 5 s, then annealing at 60°C for 10 s. The PCR products were separated by electrophoresis on a 1.5% (w/v) agarose gel and compared to a molecular marker. The relative gene expression levels compared to those in an unstressed state were presented as relative quantification values, which were calculated by the 2−⊿⊿Ct method (Livak and Schmittgen 2001).
Data analysis
The experimental data were expressed as mean ± standard deviation (mean ± SD) (n = 3), and the index growth period data were used for statistical analysis. The statistical software used was PASW Statistics 18.0 (P < 0.05 indicated significance; P < 0.01 indicated extremely high significance). The model was fitted with the data analysis platform OriginPro 8.5, and the experimental results were plotted using Oringin 2019.
RESULTS
Culture growth curve and DIP concentration under different P treatments with antibiotics
Throughout the culture period, the growth trends and cell concentrations of zooxanthellae in the phosphoester (ATP and G-6-P) media were very similar to those in the +P group. Despite the same initial cell concentration (∼10 000 cells mL−1), growth in the different P treatments started to diverge on day 3 (Fig. 1). Phosphoester (ATP and G-6-P) treatment showed an increasing trend and a highly similar growth trend with the +P treatment group, which showed exponential growth after the third day. After 6-day cultivation, the cell concentrations reached 4.7 × 104 cells mL−1 (+P), 4.3 × 104 cells mL−1 (ATP group) and 4.2 × 104 cells mL−1 (G-6-P group), roughly 4.5-fold of the initial concentrations in the three cultures, which indicated that zooxanthellae could utilize ATP and G-6-P as efficiently as +P. In contrast, the growth of zooxanthellae in the phosphonate (glyphosate and 2-AEP)-treated groups was inhibited throughout the experiment, only showing growth in the first 2 days and then entering the stationary phase toward a plateau, with ∼13 000 cells mL−1 as the maximum biomass (Fig. 1). The cell growth trends of phosphonate treatments were similar to those of the –P group (Fig. 1). The growth rates of +P, ATP, G-6-P, –P, AEP and glyphosate treatment with antibiotics were 0.33 d−1, 0.37d−1, 0.38 d−1, −0.041 d−1, 0.32 d−1 and 0.04 d−1, respectively during the exponential growth period (days 3–6) (Fig. 9). The growth rates of ATP, +P, G-6-P, –P, AEP and glyphosate treatment without antibiotics were 0.33 d−1, 0.35 d−1, 0.34 d−1, −0.041 d−1, 0.033 d−1 0.04 d−1, respectively during the exponential growth (days 3–6) (Fig. 10).
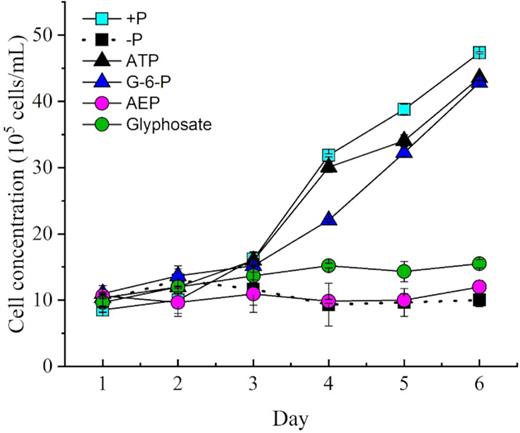
Growth curves of Symbiodinium (zooxanthellae) under different phosphorus conditions.
As shown in Fig. 2, the DIP concentration in the ATP and G-6-P treatments were undetectable at the beginning but increased sharply to 14.7 µM and 12.7 µM, respectively, on day 2, exceeding the concentration in the +P group (Fig. 2). On day 6, residual DIP values were about 78 µM and 35 µM in the ATP and G-6-P groups, respectively, despite consumption to support the observed growth, indicating a large amount of DIP released from ATP and G-6-P. In contrast, the DIP concentration of the +P group showed a decreasing trend. The initial DIP concentration was 36 µM in the +P group, and the DIP was consumed rapidly, decreasing to 18.8 µM on day 7. However, there were no significant differences in cell yield between the DOP (AEP and glyphosate) treatment group and the –P group, both of which showed marked growth depression compared to the +P group.
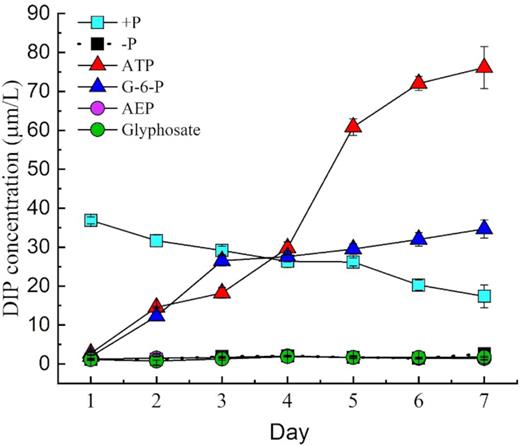
DIP concentrations in the medium of Symbiodinium (zooxanthellae) cultured in different P groups.
Cell size and Fv/Fm of different P sources under the axenic condition
As an essential physiological trait, cell size is related to cell growth and division (Prescott 1956), and it usually responds to nutrient limitation conditions (Turner et al. 2012). The cell size of zooxanthellae increased slightly in the phosphonate treatment groups after day 4 (Fig. 3), similar to the trends under –P conditions. However, cell size under the phosphoester treatments did not differ much from the original size, and the cell size was similar to that in the DIP group, although it was slightly bigger in the exponential growth phase (P < 0.05, PASW Statistics 18); therefore, it was also slightly smaller than the cell size in the –P group and phosphonate groups.
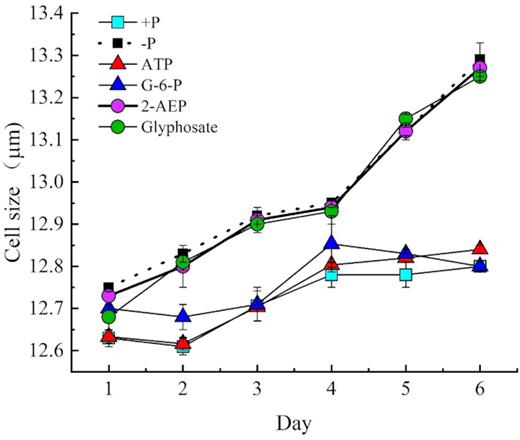
Cell sizes of zooxanthellae grown under different P conditions.
The optimal quantum yield of PSⅡ (Fv/Fm), which was monitored as a measure of photosynthetic health, was used as an indicator of physiological status. All six cultures started with an Fv/Fm of ∼0.52 ± 0.01, indicating that PSⅡ photochemistry was suitable in all the six cultures before the start of the experiment (Fig. 4). Throughout the experiment, the Fv/Fm of the phosphoester treatment groups remained stable, similar to the Fv/Fm in the +P group. However, in the phosphonate treatments, Fv/Fm decreased from day 1 to day 9, dropping to 0.196 and 0.199 in the 2-AEP and glyphosate groups, respectively (Fig. 4) (P < 0.05, paired-sample t-test). In the P-depleted (–P) treatment, Fv/Fm gradually declined from day 3 to day 9, dropping by 55.9% to 0.26 on day 9 (Fig. 4). In the +P treatment, cells maintained an Fv/Fm of ∼0.53 for the first 3 days, after which it increased to 0.56 on day 9. In the ATP and G-6-P groups, cells had an increasing Fv/Fm trend first, after which it dropped to 0.51 on day 9 in both groups (Fig. 4).
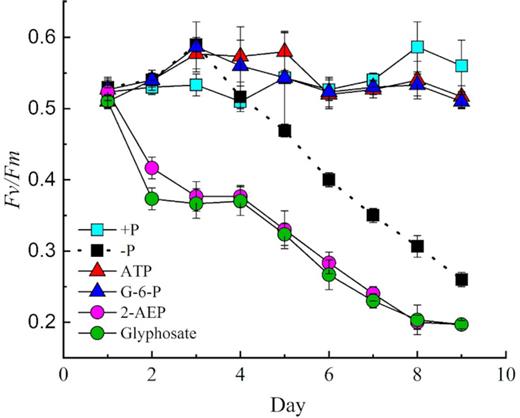
Optimal quantum yield of photosystem fluorescence (Fv/Fm) for zooxanthellae during a 9-day time course in DOP, DIP and P-depleted treatments (–P). Error bars represent the standard deviation of triplicate cultures.
Utilization of DOP
By measuring the residual DIP in the phosphoester medium, we found that more than 78.16 μM was provided from ATP in day 7(Table 1), indicating that more than one P group was obtained from each ATP molecule. Moreover, the final remaining DIP concentration in the G-6-P medium was > 35.7 μM (Table 1).
Our results showed that ATP and G-6-P were utilized to support the growth of zooxanthellae (bacteria were excluded by the antibiotic treatment) as efficiently as DIP. ATP and G-6-P were hydrolyzed because an increasing concentration of DIP was observed in the medium (Fig. 2). The release of DIP in the culture medium is consistent with the ectoenzymatic hydrolysis of ATP and G-6-P. The results showed that zooxanthellae can degrade ATP and G-6-P to phosphate (P-source), while no DIP was released into the medium in the phosphonate groups. Thus, zooxanthellae could not utilize glyphosate or 2-AEP as the sole P-source to support growth.
APA in the culture medium under different P treatment conditions
During the culture, APA was measured and was found to be similarly low in the phosphoester and +P cultures (Fig. 5), with no significant difference between them (P > 0.05, t-test). APA was barely detectable in the first 2 days in all five cultures (Fig. 5). On day 3 and thereafter, APA increased rapidly in the phosphonate groups. We found that APA in the –P group had a similar increasing trend. This indicated that AP was expressed and functioning in P-stressed cells (in the –P group) but not in the phosphoester groups or DIP-grown cells (in the +P group).
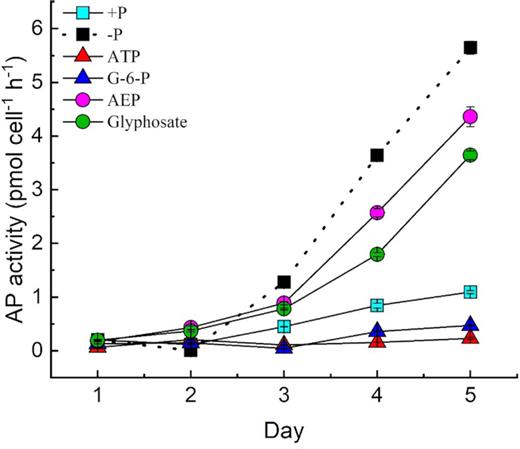
Utilization of phosphonate in zooxanthellae without added antibiotics
With the four DOP and two DIP treatments, we compared the growth in antibiotic-treated cultures with that in the non-antibiotic-treated cultures. Our results showed that all non-antibiotic-treated cultures, except phosphoesters (ATP and G-6-P), supported the growth of zooxanthellae. The cell concentration in 2-AEP cultures also displayed an increasing trend. However, cultures of the glyphosate treatment reached a relatively low maximum cell abundance of 15.5 × 104 cells mL−1 (Fig. 6). Similarly, –P treatment hardly showed any growth. Our results showed that the C-P bond of 2-AEP could support the growth of zooxanthellae in non-antibiotic-treated cultures (Fig. 6), whereas in the axenic cultures, hardly any growth occurred even with 2-AEP (Fig. 1). In addition, the DIP concentration of 2-AEP increased in the cultures without antibiotics (Fig. 7). Furthermore, the concentration of DIP in the culture group containing bacteria without algal cells showed an increasing trend (Fig. 8).
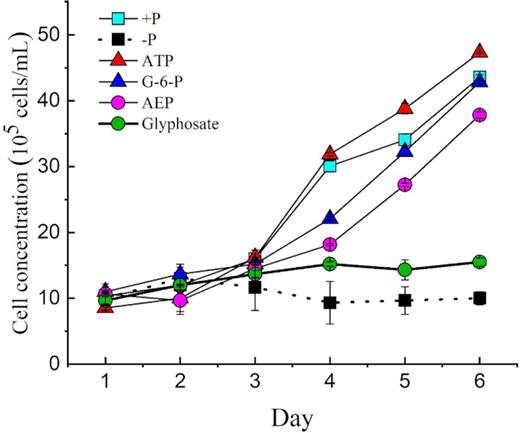
Growth curves of Symbiodinium (zooxanthellae) with different P sources without antibiotics.
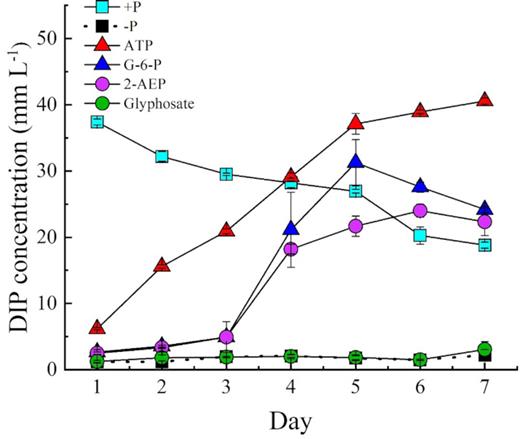
DIP concentrations in the media of Symbiodinium (zooxanthellae) in different P treatment groups without antibiotics.
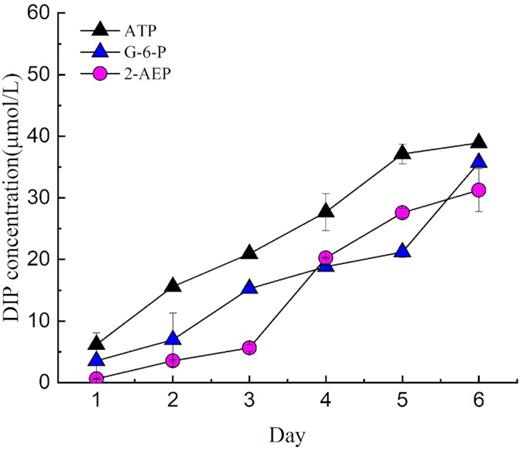
DIP concentration in the non-antibiotic cultures without algae but with bacterial filtrate.
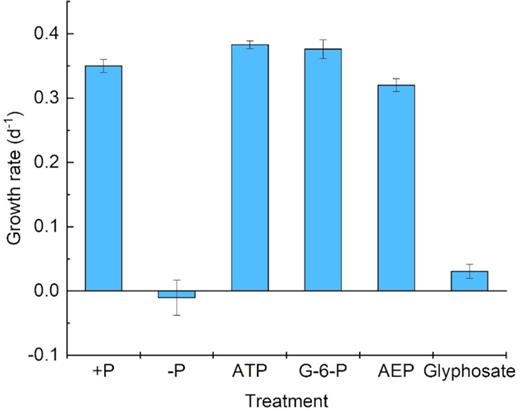
Growth rate of Symbiodinium (zooxanthellae) under different P conditions without antibiotics.
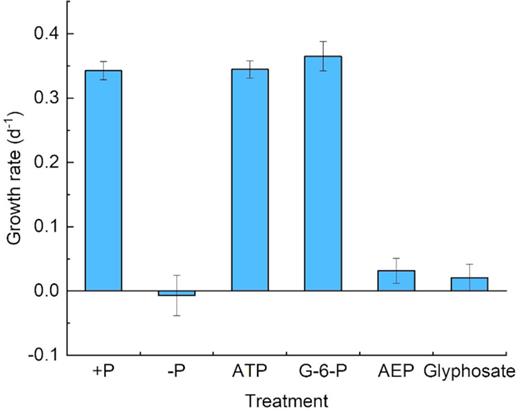
Growth rate of Symbiodinium (zooxanthellae) under different P conditions with antibiotics.
RT-qPCR analysis of the differential expression of selected genes based on different phosphorus treatments
RT-qPCR results showed that PhnX, PhoA and PhoX exhibited similar expression patterns under P conditions (Fig. 11). The expression levels of the other three genes were down-regulated while AP was up-regulated under –P conditions. Relative expressions of PhnX, PhoA and PhoX were up-regulated in phosphoester (ATP and G-6-P) groups but substantially down-regulated in phosphonate (2-AEP and glyphosate) groups. The relative expression of PhnX, PhoA and PhoX in +P culture is more than 8-fold of that in –P culture, similarly, PhnX, PhoA and PhoX expression in 2-AEP and glyphosate groups was down-regulated ∼4‒16-fold.

Relative expression of four selected genes analyzed by RT-qPCR. Shown are means ±standard deviations (error bars) from triplicate measurements from each treatment group.
A different pattern was found for AP gene: specifically, the relative expression was substantially up-regulated in phosphonates and −P cultures (Fig. 11) compared to that in +P culture. The relative expression of AP in the −P group was 3.8-fold higher than that in the +P group. Moreover, this trend remained when all the four genes were normalized to the reference gene.
DISCUSSION
Molecular insights into the utilization of DOP by Symbiodinium
ATP participates in the energy transfer for anabolic and catabolic cellular processes, and together with ADP and AMP, it controls the energy status of the cell (Karl and Craven 1980). Besides, ATP is also a source of organic P and has been reported to occur in a wide variety of aquatic habitats ranging from eutrophic freshwater lakes to temperate marine coastal regions and Antarctic seawaters (Azam and Hondson 1977; Riemann 1979). In prior studies, the uptake of ATP and other DOP compounds such as G-6-P has been shown to maintain the growth and other physiological activities in phytoplankton during DIP deficiency (Lin et al. 2012b; Flynn, Opik and Syrett 1986; Dyhrman and Palenik 1997; Xu et al. 2006). The dinoflagellate Karenia mikimotoi can also efficiently utilize ATP directly without showing evidence of DIP being released into the medium (Li et al. 2015; Luo et al. 2017; Zhang et al. 2017). It would be expected that the hydrolysis and subsequent transport of DOP-derived phosphates (or transport of DOP followed by intracellular hydrolysis) would cost energy and hence would only partially restore the growth of a phytoplankton population experiencing DIP deficiency (Zhang et al. 2014). In contrast, the aim of the present study was to determine whether symbiotic zooxanthellae absorb DOP directly or use it after extracellular hydrolysis. In general, the utilization of phosphorus esters facilitated by AP is believed to be the main factor for DOP utilization (Hoppe 2003). Therefore, DOP-based growth would be slower than DIP-based growth. Our results showed that this was not the case in zooxanthellae grown with ATP or G-6-P as the sole source of P. The growth curve of the ATP- and G-6-P-treated groups exhibited the same trend as those of the +P group, even with a slightly higher rate in the earlier stage of the experiment, until the last 2 days of the experiment (Fig. 1). While the ATP and G-6-P groups were was still growing in the exponential phase at the end of this 6-day experiment, with cell concentrations reaching >4 × 104 cells/mL, the growth of zooxanthellae was depressed in the −P, AEP and glyphosate groups, which reached the stationary phase on day 3, with a final cell concentration <1.5 × 104 cells/mL. The results indicated that the −P, AEP and glyphosate groups maintained stable cell concentrations, which means that the cells stopped dividing but did not die or that there was a balance between cell division and mortality. Perhaps certain substances secreted by the zooxanthellae decomposed phosphoesters (ATP and G-6-P), releasing a large amount of DIP into the medium (Fig. 2) instead of directly absorbing it. The increase in DIP observed in the medium suggests that ATP and G-6-P had been hydrolyzed before being absorbed by zooxanthellae. The concentration of DIP in the phosphoesters medium was finally observed to be high. The released DIP in the medium has been reported to be related to hydrolytic enzymes (Cui et al. 2016). However, in the present study, we found that zooxanthellae did not express or employ AP for ATP or G-6-P hydrolysis, indicating that other P hydrolases are necessary in DOP cycling by zooxanthellae. In contrast, APA increased sharply in the −P group and the phosphonate cultures, indicating that a P-limited state in the phosphonate group caused the zooxanthellae to secrete AP. Therefore, these contrasting results suggest that the mechanism underlying the utilization of ATP might differ in different species of dinoflagellates, as well as other groups of phytoplankton.
AP is known as a variable gene in marine phytoplankton with different forms and unique characteristics (e.g. different gene sequences, different requirements for metal in the active site and different substrates) compared to AP sequences that have been characterized in marine algae (Lin et al. 2012a). In the present study, expression of the AP gene was found to be up-regulated by more than 3.8-fold under −P conditions and more than 2-fold in 2-AEP and glyphosate groups, but not significant changed by ATP and G-6-P supplies. The results were consistent with our previous AP activity results. An increase in genomic data has provided evidence for the presence of different types of AP genes in eukaryotic phytoplankton (Dyhrman and Ruttenberg 2006); however, only some of these have ever been (partially) characterized through gene isolation and expression. Putative PhoX genes have been identified in green algae, Volvox carteri and Chamydomonas reinhardtii (Quisel, Wykoff and Grossman 1996). A study has clearly suggested that Symbiodinium has the ability to store P under phosphate enrichment (Godinot et al. 2011). Our transcriptomes and RT-qPCR results showed that PhoA, PhoX and PhnX perhaps responsible for transporting DIP from medium into cells and storage of DIP, the relative expression level of those genes may be positively correlated with the supplement of concentration of DIP from Fig. 2 and Fig. 11. Whether these AP genes are involved in the transport and storage of DIP, or the secretion of related enzymes that stimulate the decomposition of C-P bond DOP, remains to be further studied. Down-regulation in the −P and phosphonates groups may be due to the scarcity of P, leading to a decrease in the transport enzyme. In addition, the RT-qPCR result is consistent with the result of AP activity (Fig. 5). A previous study showed that PhnX is an intracellular enzyme, suggesting that the transport of phosphonates into the cell would be required for algae to utilize phosphonates from the external environment (Cui et al. 2016). From our with bacteria and without bacteria experiment results, phosphonate transporters in Symbiodinium must be largely divergent from those of other bacteria, such that they are currently unrecognizable. It is not surprising that Symbiodinium, and probably also other groups of phytoplankton, have the genetic potential to synthesize phosphonates. Moreover, phosphonates might also exist in phytoplankton cells as a form of P storage, which is produced during P abundance to sustain phytoplankton growth during periods of P-limitation (Ternan and Quinn 1998; Eixler, Karsten and Selig 2006). However, future research is required to uncover the interaction between bacteria and zooxanthellae.
Continued cell size growth under P deficiency
As an essential physiological trait, cell size is related to cell growth and division, and it usually responds to nutrient limitation conditions (Turner, Ewald and Skotheim 2012). Accordingly, nutrient limitation in phytoplankton usually leads to a reduction in cell size, as in the case of N (Sunda and Hardison 2007) and Fe (Sunda and Huntsman 1995). Contradictory to this, zooxanthellae cells were slightly larger under P-limiting conditions than under P-replete conditions. Similarly, the size of Alexandrium catenella cells has been found to be significantly larger under P-depleted than under P-replete conditions (Zhang et al. 2014).
On the basis of the algal growth curves, Fv/Fm, cell size and APA during the incubation period, we believe that the test species was under P-limiting conditions after day 4 of the P-depleted treatment.
Ecological significance of phosphonate utilization through bacteria in the marine environment
The results of the culture experiments showed that although zooxanthellae could not directly utilize the external phosphonate under aseptic conditions, the bacteria present in the culture effectively made the P in the 2-AEP available to zooxanthellae. However, glyphosate cannot be directly used by zooxanthellae, nor can it be decomposed into inorganic P by bacteria and then used by zooxanthellae. It is noteworthy that in the 2-AEP culture experiment, the DIP concentration continued to increase despite a high concentration of DIP in the medium. This indicates that 2-AEP catabolism is DIP-independent, consistent with earlier studies, showing that 2-AEP catabolism in some bacteria is not limited by the presence of DIP (Quinn et al. 2007). We noticed that the amount of DIP released from 2-AEP by the bacteria was quite high, enough to support the growth of zooxanthellae, indicating that these bacteria have a strong ability to degrade 2-AEP and release DIP into the environment.
Bacteria capable of metabolizing phosphonate are widely distributed in marine environments (Villarreal-Chiu 2012). Considering that phosphonate contributes 25% of high-molecular-weight DOP in the ocean, the bacterial use of phosphonate can provide a large amount of DIP for zooxanthellae in light-transmissive areas of P-limited waters (Kolowith, Ingall and Benner 2001). We found that phosphoesters can be decomposed by the extracellular products of zooxanthellae and can also be decomposed by bacteria to produce DIP, which can effectively support the growth of zooxanthellae. However, this was an in vitro experiment; therefore, further research is needed to understand whether these bacteria are associated with reef-building organisms, corals, algae or zooxanthellae in wild coral reef ecosystems, because many bacteria have been shown to be intimately linked to algae (Seyedsayamdost et al. 2011). This is relevant because some corals have strong antibacterial and immune systems.
CONCLUDING REMARKS
This study is the first attempt to compare the physiological variety analysis and differential expression gene in zooxanthellae under different P conditions. The ability to utilize DOP is important for zooxanthellae to survive in areas with scarce DIP. We found that in aspetic conditions, zooxanthellae efficiently utilized phosphoesters (ATP and G-6-P). However, phosphonates (2-AEP and glyphosate) could not be used directly by zooxanthellae to maintain their growth. In fact, phosphoesters supported equivalent growth rates and final growth yields within zooxanthellae. Next, we found that bacteria might be involved in the hydrolysis of 2-AEP. The types of bacteria responsible and their relationship with zooxanthellae remain to be explored. We also found that the lack of P, an essential element of algal growth, caused physiological changes in zooxanthellae, e.g. cell growth rapidly decreased, while cell size increased slightly, in addition Fv/Fm of cells decreased. Our research fills the knowledge gap regarding the use of DOP by zooxanthellae. Probably as an adaptation to DIP deficiency (e.g. as result of N overloading in coastal waters) and the relatively high availability of DOP (e.g. after a diatom bloom), zooxanthellae cultured in the laboratory can utilize different types of DOP efficiently. Results of differential gene expression will allow us to interrogate the process and molecular mechanism regulating phosphoester uptake by Symbiodinium and shed light on potential related genes for phosphoester utilization. Furthermore, our results indicate that AP activity is an indicator of P stress, but not an exclusive indicator of DOP utilization. Extracellular phosphonates cannot be utilized by Symbiodinium apparently owing to the absence of phosphonate transporters in the cell membrane. However, the exact function of phosphoesters and phosphonates, as well as DOP utilization, in Symbiodinium with symbionts in the marine environment require further investigation. Importantly, our findings on the P utilization and metabolism of the symbiotic zooxanthellae provide a theoretical basis for assessing global changes in marine environments, protecting coral reefs and monitoring reef health.
ACKNOWLEDGEMENTS
We thank all members of South China Sea Institute of Oceanology, CAS, for their technical support and assistance. This work was financially supported by the National Basic Research Program of China (2015CB452903), Guangdong marine economy promotion projects Fund (GDOE[2019]A32), National Natural Science Foundation of China(41806198), Science and Technology Planning Project of Guangdong Province, China (2017B0303014052), and Innovation Academy of South China Sea Ecology and Environmental Engineering, Chinese Academy of Sciences (ISEE2018PY01).
Conflicts of interest. None declared.