-
PDF
- Split View
-
Views
-
Cite
Cite
Simone Dealtry, Eman H. Nour, Peter N. Holmsgaard, Guo-Chun Ding, Viola Weichelt, Vincent Dunon, Holger Heuer, Lars H. Hansen, Søren J. Sørensen, Dirk Springael, Kornelia Smalla, Exploring the complex response to linuron of bacterial communities from biopurification systems by means of cultivation-independent methods, FEMS Microbiology Ecology, Volume 92, Issue 2, February 2016, fiv157, https://doi.org/10.1093/femsec/fiv157
- Share Icon Share
On-farm biopurification systems (BPSs) treat pesticide-contaminated wastewater at farms through biodegradation and sorption processes. However, information on the microbiota involved in pesticide removal in BPSs is scarce. Here we report on the response of BPS bacterial communities to the herbicide linuron (BPS+) compared with the control (BPS−) in a microcosm experiment. Both denaturing gradient gel electrophoresis (DGGE) and pyrosequencing of 16S rRNA gene fragments amplified from community DNA indicated shifts in the bacterial community after linuron application. Responding populations belonged to taxa that were previously reported from linuron degrading consortia cultivated from soil (Hyphomicrobiaceae, Comamonadaceae, Micrococcaceae). In addition, numerous taxa with increased relative abundance were identified that were previously not associated with linuron degradation. The relative abundance of IncP-1 korB copies increased in response to linuron application. Amplicon pyrosequencing of IncP-1 trfA genes revealed a high IncP-1 plasmid diversity and suggested that populations carrying IncP-1β plasmids increased in relative abundance. Transferable mercury resistance plasmids were exogenously captured from BPS+/BPS−, and in three transconjugants from BPS+ the gene hylA was detected. Our data suggest the existence of a multispecies linuron degrading bacterial food web and an involvement of IncP-1 plasmids in the adaptation of bacterial communities to pesticide pollution in BPSs.
INTRODUCTION
On-farm biopurification systems (BPSs) are increasingly used for the local treatment of pesticide-contaminated wastewater at farms. In BPSs, the contaminated water is percolated over a solid matrix, the so-called biomix, which is a mixture of local soil, chopped straw and peat, or composted material in which the pesticides are removed by biodegradation and sorption processes. BPSs are considered a simple, low cost, practical approach for farmers to treat pesticide-contaminated water on farms (Castillo, Torstensson and Stenstrom 2008). Understanding the microbiology and the actual biodegradation processes in BPSs is of importance for further acceptance and implementation of the technology. Moreover, since the microbial communities in BPSs are regularly fed with pesticide-contaminated water imposing a continuous selection pressure for pesticide utilizing bacteria, BPSs provide an ideal model system to study mechanisms of adaptation of bacterial communities to pesticide pollution including the genetic adaptation towards the acquisition of novel biodegradation pathways. Agricultural soil regularly exposed to a particular pesticide often shows accelerated degradation of that compound, which is explained by the proliferation of adapted bacteria that carry biodegradation pathways (Arbeli and Fuentes 2007). Mobile genetic elements (MGEs), collectively referred to as the mobilome, were shown in many studies to play an important role in the bacterial adaptation to pesticide biodegradation within a microbial community by promoting the assembly and spread of pesticide catabolic gene clusters within the community (Top and Springael 2003; Dennis 2005; Heuer and Smalla 2012; Król et al. 2012). Among the known catabolic plasmids, IncP-1 plasmids are of particular importance as they have a broad host range and transfer efficiently in soil-like environments (Pukall, Tschäpe and Smalla 1996; Götz and Smalla 1997; Musovic et al., 2006, 2010; Mølbak, Molin and Kroer 2007; Heuer and Smalla 2012). IncP-1 plasmids have been often associated with catabolism of pesticides such as 2,4-dichlorophenoxyacetic acid (Top, Holben and Forney 1995; Trefault et al. 2004; Vedler, Vahter and Heinaru 2004), atrazine (Martinez et al. 2001) and chloroaniline (Król et al. 2012). Although biodegradation is assumed to be an important process for pesticide removal in BPSs, in contrast to agricultural soil, information about the microbiota involved and/or the microbial community response to pesticide application in a BPS environment is scarce. Some studies have examined the biodegradation of the phenylurea herbicide linuron in artificially composed BPS environments in controlled microcosm systems, but they have mainly focused on the role of pesticide-primed soil, i.e. soil with a history of pesticide exposure, as a material to bioaugment BPS materials with linuron degrading bacteria present in that soil. Dunon et al. (2013) showed an increase in the abundance of MGEs such as IS1071 and IncP-1 plasmids, accompanied by an increase in mineralization of various pesticides, indicating that bacteria hosting those elements might carry genes involved in the degradation of those pesticides (Dunon et al. 2013). Dunon et al. (2013) and Dealtry et al. (2014a,b) also showed that BPSs in operation naturally show quite a high abundance of IncP-1 plasmids, suggesting that this occurs in BPS materials in the field, where these elements play a role. However, it was not studied how bacterial communities present in a long-term treated BPS matrix and the IncP-1 plasmid diversity respond in detail to linuron addition in comparison with an untreated control.
In the present study, we used 454 pyrosequencing of the bacterial 16S rRNA gene fragments and of the IncP-1 trfA gene amplified from total community (TC-) DNA to study the response of the bacterial communities and their IncP-1 mobilome to linuron in a ‘true’ BPS environment and to quantify IncP-1 plasmid abundance by korB real-time PCR over time. The BPS material used was obtained from a BPS that has been in operation for several years at a farmyard in Kortrijk (Belgium), and hence the microbial communities were exposed to a wide range of pesticides including linuron for several years. We hypothesized (i) that the exposure of the BPS bacterial communities to high concentrations of linuron will, in comparison with untreated controls, cause changes in the relative abundance of specific bacterial populations, and (ii) that the relative abundance of IncP-1 plasmids potentially carrying catabolic genes might increase due to the proliferation of bacteria with IncP-1 plasmids or their spread through horizontal gene transfer. In particular, as IncP-1 plasmids belonging to different subgroups were recently shown to occur in BPS material from different sites and to increase over a field season (Jechalke et al. 2013; Dealtry et al. 2014a,b), we were interested to follow the changes in the relative abundance of the IncP-1 subgroups in BPS material without linuron (control: BPS−) and with linuron addition (BPS+). These TC-DNA based analyses were accompanied by attempts to exogenously capture plasmids from BPS− and BPS+ material to test whether they carry known genes involved in linuron degradation, such as libA (Bers et al. 2011b) and hylA (Bers et al. 2013) coding for the enzymes initiating the hydrolysis of linuron to 3,4-dichloroaniline and N,O-dimethylhydroxylamine. Our study provides the first detailed description of the bacterial community composition in a ‘true’ BPS environment as well as of the IncP-1 mobilome in response to linuron addition compared with an untreated control under well-controlled conditions.
MATERIALS AND METHODS
Linuron
Linuron [3-(3,4-dichlorophenyl)-1-methoxy-1-methyl urea] (purity, 99.5%) was purchased from Sigma-Aldrich (Belgium).
Biopurification system and sampling
The BPS in Kortrijk, Belgium, a Phytobac system (20 m × 1.2 m), was taken into operation in 2008 and treats around 15 m3 of pesticide-contaminated water per annum. In a closed circuit, the pesticide-contaminated water is run over a biomix where pesticides are removed by sorption and microbial degradation while water is partially removed by evaporation. The percolate is drained at the bottom of the BPS system, collected in a tank and reused as influent. The biomix of the BPS consists of soil and manure (straw and animal feces). The influent water is contaminated with a broad variety of pesticides from spillage and residue water collected when cleaning the spraying equipment. No data are available on the actual amount and composition of the different pesticides in the influent water, which are mainly dependent on the good practices of the farmer. In total, 71 different pesticides, mainly herbicides including linuron, were used at the farm and were thus likely added to the BPS with contaminated water. An overview of the pesticides detectable in BPS material was provided previously by Dealtry et al. (2014a). Five kilograms of BPS samples were taken from the top material (to a depth of 10 cm) at the left, middle and right site section of the Phytobac, dried and sieved (2 mm). After sieving, the BPS material was stored at 4°C until use.
Microcosm set-up
Three replicates representing the three BPS sections were used in the experiment. The microcosms were 50 mL Erlenmeyer flasks that contained either BPS biomix material amended with linuron (BPS+ microcosms) or biomix material without the linuron spike (BPS− microcosms). Linuron-spiked BPS material was prepared as follows. Twenty-five milligrams of linuron (Sigma-Aldrich, Belgium) was dissolved in 5 mL of acetone in the Erlenmeyer flask and mixed with 1 g of BPS material. The linuron concentration used was higher than what is typically applied during on-farm operation of the BPS in order to clearly induce responses. After overnight evaporation of the acetone, an additional 4 g of BPS material was given to each replicate, resulting in a final linuron concentration of 5 mg g−1. BPS− microcosms were prepared similarly but 5 mL of acetone without linuron was first mixed with 1 g of BPS material. The six microcosms were incubated in the dark at approximately 20°C, and the percentage of water in each sample was determined by weighing the samples before and after drying at 110°C. In order to keep the moisture content constant, the samples were weekly weighed and water was added when necessary. Biomix samples were taken on days 1, 12 and 25 of the incubation. At each sampling time, the biomix material was mixed with a sterile spatula and 0.5 g material was taken for TC-DNA extraction.
DNA extraction from biomix samples
TC-DNA was extracted from 0.5 g biomix samples using the FastDNA® Spin Kit for Soil (Bio101, Qbiogene, Carlsbad, CA, USA) after a harsh lysis step with the FastPrep FP120 bead beating system for cell lysis. The DNA was purified by GENECLEAN Spin Kit (Qbiogene), and the yield and quality were checked by electrophoresis in 1% (w/v) agarose gels under UV light after staining with ethidium bromide. The extracted TC-DNA was used for PCR-Southern blot hybridization, qPCR, denaturing gradient gel electrophoresis (DGGE) and sequencing. The absence of PCR-inhibiting substances was checked by PCR amplification of 16S rRNA gene fragments from TC-DNA using the primers F27 and R1494 and the conditions as previously described (Heuer et al. 2009).
PCR-DGGE analysis
PCR-DGGE analysis of community bacterial 16S rRNA gene was performed as previously described by Gomes et al. (2005). The GelCompar 5.0 program was used to analyse the fingerprints. Clustering of patterns was calculated applying the unweighted pair group method with arithmetic mean (UPGMA) (Smalla et al. 2001).
Detection of 16S rRNA and IncP-1 gene copies by quantitative real-time PCR
Bacterial 16S rRNA gene copy numbers were quantified as described by Suzuki, Taylor and DeLong (2000), while korB copy numbers were quantified as described by Jechalke et al. (2013), with the recently discovered IncP-1ζ plasmids not being targeted. korB gene copy numbers were related to 16S rRNA gene copy numbers.
Pyrosequencing of bacterial 16S rRNA gene amplicons and IncP-1 trfA gene amplicons
Pyrosequencing of the 16S rRNA and IncP-1 trfA gene amplicons was performed on TC-DNA extracted from samples taken after a 25-day incubation. A 480 bp region of the bacterial 16S rRNA gene was amplified with primers 341F (5′-CCTAYGGGRBGCASCAG-3′) and 806R (5′-GGACTACNNGGGTATCTAAT-3′) (Hansen et al. 2012) flanking the V3–V4 regions. A 281 bp region of the IncP-1 trfA gene was amplified using the three primer sets described by Bahl et al. (2009). 16S rRNA gene amplification and attachment of adapters and barcode tags were performed as described previously (Masoud et al. 2011). The attachment of adapters and barcodes to the trfA amplicons was done using the U-linker system described by Holmsgaard, Sørensen and Hansen (2013). Thirty-five cycles were run in the initial PCR amplification, and the amplicon DNA was purified using the Montage Gel extraction kit (Millipore, Billerica, MA, USA). Sequencing was done at the Danish National High-Throughput DNA-Sequencing Centre, Copenhagen, on a GS FLX Titanium PicoTiterPlate using the GS FLX pyrosequencing system (Roche, Basel, Switzerland).
Analysis of bacterial 16S rRNA gene amplicon sequences
Pyrosequencing data from the 16S rRNA gene amplicons were trimmed, quality-filtered and denoised as explained for the trfA sequences. Chimeric reads were removed with uChime in uSearch 5.2.32 (Edgar et al. 2011) against the GOLD database (Pagani et al. 2012). The analysis of sequences was done according to Ding, Heuer and Smalla (2012). Briefly, sequences were used for BLASTN analysis against the SILVA database (Pruesse et al. 2007). In order to compare the bacterial community structure in BPS− and BPS+ on day 25, cluster analyses were performed based on the pair-wise Pearson correlation that is suitable for comparing samples with a different number of sequence reads. The reliability of clusters was tested by 500 times bootstrap analyses. Sequence clusters identified as belonging to the family Comamonadaceae were further analysed for their possible membership to the Variovorax phylotypes. A representative sequence from each cluster was used to calculate a neighbor-joining tree (1000 bootstrap replicates) together with the 16S rRNA gene sequences from 37 Variovorax and 13 Acidovorax strains. Sequences from the strains were trimmed to between primers 341F and 806R used for sequencing and aligned using Muscle in MEGA 5.0 (Tamura et al. 2011).
Analysis of trfA amplicon sequences
Primers, barcode tags and adapters were trimmed away and the sequences quality-filtered and denoised using AmpliconNoise (Quince et al. 2011). Sequences of less than 231 ± 3 bp in length were discarded (Holmsgaard, Sørensen and Hansen 2013). Chimeric sequences were removed de novo with uChime in uSearch 5.2.32 as described by Edgar et al. (2011). Briefly, sequences were dereplicated and chimeric sequences removed de novo based on abundance. These non-chimeric sequences were then used as reference database to remove chimeric sequences in the standard uChime database mode. The cleaned amplicon sequences were assigned to the seven IncP-1 subgroups by clustering them together with known IncP-1 trfA reference sequences (supplementary Table S1) at 89% nucleotide sequence similarity (Holmsgaard, Sørensen and Hansen 2013). Each cluster was given a number. Sequences from five large clusters, all containing reference sequences, were extracted and independently re-clustered at 97% nucleotide sequences similarity. Each new cluster was given a number appended to that of the cluster the sequences were extracted from. To calculate neighbor-joining trees, a representative sequence from each of the original clusters (89% similarity) or each of the new clusters (97% similarity) that contained ≥1% of the sequences from the original cluster were used. Sequences were translated to amino acids, aligned using Muscle (eight iterations), back-translated to nucleotides and used to build a neighbor-joining tree (1000 bootstrap replicates) in MEGA5.0 (Tamura et al. 2011). Significant differences (P < 0.05) in the relative number of sequences from BPS− and BPS+ were tested using Student's t-test at both clustering levels. Data were log-transformed and variance homogeneity verified with Bartlett's test. Denoising and clustering were done with the QIIME 1.5.0 software package (http://qiime.org/, Caporaso et al. 2010) using QIIME tools or QIIME wrappers of other tools. All other bioinformatics were done using Biopieces (www.biopieces.org, developed by Martin A. Hansen), and statistical analyses were performed in R 2.15.1 (R Core Team 2012).
Biparental exogenous isolation of plasmids
The rifampicin-resistant mutant, Pseudomonas putida KT2442 gfp, carrying the gfp gene coding for green fluorescence protein (GFP) (Heuer et al. 2002) was used as recipient strain for biparental exogenous capturing of plasmids either conferring growth on linuron or resistance to mercury. Potential transconjugants were selected on minimal salt medium (Dejonghe et al. 2003) supplemented with linuron (20 μg mL−1; Sigma-Aldrich) or HgCl2 (20 μg mL−1), kanamycin (50 μg mL−1) and rifampicin (50 μg mL−1; Serva Electrophoresis GmbH). The bacterial fraction from BPS− and BPS+ material was obtained through shaking 3 g of material taken from each microcosm after 25 days of incubation in 25 mL of 1/10 tryptic soy broth (TSB; Merck KGaA, Darmstadt, Germany) at room temperature for 2 h. After settling for 1 h, the supernatant was transferred to a Falcon tube and settled again for 15 min. The supernatant was transferred to a new Falcon tube and centrifuged for 10 min at 500 × g. The recipient was obtained after overnight growth of Pseudomonas putida KT2442 gfp under shaking in 10 mL Luria–Bertani (LB) broth containing kanamycin (50 μg mL−1) and rifampicin (50 μg mL−1) at 28°C. The cells were harvested by centrifugation for 10 min at 500 × g and resuspended in 1 mL TSB without antibiotics added. Five hundred microlitres of the cell pellet (corresponding to donor bacteria detached from 3 g of microcosm material) and 250 μL of resuspended recipient cells were mixed and centrifuged (5 min, 10 000 × g). After discarding the supernatant the cell pellet resuspended in 200 μL of 1/10 TSB was transferred to a filter placed on Plate Count Agar (PCA) as described by Heuer et al. (2002). After overnight growth at 28°C, the filters were resuspended in 10 mL of sterile saline solution to dislodge the cells. Transconjugants were obtained by plating serial 10-fold dilutions of the cells resuspended from the filters onto either a minimal salt medium (Dejonghe et al. 2003) containing linuron (20 μg mL−1), cycloheximide (100 μg mL−1; Serva Electrophoresis GmbH), kanamycin (50 μg mL−1) and rifampicin (50 μg mL−1), or on Mueller–Hinton (MH) agar plates (Merck KGaA, Darmstadt, Germany) containing HgCl2 (20 μg mL−1), cycloheximide (100 μg mL−1), kanamycin (50 μg mL−1) and rifampicin (50 μg mL−1). The plates containing linuron were incubated at 28°C and evaluated for the presence of transconjugants after 48 h up to 20 days of incubation. Plates containing HgCl2 were counted for colony forming units (CFUs) after 48 h of incubation at 28°C, and GFP-positive colonies were picked for further analysis. The recipient counts were determined on the same medium but supplemented with cycloheximide (100 μg mL−1), kanamycin (50 μg mL−1) and rifampicin (50 μg mL−1) only. The transfer frequencies are given as quotient of numbers of transconjugants and numbers of recipients.
Transconjugants were harvested from 5 mL overnight cultures in LB broth, and plasmid DNA was obtained by phenol–chloroform extraction of the potassium acetate fraction (Smalla et al. 2000). The quality of the PCR products was checked under UV light after agarose gel electrophoresis (1%, w/v) and after staining of the gel with ethidium bromide. Plasmid DNA from transconjugants was screened for the presence of IncP-1 (Bahl et al. 2009), IncP-7 (Izmalkova et al. 2005) and IncP-9 (Dealtry et al. 2014a) by PCR. Plasmid DNA was digested with Sma1 (Fermentas GmbH, St Leon Rot, Germany) and used for Southern blot hybridization with the different PCR-derived probes according to Dealtry et al. (2014a).
PCR–Southern blot-based screening detection of exogenously captured IncP-1 plasmids for the presence of libA and hylA genes
Plasmid DNA obtained from transconjugants of BPS+ was examined by PCR for the presence of genes involved in the degradation of linuron (libA and hylA). The primer sets and PCR conditions used were recently published by Bers et al. (2011b, 2013) (supplementary Table S2). In order to confirm the PCR results, plasmid DNA was digested with BstI/PstI enzymes (Fermentas GmbH) and Sma1 enzyme for detection of libA and hylA genes, respectively. The restriction fragments were separated by agarose gel electrophoresis (1%, w/v), Southern blotted and hybridized with digoxigenin-labeled libA and hylA probes. The probes were generated by labeling the PCR amplicon obtained from reference strains (supplementary Table S2) (Bers et al. 2011a,b, 2013) after gel purification with QIAEX II Gel® Extraction kit, with the Digoxigenin kit following the manufacturer's protocol (Roche Diagnostics, Mannheim, Germany).
Triparental mating
In order to demonstrate the localization of hylA on the IncP-1 plasmid, the ability of IncP-1 plasmids to mobilize IncQ plasmids was exploited. The triparental mating was performed as described by Smalla et al. (2006) except that C. necator JMP228r (Rifr Nalir) was used as a recipient, E. coli (pSM1890) (GFP, Smr Gmr) as donor of IncQ plasmid, and one hylA positive P. putida KT2442 (GFP, Rifr) transconjugant as a helper strain. Potential transconjugants were selected on plate count agar supplemented with gentamicin (20 μg mL−1), streptomycin (50 μg mL−1), rifampicin (50 μg mL−1) and nalidixic acid (200 μg mL−1). One potential transconjugant colony was picked, re-streaked, and freshly grown colonies were harvested and used for plasmid DNA extraction. PCR screening for hylA and trfA was done as previously described by Bers et al. (2013) and Dealtry et al. (2014a). Furthermore, Southern blot hybridizations of digested plasmid DNA with digoxigenin-labeled probes for hylA and trfA were performed.
Nucleotide sequence accession numbers
Amplicon sequences have been submitted to NCBI SRA as study SRA072553 with 16S rRNA gene amplicons under accession numbers SRX259838, SRX259839, SRX259843–SRX259846, and IncP-1 trfA gene amplicons under accession numbers SRX262771–SRX262776.
RESULTS
Response of bacterial communities to linuron as revealed by 16S rRNA gene-based qPCR and DGGE analysis
The addition of linuron did not significantly affect the bacterial 16S rRNA gene copy numbers among treatments and at different sampling times. In both BPS− and BPS+ microcosms, the bacterial abundance remained high with 109–1010 16S rRNA gene copies per gram BPS sample (Fig. 1). To evaluate the effect of linuron on the bacterial community composition over time, DGGE analysis of 16S rRNA genes amplified from TC-DNA extracted from the BPS− and BPS+ microcosm samples taken after 1, 12 and 25 day(s) of incubation was performed. The DGGE fingerprint (supplementary Fig. S1a) showed a high stability of the bacterial community over time with a low variation among replicates. UPGMA analysis based on Pearson correlation indices confirmed a high similarity of the bacterial fingerprints among the samples over time (supplementary Fig. S1b, similarities varied between 77% and 88%). A temporal change was observed in the BPS+ microcosm containing material from the middle and right section of the BPS. In the fingerprints of these replicates one band with a stronger intensity on days 12 and 25 compared with day 1 was detected, and these four samples also formed a separate branch in the UPGMA dendrogram, sharing 64% similarity only with the fingerprints from the other samples. This indicates a shift in the bacterial community composition in response to linuron.
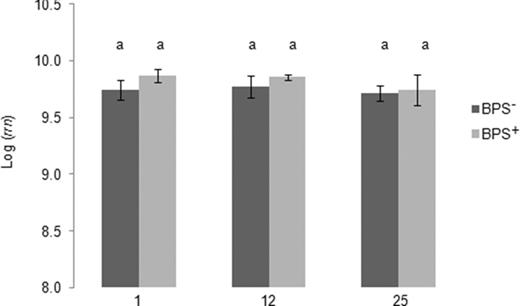
Quantification of bacterial 16S rRNA gene copy numbers (rrn) in the BPS material untreated with linuron (BPS−) or treated with linuron (BPS+) sampled on days 1, 12 and 25. Different letters indicate significant differences (Tukey's test). Error bars are standard deviation (n = 3).
Response of bacterial communities to linuron as revealed by bacterial 16S rRNA gene amplicon pyrosequencing
Altogether, 13091 amplicon sequences of the 16S rRNA gene V3–V4 region were obtained from BPS− and BPS+ TC-DNA extracted from the day 25 samples, which was the sampling day when changes in bacterial DGGE profiles were observed in the BPS material spiked with linuron (see above). After removal of low quality sequences, between 1698 and 2605 sequences per sample were left, with an average length of 353 nucleotides. The main effect of linuron in the BPS+ was observed in a significant increase in the relative abundance of Proteobacteria and a decrease of Bacteroidetes and Firmicutes (t-test, P < 0.05, data not shown). The positive responders in the BPS+ on day 25 were the proteobacterial families Comamonadaceae, Hyphomicrobiaceae, Methylophilaceae and Sphingomonadaceae, but also Microbacteriaceae and Trueperaceae (Table 1). Major phyla with a significantly decreased relative abundance in the BPS+ were Bacteroidetes and Firmicutes. Sequences classified to the family Comamonadaceae were further analysed by including the sequence in a neighbor-joining tree with sequences from known Variovorax and Acidovorax strains (supplementary Fig. S2). Acidovorax strains were included because they carry 16S rRNA genes highly similar to Variovorax, making the two difficult to distinguish based on the sequence of the 16S rRNA gene stretch amplified. One cluster of sequences recognized as positive responders to linuron was identified as Variovorax phylotype A or C while another cluster was identified as Acidovorax. Linuron degraders are found in phylotype A, which contains Variovorax sp. SRS16 (Sørensen et al. 2005), and phylotype B, which contains Variovorax sp. WDL1 (Dejonghe et al. 2003) and Variovorax sp. RA8 (Satsuma 2010). Phylotype C, which contains all remaining Variovorax phylotypes, has not previously been associated with linuron degradation. Furthermore, a small cluster of sequences was identified as Variovorax phylotype B and another as phylotype C or A, and neither of these responded to linuron. A few sequences were also identified as belonging to the Comamonadaceae genera Ramlibacter and Hydrogenophaga. The lowest Chao1 and Pielou's indices, corresponding to richness and evenness, respectively, were observed in BPS+ microcosms and were significantly lower than in the BPS− microcosms (t-test, P < 0.05) (Fig. 2).
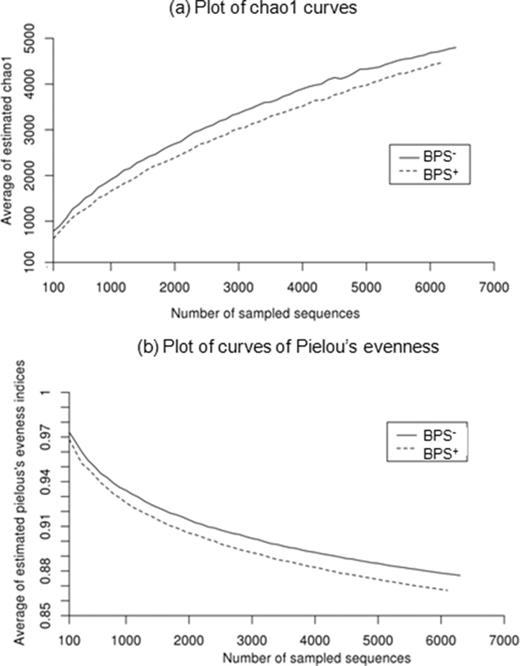
Detection of bacterial richness (chao1) (a) and Pielou's evenness (b) in the BPS− (control) and BPS+ (linuron spiked). Average diversity indices were calculated from a data set randomly sampled 100 times. A significant difference was found between BPS− and BPS+ in both (a) and (b) (t-test, P < 0.05).
Relative abundance of Bacteria responding to the spiking of BPS material with linuron analysed by pyrosequencing of 16S rRNA gene amplicons from BPS− and BPS+ on day 25.
Phylum . | Class . | Order . | Family . | Genus . | BPS− . | BPS+ . |
---|---|---|---|---|---|---|
Proteobacteria (+) | Alphaproteobacteria (+) | Rhizobiales (+) | Hyphomicrobiaceae (+) | Hyphomicrobium (+) | 1.7 ± 0 | 4.1 ± 1 |
Sphingomonadales (+) | Sphingomonadaceae (+) | 0.3 ± 0 | 0.8 ± 0 | |||
Betaproteobacteria (+) | Burkholderiales (+) | Comamonadaceae (+) | 0.4 ± 0 | 3.4 ± 1 | ||
Methylophilales (+) | Methylophilaceae (+) | 0 ± 0 | 0.7 ± 1 | |||
Bacteroidetes (−) | Sphingobacteria (−) | Sphingobacteriales (−) | Cytophagaceae (−) | Adhaeribacter (−) | 2.1 ± 0 | 0.8 ± 0 |
Pontibacter (−) | 0.9 ± 1 | 0.1 ± 0 | ||||
Actinobacteria (−) | Actinobacteria (−) | Actinomycetales (+) | Microbacteriaceae (+) | 0.6 ± 0 | 1.5 ± 0 | |
Firmicutes (−) | Bacilli (−) | Bacillales (−) | Bacillaceae (−) | 9.6 ± 0 | 7.6 ± 1 | |
Clostridia (−) | 3.8 ± 0 | 2.6 ± 1 | ||||
Acidobacteria (+) | Acidobacteria_Gp16 (+) | Gp16 (+) | 1.1 ± 1 | 1.9 ± 0 | ||
Acidobacteria_Gp6 (−) | 1.2 ± 0 | 0.6 ± 0 | ||||
Deinococcus–Thermus (+) | Deinococci (+) | Deinococcales (+) | Trueperaceae (+) | Truepera (+) | 0.3 ± 0 | 0.9 ± 0 |
Gemmatimonadetes (−) | Gemmatimonadetes (−) | Gemmatimonadales (−) | Gemmatimonadaceae (−) | Gemmatimonas (−) | 1.6 ± 0 | 0.8 ± 0 |
Phylum . | Class . | Order . | Family . | Genus . | BPS− . | BPS+ . |
---|---|---|---|---|---|---|
Proteobacteria (+) | Alphaproteobacteria (+) | Rhizobiales (+) | Hyphomicrobiaceae (+) | Hyphomicrobium (+) | 1.7 ± 0 | 4.1 ± 1 |
Sphingomonadales (+) | Sphingomonadaceae (+) | 0.3 ± 0 | 0.8 ± 0 | |||
Betaproteobacteria (+) | Burkholderiales (+) | Comamonadaceae (+) | 0.4 ± 0 | 3.4 ± 1 | ||
Methylophilales (+) | Methylophilaceae (+) | 0 ± 0 | 0.7 ± 1 | |||
Bacteroidetes (−) | Sphingobacteria (−) | Sphingobacteriales (−) | Cytophagaceae (−) | Adhaeribacter (−) | 2.1 ± 0 | 0.8 ± 0 |
Pontibacter (−) | 0.9 ± 1 | 0.1 ± 0 | ||||
Actinobacteria (−) | Actinobacteria (−) | Actinomycetales (+) | Microbacteriaceae (+) | 0.6 ± 0 | 1.5 ± 0 | |
Firmicutes (−) | Bacilli (−) | Bacillales (−) | Bacillaceae (−) | 9.6 ± 0 | 7.6 ± 1 | |
Clostridia (−) | 3.8 ± 0 | 2.6 ± 1 | ||||
Acidobacteria (+) | Acidobacteria_Gp16 (+) | Gp16 (+) | 1.1 ± 1 | 1.9 ± 0 | ||
Acidobacteria_Gp6 (−) | 1.2 ± 0 | 0.6 ± 0 | ||||
Deinococcus–Thermus (+) | Deinococci (+) | Deinococcales (+) | Trueperaceae (+) | Truepera (+) | 0.3 ± 0 | 0.9 ± 0 |
Gemmatimonadetes (−) | Gemmatimonadetes (−) | Gemmatimonadales (−) | Gemmatimonadaceae (−) | Gemmatimonas (−) | 1.6 ± 0 | 0.8 ± 0 |
(+): taxa with significantly higher relative abundance in the BPS+ samples; (−): taxa with significantly lower relative abundance in the BPS+ samples.
Relative abundance of Bacteria responding to the spiking of BPS material with linuron analysed by pyrosequencing of 16S rRNA gene amplicons from BPS− and BPS+ on day 25.
Phylum . | Class . | Order . | Family . | Genus . | BPS− . | BPS+ . |
---|---|---|---|---|---|---|
Proteobacteria (+) | Alphaproteobacteria (+) | Rhizobiales (+) | Hyphomicrobiaceae (+) | Hyphomicrobium (+) | 1.7 ± 0 | 4.1 ± 1 |
Sphingomonadales (+) | Sphingomonadaceae (+) | 0.3 ± 0 | 0.8 ± 0 | |||
Betaproteobacteria (+) | Burkholderiales (+) | Comamonadaceae (+) | 0.4 ± 0 | 3.4 ± 1 | ||
Methylophilales (+) | Methylophilaceae (+) | 0 ± 0 | 0.7 ± 1 | |||
Bacteroidetes (−) | Sphingobacteria (−) | Sphingobacteriales (−) | Cytophagaceae (−) | Adhaeribacter (−) | 2.1 ± 0 | 0.8 ± 0 |
Pontibacter (−) | 0.9 ± 1 | 0.1 ± 0 | ||||
Actinobacteria (−) | Actinobacteria (−) | Actinomycetales (+) | Microbacteriaceae (+) | 0.6 ± 0 | 1.5 ± 0 | |
Firmicutes (−) | Bacilli (−) | Bacillales (−) | Bacillaceae (−) | 9.6 ± 0 | 7.6 ± 1 | |
Clostridia (−) | 3.8 ± 0 | 2.6 ± 1 | ||||
Acidobacteria (+) | Acidobacteria_Gp16 (+) | Gp16 (+) | 1.1 ± 1 | 1.9 ± 0 | ||
Acidobacteria_Gp6 (−) | 1.2 ± 0 | 0.6 ± 0 | ||||
Deinococcus–Thermus (+) | Deinococci (+) | Deinococcales (+) | Trueperaceae (+) | Truepera (+) | 0.3 ± 0 | 0.9 ± 0 |
Gemmatimonadetes (−) | Gemmatimonadetes (−) | Gemmatimonadales (−) | Gemmatimonadaceae (−) | Gemmatimonas (−) | 1.6 ± 0 | 0.8 ± 0 |
Phylum . | Class . | Order . | Family . | Genus . | BPS− . | BPS+ . |
---|---|---|---|---|---|---|
Proteobacteria (+) | Alphaproteobacteria (+) | Rhizobiales (+) | Hyphomicrobiaceae (+) | Hyphomicrobium (+) | 1.7 ± 0 | 4.1 ± 1 |
Sphingomonadales (+) | Sphingomonadaceae (+) | 0.3 ± 0 | 0.8 ± 0 | |||
Betaproteobacteria (+) | Burkholderiales (+) | Comamonadaceae (+) | 0.4 ± 0 | 3.4 ± 1 | ||
Methylophilales (+) | Methylophilaceae (+) | 0 ± 0 | 0.7 ± 1 | |||
Bacteroidetes (−) | Sphingobacteria (−) | Sphingobacteriales (−) | Cytophagaceae (−) | Adhaeribacter (−) | 2.1 ± 0 | 0.8 ± 0 |
Pontibacter (−) | 0.9 ± 1 | 0.1 ± 0 | ||||
Actinobacteria (−) | Actinobacteria (−) | Actinomycetales (+) | Microbacteriaceae (+) | 0.6 ± 0 | 1.5 ± 0 | |
Firmicutes (−) | Bacilli (−) | Bacillales (−) | Bacillaceae (−) | 9.6 ± 0 | 7.6 ± 1 | |
Clostridia (−) | 3.8 ± 0 | 2.6 ± 1 | ||||
Acidobacteria (+) | Acidobacteria_Gp16 (+) | Gp16 (+) | 1.1 ± 1 | 1.9 ± 0 | ||
Acidobacteria_Gp6 (−) | 1.2 ± 0 | 0.6 ± 0 | ||||
Deinococcus–Thermus (+) | Deinococci (+) | Deinococcales (+) | Trueperaceae (+) | Truepera (+) | 0.3 ± 0 | 0.9 ± 0 |
Gemmatimonadetes (−) | Gemmatimonadetes (−) | Gemmatimonadales (−) | Gemmatimonadaceae (−) | Gemmatimonas (−) | 1.6 ± 0 | 0.8 ± 0 |
(+): taxa with significantly higher relative abundance in the BPS+ samples; (−): taxa with significantly lower relative abundance in the BPS+ samples.
Effects of linuron spiking on IncP-1 plasmid abundance and diversity
Relative abundance of IncP-1 plasmids determined by korB qPCR
The effect of linuron on the relative abundance of IncP-1 plasmids in the BPS− and BPS+ microcosms was evaluated by TaqMan qPCR using primers targeting the korB region of five IncP-1 subgroups (α, β, γ, δ and ɛ) (Jechalke et al. 2013). The BPS− microcosms showed stable korB copy numbers per gram BPS− material over time, with an abundance of korB relative to the 16S rRNA gene copy numbers of around 10−3 (Fig. 3). However, in the BPS+ microcosms, a significant increase (Tukey's test, P < 0.05), compared with the BPS− system was observed. An increase of the relative korB abundance from 10−3 to 10−2 was detected already in TC-DNA of samples taken on day 12, and a further increase was observed on day 25 compared with day 1, indicating a strong linuron effect on the relative abundance of IncP-1 plasmid carrying bacteria.
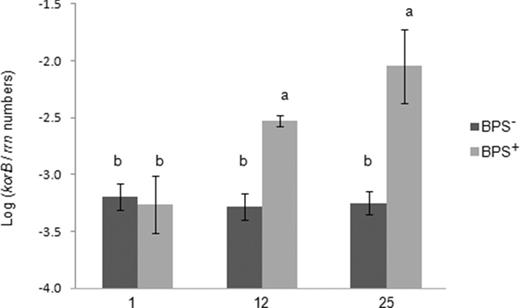
Relative abundance of korB from IncP-1 plasmids in the BPS material untreated with linuron (BPS−) or treated with linuron (BPS+) sampled on days 1, 12 and 25. korB copy numbers were normalized for bacterial 16S rRNA gene copy numbers (rrn) quantified from the same sample. Different letters indicate significant differences (Tukey's test). Error bars are standard deviation (n = 3).
trfA-based pyrosequence analysis
The effect of linuron on the diversity of IncP-1 plasmids and their relative abundance was further evaluated by amplicon pyrosequencing of a region of the trfA gene. After denoising and chimera removal, 45 955, 18 821 and 21 536 sequences were obtained from primer sets targeting IncP-1αβɛ, IncP-1γ and IncP-1δ, respectively. Sequences were assigned to the different IncP-1 subgroups by clustering at 89% nucleotide sequence similarity. Twenty-one trfA sequences from known sequenced IncP-1 plasmids were included in the clustering as reference for the subgroups α, β-1, β-2, γ, δ, ɛ and ζ. Eighteen sequence clusters were identified, and the reference sequences were found in eight of the clusters (Fig. 4a). There was a small and almost negligible overlap in the IncP-1 subgroups targeted by the three primer sets, the largest overlap being the δ primer set also amplifying IncP-1β-1. However, the relative abundances of the trfA amplicons are primer-specific, and thus comparisons cannot be made between sequences obtained with the different primer sets. Furthermore, the numbers of sequences are not related to the abundance of the different IncP-1 subgroups in the TC-DNA samples because of the method used for amplicon library construction. IncP-1α sequences were only detected in the BPS− microcosms and contributed 0.01% of the sequences amplified with the αβɛ primer set. The sequences found in the clusters representing the β-1, β-2, γ, δ and ɛ subgroups (Fig. 4a clusters 11, 19, 9, 21 and 18, respectively), and encompassing the large majority of sequences were extracted and re-clustered at 97% nucleotide sequence similarity to identify possible changes in abundance within the subgroups. After re-clustering, the sequences from the original clusters split into many new clusters, with reference sequences found in several of these (Fig. 4b). For instance, cluster 9 split into clusters 9.1 and 9.3. The 97% sequence similarity was chosen as a conservative estimate to avoid potential sequencing errors not removed by denoising and chimera checking. Pyrosequencing has an error rate of about 1% (Huse et al. 2007), and the sequences have a size of only 237 bp. No sequences were obtained that clustered with IncP-1ζ (cluster 2), the IncP-1γ plasmids pKS208 (cluster 12) and pMBUI1 (cluster 9.1) and IncP-1β-2 plasmid pB1 (cluster 19.7) as the primer sets do not target these plasmids. For β-1, the majority of amplicon sequences were found in cluster 11.101, along with most of the β-1 reference sequences. No sequences clustered with pB136 (cluster 11.25, Fig. 4b). For β-2, almost all amplicon sequences were found in cluster 19.51 together with the β-2 reference sequences. The IncP-1ɛ sequences split into two major clusters with the majority of amplicon sequences clustering with pKJK5 in 18.64 and with pEMT3 in 18.74. Two small IncP-1ɛ clusters (18.49, 18.87) formed mainly with sequences from BPS− samples were also found. These were most similar to pEMT3. All sequences identified in the γ-subgroup clustered with pQKH54 (cluster 9.3) as expected since it is the only known IncP-1γ plasmid targeted by the primer set. A list of all reference plasmids used and the clusters is given in supplementary Table S1. The representative sequences from all clusters in Fig. 4b differed after translation into amino acids. The spiking of the BPS material with linuron resulted in significant changes in the abundance of the different IncP-1 subgroups with an increase of β-1 and a decrease of β-2 and ɛ subgroups (β-2 not significant, P > 0.05).
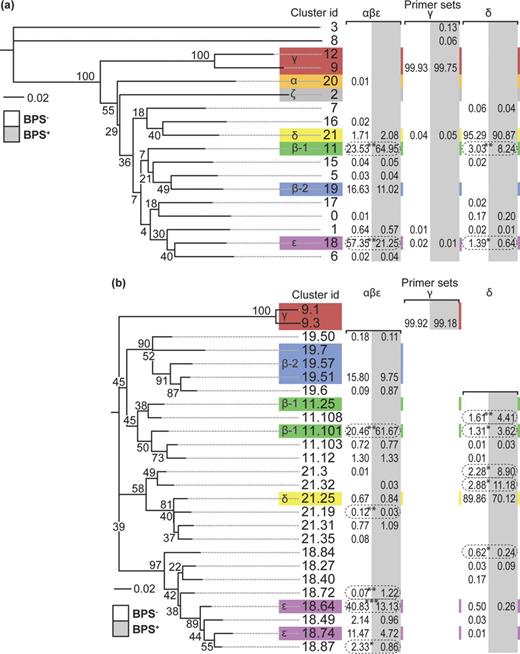
Neighbor-joining trees of clusters of IncP-1 trfA amplicons sequences from BPS− and BPS+ sampled on day 25. (a) Each branch represents one cluster of sequences with 89% sequences similarity. (b) Sequences from clusters 9, 11, 18, 19 and 21 in (a) re-clustered at 97% sequence similarity. Small clusters are not included except if they contain reference sequences. In both panels known reference sequences were included in the clustering and their locations and subgroup names are indicated by colored boxes. The tables show the mean relative abundance of sequences from each primer set. There is an overlap in targets between primer sets. Dashed ovals indicate significant changes in abundance between BPS− and BPS+ (Student's t-test, n = 3, * P < 0.05, ** P < 0.01, *** P < 0.001). Numbers on branches are bootstrap-values as a percentage of 1000 bootstraps.
Exogenous plasmid isolation and characterization
In an attempt to capture plasmids conferring the ability to utilize linuron as carbon source, biparental exogenous isolation of plasmids into Pseudomonas putida KT2442 gfp was applied using minimal media containing linuron (20 μg mL−1). However, no transconjugants were obtained. Following this an alternative approach was tried to capturing plasmids potentially involved in linuron degradation by biparental exogenous isolation with HgCl2 (20 μg mL−1) as selective marker, and once again, Pseudomonas putida KT2442 gfp was used as the recipient cell. The frequency of plasmid transfer was about two orders of magnitude higher in BPS− (10−6) compared with the BPS+ microcosms (10−8). Transfer frequencies were 6.2 × 10−6, 3.8 × 10−5 and 5.0 × 10−6 in BPS− (middle, right and left section) and 5.9 × 10−8 and 2.2 × 10−8 in BPS+ (right and left; no transconjugants were obtained from material of the middle section). The number of recipient cells in all BPS− and BPS+ replicates was quite high, ranging from 109 and 1010 cells per gram. A total of 50 transconjugants (25 from both BPS− and BPS+) were picked, and out of them, 18 and 24 from BPS− and BPS+, respectively, grew on a fresh Mueller–Hinton (MH) agar plate (Merck KGaA, Darmstadt, Germany) containing HgCl2 (20 μg mL−1). Specific PCR amplifications for IncP-1, IncP-7 and IncP-9 plasmids indicated that IncP-9 plasmids were captured in all transconjugants from BPS+ and BPS− while IncP-1 plasmids were mainly found in the transconjugants from BPS− but also in six of the 24 transconjugants from BPS+. Non-specific bands were observed with the IncP-7 primers (data not shown). Southern blot hybridizations of digested plasmid DNA from each transconjugant were performed by using IncP-1 probes (IncP-1β and IncP-1ɛ) and IncP-9 mixed probe were performed. Of the 42 transconjugants analysed, 15 from BPS− microcosms and six from BPS+ carried IncP-1 plasmids. Southern blot hybridization with IncP-1β and IncP-1ɛ specific probes indicated that all exogenously isolated IncP-1 plasmids belonged to the IncP-1β subgroup. Positive hybridization signals with the IncP-9 mixed probe were observed by Southern blot hybridization of BstI/PstI digested plasmids for all 42 transconjugants, confirming that all transconjugants contained IncP-9 plasmids (data not shown).
In order to study the diversity of plasmids captured, five different restriction profiles were generated (supplementary Fig. S3). While the plasmid DNA of transconjugants from BPS− microcosms displayed five different restriction patterns, only one pattern was observed in the transconjugants from BPS+, indicating a higher diversity of IncP-1 plasmids in BPS− samples. One restriction pattern type that was observed for all six IncP-1 containing transconjugants from the BPS+ was also displayed by one of the IncP-1 positive transconjugants from BPS−. Eighteen out of the 24 transconjugants from BPS+ carried only IncP-9 plasmids and three different restriction patterns were observed, while nine of the transconjugants from BPS− carried only IncP-9 plasmids, which displayed identical restriction patterns.
Plasmid DNA from IncP-1 positive transconjugants from BPS+ was screened for the presence of libA and hylA genes, and the hylA was detected in three of the transconjugants. No PCR products were obtained with the libA-specific primers. Southern blot hybridization of Sma1-digested plasmid DNA with the hylA probe confirmed the presence of hylA for the PCR-positive transconjugants (Fig. 5). The triparental mating with one of the P. putida transconjugants and E. coli pSM1890 as donors and C. necator as recipient confirmed the presence of the gene-mobilizing IncP-1 plasmid as the IncQ plasmid pSM1890 was successfully mobilized. Plasmid DNA extracted from one transconjugant colony with green fluorescence and gentamycin and streptomycin resistance (antibiotic markers of pSM1890) was shown to be hylA positive by both, PCR and Southern blot hybridization. Surprisingly, PCR screening revealed that the pSM1890 and hylA positive C. necator transconjugant did not carry the IncP-1 plasmid. Thus the localization of hylA remains to be elucidated by sequencing of the plasmid content of both the P. putida and the C. necator transconjugants.
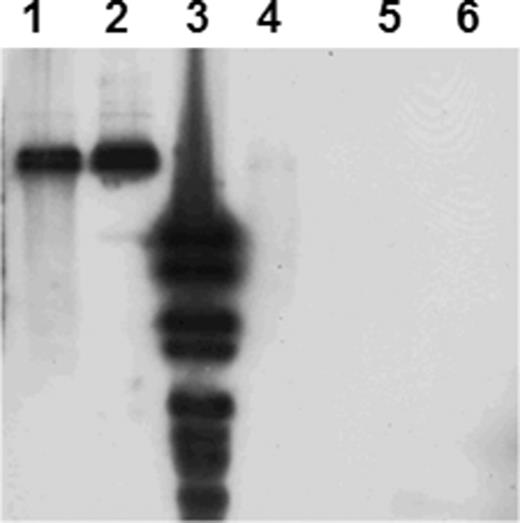
Southern blot hybridization of SmaI-digested plasmid DNA with hylA probe. Lane 1, P. putida KT2442 (hylA positive transconjugant of the biparental mating); lane 2, C. necator (hylA positive transconjugant of the triparental mating); lane 3, DIG VI ladder, Fermentas; lane 4, Variovorax sp. strain WDL1 (positive control); lane 5, P. putida KT2442 (recipient of the biparental mating) and lane 6, C. necator (recipient of the triparental mating).
DISCUSSION
In the present study, pre-adapted bacterial communities of BPS material were exposed to rather high concentrations of linuron in a microcosm set-up. For the first time, amplicon sequencing revealed clear effects of linuron on the structure of BPS bacterial communities and changes in the relative abundance of populations carrying different IncP-1 plasmid subgroups over time.
A cultivation-independent 16S rRNA gene-based analysis was performed to better understand the response of the bacterial community of a BPS to the application of linuron. The 16S rRNA gene-based DGGE fingerprints indicated only minor changes of the dominant bacteria in response to linuron in the microcosms with material from the middle and right section of the BPS in Kortrijk (Belgium). Earlier studies did not show effects of single or multiple applications of linuron on the bacterial community structure in agricultural soil, both in microcosms and in the field (Bers et al. 2011a, 2013) and in microcosms with a simulated BPS matrix (Sniegowski et al. 2011), which was the reason for using higher concentrations of linuron in the present study.
In contrast to the DGGE analysis, the 16S rRNA gene pyrosequencing results indicated larger shifts in bacterial communities in response to linuron spiking. One of the largest positive responders in the BPS+ was an OTU with high sequence identity with Variovorax, a genus often associated with linuron degradation (Field et al. 1997; Dejonghe et al. 2003; Breugelmans et al. 2007; Bers et al. 2011a,b, 2012; Sniegowski et al. 2011). Another major responder with increased relative abundance in the BPS+ was the Hyphomicrobiaceae family. Members of this family were reported to be enriched in synergistic linuron degrading consortia in which they contribute to linuron mineralization by degrading and utilizing N,O-dimethylhydroxylamine (N,O-DMHA) which is in addition to 3,4-dichloroaniline (3,4-DCA), the product of Variovorax-mediated initial linuron hydrolysis (Dejonghe et al. 2003; Breugelmans et al. 2007). The increased relative abundance of Hyphomicrobiaceae to linuron application suggests that those organisms might also feed on N,O-DMHA originating from linuron hydrolysis in BPSs and further point to the occurrence of linuron-based food webs in the BPS that are similar to those observed in consortia obtained by enrichment (Dejonghe et al. 2003). Other bacterial groups showing a clear increase in relative abundance were Gp16 (Acidobacteria), Microbacteriaceae (Actinobacteria) and Truepera (Deinococcus–Thermus). Actinobacteria and Acidobacteria are common and abundant phyla in both agricultural and contaminated soil (Holmsgaard et al. 2011; Berg et al. 2012) while Deinococcus–Thermus is rare. The actinobacterial isolates Arthrobacter globiformis D47 (Turnbull et al. 2001) and Mycobacterium brisbanense JK1 (Khurana et al. 2009), both of them not belonging to the Microbacteriaceae, were previously reported as linuron degraders. To date only one strain type of Trueperaceae has been described. This strain is radiation-resistant and originated from a hot spring (Albuquerque et al. 2005). No type isolates exist for Gp16. Therefore, we can only speculate on their involvement in linuron degradation if any.
The quantification of IncP-1 plasmids in TC-DNA by means of the korB qPCR system showed unequivocally that IncP-1 plasmids significantly increased in BPS+ material compared with the BPS− material, confirming the correlation of IncP-1 plasmid abundance and pesticide pollution that was recently proposed for an on-field BPS monitored for IncP-1 plasmid abundance over an agricultural season (Jechalke et al. 2013; Dealtry et al. 2014b). The field experiment was obviously lacking an untreated control and proper replication.
IncP-1 plasmids were previously reported to occur in several contaminated environments like soils, manure, sewage and mangrove (Smalla et al. 2006; Malik et al. 2008; Gomes et al. 2010; Oliveira et al. 2012; Jechalke et al. 2013). In several studies a correlation of IncP-1 plasmid abundance and pollution was assumed, but the experimental design was often not suitable to demonstrate this correlation. Dunon et al. (2013) previously showed such a correlation in microcosm experiments with agricultural soil as well as with an artificial biomix matrix. In both cases, the relative abundance of IncP-1 plasmids appeared to increase when the microcosms were treated with pesticide compounds including linuron. In addition, high relative abundance of IncP-1 plasmids was found in biomix samples of various BPSs in operation (Dealtry et al. 2014a). The present study showed that the relative abundance of IncP-1 plasmids in a BPS matrix in the field responds to linuron application. As in agricultural soil, an increase in IncP-1 abundance in BPS+, as shown by the korB qPCR data, was associated with an increased relative abundance of Variovorax 16S rRNA genes as shown by amplicon pyrosequencing (supplementary Fig. S2), providing indications that IncP-1 plasmids might be hosted by Variovorax species or others related to linuron degradation. In the linuron degradation pathway, the gene coding for an enzyme involved in the degradation of 3,4-DCA was localized on IncP-1 plasmids (Król et al. 2012). Changes in the abundance of IncP-1 subgroups were shown by amplicon pyrosequencing of trfA genes amplified from TC-DNA with three different primer systems. Analysis of the trfA gene amplicons from TC-DNA of BPS− and BPS+ taken on day 25 showed a high diversity of IncP-1 plasmids belonging to various subgroups in the BPS samples and differences in the relative abundance of the IncP-1 plasmid subgroups between the BPS− and BPS+ samples (Fig. 4a and b). As the three different primer systems described by Bahl et al. (2009) were used for generating the PCR products for amplicon sequencing, a comparison of the relative abundance was only possible for IncP-1α, IncP-1β and IncP-1ɛ as these amplicons were obtained with the same primer system. Considering the broad host range of IncP-1 plasmids, we suggest that degradative genes might be shared among BPS bacteria through conjugation or mobilization. Shifts in the relative abundance of the IncP-1β and IncP-1ɛ subgroups were observed in response to the linuron treatment. A significantly higher number of sequences sharing high similarity (97%) to IncP-1β-1 plasmids were observed in the BPS+. Several catabolic plasmids such as pADP-1, pUO1, pJP4 and pWDL7 (Martinez et al. 2001; Sota, Kawasaki and Tsuda 2003; Ledger, Pieper and Gonzalez 2006; Król et al. 2012), but also the antibiotic resistance plasmids R751 and pB10 and mercury resistance plasmid pTP6, belong to this group. In contrast, sequences with similarity to IncP-1β-2 plasmid such as pA81, pNB8c (Król et al. 2012) and pPC1-1 were detected in a higher abundance in the BPS−. The highly significant decrease in the relative abundance of plasmids with similarity to IncP-1ɛ observed in the present study confirms the findings for the BPS in Kortrijk monitored over an agricultural season (Dealtry et al. 2014b). Populations carrying plasmids belonging to the IncP-1ɛ group with high similarity to pKJK5 and pEMT3 decreased in abundance as their hosts were likely decreased in relative abundance and the IncP-1ɛ plasmid did not provide a selective advantage in the presence of linuron. The very low abundance of IncP-1α trfA sequences was surprising as manure was previously added as a nutrient source to BPS, and IncP-1α plasmids were previously reported in manure (Smalla et al. 2000). Due to the absence of a control in the BPS study under field conditions (Dealtry et al. 2014b), only the present study unequivocally shows that the changes observed in the abundance and diversity of IncP-1 plasmids were caused by the presence of linuron.
However, our attempts failed to exogenously capture IncP-1 plasmids conferring the ability to degrade linuron into Pseudomonas putida recipient strains by selecting transconjugants on minimal media containing linuron as sole carbon source. We speculate that either the linuron concentration in the medium was too low or that the linuron degradative genes were not expressed in the Pseudomonas putida background, or that they did not encode the initial hydrolysis step for linuron degradation. Therefore, based on the knowledge that IncP-1 catabolic plasmids often carry mercury resistance genes (Smalla et al. 2006; Sen et al. 2011), we tried to capture IncP-1 plasmids by selecting transconjugants on MH medium containing HgCl2. Contrary to our expectations, higher numbers of transconjugants were obtained from the BPS− than from the BPS+. Although all IncP-1 plasmids captured from BPS+ and BPS− microcosms belonged to IncP-1β plasmid subgroup, based on the diversity of restriction patterns, a higher diversity of IncP-1β plasmids was captured from BPS−. Five types of restriction patterns of IncP-1β plasmids were isolated from BPS−, while only one restriction pattern of IncP-1β plasmids was captured from the BPS+, indicating the influence of linuron on the composition of IncP-1 plasmids. Obviously the abundance of populations with IncP-1 plasmids conferring mercury resistance decreased in the BPS+, suggesting that the bacterial populations with IncP-1 plasmids that were significantly increased in BPS+ probably did not confer mercury resistance.
Enzymes encoded by hylA and libA genes were recently shown to initiate the first step of the hydrolysis of linuron to 3,4-dichloroaniline and N,O-dimethylhydroxylamine. Both PCR screening and Southern blot hybridization of digested plasmid DNA revealed that three of the transconjugants that captured based IncP1-β plasmids and IncP-9 plasmids also carried the hylA gene. The recently identified HylA represents an alternative linuron hydrolase detected in the Variovorax sp. strain WDL1 (Bers et al. 2013). The ability of IncP-1 plasmids to mobilize IncQ plasmids was exploited to further investigate the localization of hylA on IncP-1 plasmids. Unexpectedly, a C. necator transconjugant positive for pSM1890 and hylA did not contain the IncP-1 plasmid. Interestingly, also Bers et al. (2013) reported that a mutant of the Variovorax sp. strain WDL1 lost its ability to hydrolyze linuron. Sequencing of the C. necator transconjugants will be required to elucidate the localization of hylA genes.
The presence of IncP-9 plasmids in all transconjugants is not a surprise as previous studies involving a BPS contaminated with different pesticides showed a high abundance of those plasmids in such an environment (Dealtry et al. 2014a,b). The high abundance of IncP-9 plasmids might be explained by the high concentration of aromatic compounds in the BPS studied and the presence of genes encoding different aromatic-ring-degrading enzymes in those plasmids (Dennis 2005; Gomes et al. 2010). However, no correlation between IncP-9 plasmid abundance and linuron pollution was found.
In conclusion, our results indicate that linuron degradation food webs, involving genera like Variovorax, Hyphomicrobium and Microbacteriaceae, previously observed in linuron degrading enrichment cultures from agricultural soil also proliferated as a response to linuron in BPS material and probably play a role in linuron degradation in BPS. Besides the known Variovorax and Hyphomicrobium responders to linuron, novel potential bacterial taxa responding to linuron were indicated, affiliating to Methylophilaceae, Sphingomonadaceae and Trueperaceae. Moreover, it was demonstrated that IncP-1 plasmids were enriched in response to linuron and that their diversity changed as indicated by amplicon sequencing of IncP-1-specific trfA sequences. The increased IncP-1 plasmid abundance might be due to enrichment either of bacteria carrying IncP-1 plasmids with degradative genes, or of bacteria that have acquired the degradative genes by mobilization, a process in which the IncP-1 plasmids were co-transferred. Three exogenously captured plasmids carried hylA genes involved in the first step of the linuron degradation. The present study contributes to a better understanding of the microbiology of pesticide biodegradation in BPSs and the further acceptance and implementation of BPSs as a technology.
SUPPLEMENTARY DATA
This study was funded by the EU 7th Framework Programme (MetaExplore 222625) and the Inter-University Attraction Pole (IUAP) ‘μ-manager’ of the Belgian Science Policy (BELSPO, P7/25). Additional acknowledgements are given to the CAPES-PNPD (Brazilian Ministry of Education) for funding the postdoctoral fellowship of S.D. Tácio M. P. de Campos (Catholic University of Rio de Janeiro) and I-M. Jungkurth (JKI) are acknowledged for proof-reading and valuable comments and suggestions on the manuscript.
Conflict of interest. None declared.
REFERENCES