-
PDF
- Split View
-
Views
-
Cite
Cite
Ilona Gasser, Henry Müller, Gabriele Berg, Ecology and characterization of polyhydroxyalkanoate-producing microorganisms on and in plants, FEMS Microbiology Ecology, Volume 70, Issue 1, 3 September 2009, Pages 142–150, https://doi.org/10.1111/j.1574-6941.2009.00734.x
- Share Icon Share
Abstract
Polyhydroxyalkanoates are energy reserve polymers produced by bacteria to survive periods of starvation in natural habitats. Little is known about the ecology of polyhydroxyalkanoate producing bacteria. To analyse the occurrence of this specific group on/in seven different plant species, a combined strategy containing culture-dependent and -independent methods was applied. Using microbial fingerprint techniques (single strand conformation polymorphism analysis with specific primers for phaC gene encoding the key enzyme of the polyhydroxyalkanoate synthesis), a high number of bands were especially found for the rhizosphere. Furthermore, cluster analysis revealed plant species specific communities. Isolation of bacteria, recognition of brightly refractile cytoplasmatic inclusions, lipophilic stainings and a PCR strategy targeted on the phaC gene were used as a culture dependent strategy for the detection of polyhydroxyalkanoate producing bacteria. Results again represent a high degree of plant specificity: the rhizosphere of sugar beet contained the highest number of positive strains. This was confirmed by quantitative PCR: the relative copy number of phaC was statistically and significantly enhanced in all rhizospheres in comparison with bulk soil. New polyhydroxyalkanoate producing bacterial species were detected: for example, Burkholderia terricola, Lysobacter gummosus, Pseudomonas extremaustralis, Pseudomonas brassicacearum and Pseudomonas orientalis. Our results confirm the hypothesis that the rhizosphere is an interesting hidden reservoir for polyhydroxyalkanoate producers.
Introduction
Plants constitute microbial ecosystems, which harbour diverse and specific bacterial communities (Smalla et al., 2001; reviewed by Garbeva et al., 2004; Berg & Smalla, 2009). A long list of microorganisms are known for positive interactions with their host plant and fulfil important functions for them (Whipps, 2001; Lugtenberg et al., 2002; Raaijmakers et al., 2008). One of these functions is plant growth promotion by bacterial synthesis of phytohormones, the breakdown of plant-produced ethylene by bacterial production of 1-aminocyclopropane-1-carboxylate deaminase and/or increased mineral and N availability in soil (Glick et al., 2004). Another important function of plant-associated bacteria is pathogen defence (Cook et al., 1995; Weller et al., 2002). However, so far not all plant–microorganism interactions and their effects on each other are understood.
Plants themselves can be divided into different microenvironments, which are characterized by specific biotic and abiotic conditions. Especially in the rhizosphere, according to root exudations, the conditions and nutrient contents are dynamically changing (Bais et al., 2006). Indigenous microorganisms must be adapted to the changing conditions of their environment and fluctuations in the concentration of nutrients exuded by plant roots. Prokaryotes have evolved multitudinous mechanisms of resistance towards stress conditions such as those that have an inherent ability to form cysts and spores to survive desiccated environments (Potts, 1994). Additionally, bacteria are able to exhibit a metabolic versatility in order to overcome fluctuations in the chemical conditions of their environment. Inclusion of storage substances is another bacterial strategy that increases survival in changing surroundings (Müller et al., 1999). Polyhydroxyalkanoates are one such group that bacteria produce to store energy (Steinbüchel et al., 2002). A long list of bacterial species such as Ralstonia eutropha, Bacillus megaterium and Pseudomonas oleovorans are able to accumulate polyhydroxyalkanoates (Füchtenbusch et al., 2000; Rehm et al., 2002; McCool et al., 2006). The synthetic pathway was already analysed in detail (Rehm & Steinbüchel, 1999; Steinbüchel et al., 2006), and on this basis, biotechnological applications for the biodegradable polymers as bioplastics were developed (Zinn et al., 2001; Chen & Wu, 2005; Koller et al., 2007). The majority of polyhydroxyalkanoate-producing bacteria were isolated from soil and activated sludge (Wang & Bakken, 1998; Khardenavis et al., 2005). Recently, new bioresources such as marine environments were analysed regarding their potential to harbour new polyhydroxyalkanoate producers (Arun et al., 2009). In these studies, culture-dependent strategies such as microscopic methods or chemical extractions of polyhydroxyalkanoates were applied to isolate and characterize the polyhydroxyalkanoate production of bacteria. Although fast-growing Rhizobium species, which are naturally occurring inhabitants of plants, are well known as producers of polyhydroxyalkanoates in sludge and industrial wastewater (Kadouri et al., 2005; Ben Rebah et al., 2009; Ratcliff & Denison, 2009), little is known about plants as a reservoir for polyhydroxyalkanoate-producing bacteria. The hypothesis of this study was that especially the rhizosphere should provide conditions under which diverse polyhydroxyalkanoate-producing bacteria are enriched.
The objective of this work was to analyse the diversity and distribution of polyhydroxyalkanoate-producing bacteria in the rhizosphere and other microenvironments of seven different plant species by a hierarchical combination of cultivation-dependent and -independent methods. By the cultivation-dependent approach, bacterial isolates were screened for their ability to form polyhydroxyalkanoates in vitro as well as for the presence of polyhydroxyalkanoate-synthase genes using a PCR strategy. On the other hand, a cultivation-independent method [single-strand conformation polymorphism (SSCP) analysis with specific primers for phaC, targeted the key enzyme of the polyhydroxyalkanoate synthesis] was used to determine the occurrence of genes within the microbial community of plant habitats. Both strategies resulted in the conclusion that the rhizosphere is an interesting reservoir for polyhydroxyalkanoate producers and harbours as yet undiscovered potential of these biotechnologically relevant bacteria.
Materials and methods
Experimental design and sampling
For a preliminary screening, we analysed the genetic potential of single strains and total bacterial communities to synthesize polyhydroxyalkanoates. Therefore, we used isolates from the culture collection Strain Collection of Antagonistic Microorganisms (SCAM), Graz University of Technology, Austria and samples from other research projects of our working group. The latter included samples from the rhizosphere of oilseed rape (Brassica napus L.), polygonum (Polygonum bistorta L.), olive (Olea europaea L.), sugar beet (Beta vulgaris L.) and from the phyllosphere and carposphere of strawberry (Fragaria×ananassa [Duchesne] Decaisne & Naudin) and grapevine (Vitis vinifera L.) (Zachow et al., 2008; Schmid & Berg, 2009). In addition, bacterial communities isolated from peat mosses (Sphagnum fallax Klinggr.) were tested (Opelt et al., 2007).
For a systematic study, bacterial communities of two different field trials were analysed. In Field trial 1, in Deggendorf (Germany), bacteria were isolated from rhizosphere of sugar beet (B. vulgaris L.), oilseed rape (B. napus L.) and wheat (Triticum aestivum L.). In Field trial 2, strawberry (Fragaria×ananassa [Duchesne] Decaisne & Naudin cv. Elsanta) and oilseed rape (B. napus L.) rhizosphere-associated bacteria were investigated at three different growth stages of host plants (young, flowering and senescent plants) at different locations in Germany (Braunschweig, Rostock). The different crop plants were grown in a randomized block design with a minimum of four replicates per crop plant. In general, plants were analysed at three different developmental stages: (1) young plants, (2) flowering plants and (3) early senescent plants. Detailed information about the field trials are described in Zachow et al., 2008 (Field trial 1) and Berg et al., 2006 (Field trial 2).
For all analyses, plant roots with adhering soil, leafs, stems and fruits of investigated plants were sampled into sterile plastic bags. Samples taken from six plants were considered as one composite sample. For the isolation of plant-associated microorganisms 5g each of roots and other organs were pooled and transferred into a new Stomacher bag. Samples were extracted in a Stomacher laboratory blender (BagMixer, Interscience, St Nom, France). After addition of 50mL 0.85% NaCl solution samples were shaken for 3min; and then the supernatants were decanted into 50-mL tubes. The supernatants decanted into 50-mL tubes were used for cultivation as well as for cultivation-independent investigation procedures.
Isolation of community DNA for SSCP and quantitative real time (qRT)-PCR analysis
Total genomic DNA from soil microbial communities were extracted by mechanical disruption and homogenization of up to 500mg soil in a FastPrep® Instrument (MP Biomedicals, Carlsbad) for 40s at speed 6.0. Afterwards, DNA was purified by the FastDNA® Spin Kit for soil (MP Biomedicals). DNA was additionally purified by the GeneClean Turbo Kit (MP Biomedicals) containing the special binding buffer guanidine thiocyanate. The procedure of SSCP analysis was carried out according to Schwieger & Tebbe (1998) using the phaC-specific primers I-179L (5′-ACAGATCAACAAGTTCTACATCTTCGAC-3′) and 5′phosphorylated I-179R (5′-GGTGTTGTCGTTGTTCCAGTAGAGGATGTC-3′) (Solaiman et al., 2000) in a reaction mixture of 30μL Taq&Go PCR Mastermix (MP Biomedicals) supplemented with 0.5μM of each primer and about 60–80ng of template DNA. The thermal cycle programme consisted of one cycle of 94°C for 5min, 53°C for 1min, 72°C for 2min; 36 cycles of 94°C for 1min, 53°C for 1min, 72°C for 2min; and a final incubation step at 72°C for 10min. The PCR products were purified by the GeneClean Turbo Kit (MP Biomedicals) and subjected to a λ-exonuclease (New England Biolabs, Ipswich) digestion and DNA single-strand folding. The polyacrylamide gel electrophoresis was performed on a TGGE apparatus (Biometra, Göttingen, Germany) at 26°C and 400V for 24h using 8% (w/v) acrylamide gels. Afterwards, the gels were silver stained according to the procedure described by Bassam et al. (1991).
To determine the relative abundance of the gene phaC, a qRT-PCR using the RotorGene 6000 apparatus (Corbett Research, Sydney, Australia) was performed. The same PCR conditions and primer set as described above, except for the addition of SybrGreen I to a final concentration of 0.4 × (Invitrogen, Carlsbad) and exactly 40ng template DNA, were applied. Following the PCR reactions, the amplicons were verified by melting-point analysis and agarose gel electrophoresis. Four samples of each habitat were analysed in two independent PCR experiments as duplicates. Additionally, a standard curve was generated by quantifying phaC in aliquots of a dilution series of a total community DNA sample. Abundances of the amplified gene in relation to the soil samples were calculated by applying the equation , where E is the PCR efficiency according to the standard curve. For statistical analyses, calculated values were subjected to post hoc Tukey's test.
Isolation of bacteria
An aliquot was taken from the supernatant as described above in the sampling procedure, serially diluted and plated onto R2A, a nutrient-poor medium suitable for the growth of diverse plant-associated bacteria (Difco, Detroit). Plates were incubated for 3 days at 20°C. Colonies that developed on agar were differentiated by colour, elevation, shape and edge appearance. From each sample, 16 colonies were picked up with a sterilized toothpick and plated onto Luria–Bertani (LB) plates for further screening.
Screening of polyhydroxyalkanoate-producing bacteria
Bacterial isolates were screened for their genetic potential for polyhydroxyalkanoate production and their in vitro ability to produce polyhydroxyalkanoates. The screening was based upon both molecular and microscopic approaches. Colony PCR techniques were used for screening bacterial polyhydroxyalkanoate producers isolated from the environment using a phaC-specific primer set (Sheu et al., 2000) in a PCR reaction mixture with a total volume of 30μL consisting of 5 × Taq&Go PCR Mastermix (MP Biomedicals), 0.5μM of each primer [phaCF1: 5′-ATCAACAA(GGG/A)T(TT/A) CTAC(AA/G)TC(CC/T)T(CC/G)GACCT-3′ and phaCR4: 5′-AGGTAGTTGT(TT/C)GAC(CCC/GG)(AAA/CC)(AAA/CC)(GGG/A)TAG(TTT/G)TCCA-3′], 3% dimethyl sulphoxide and 1M betaine (Sigma). The thermal cycle programme consisted of one cycle of 94°C for 10min, 51°C for 2min, 72°C for 2min; 35 cycles of 94°C for 20s, 57°C for 45s, 72°C for 1min; and a final incubation step at 72°C for 5min. One microlitre of a DNA preparation obtained by suspending cell material from an overnight culture in 50μL demineralized water and incubating at 96°C for 10min served as the template. PCR-amplified DNA fragments were observed by gel electrophoresis using 1.0% agarose gels. Five microlitres of each amplification mixture were subjected to agarose gel electrophoresis and ethidium bromide staining. The amplified DNA fragments were visualized by UV illumination. Exemplarily, DNA fragments were sequenced to prove their identity. To detect the formation of polyhydroxyalkanoate-containing granules microscopically, bacteria were plated onto a solid minimal medium containing high amount of glucose (1.0gL−1) and low amount of nitrogen (0.5gL−1 NH4Cl), which is optimized for polyhydroxybutyrate production. Plates were incubated for at least 5 days at 30°C before the cells were stained by Sudan black B (Steinbüchel & Oppermann-Sanio, 2003).
Identification and characterization of polyhydroxyalkanoate-producing bacteria
A single colony on LB medium was picked with a sterile toothpick into 50-μL demineralized water in a 1.5-mL Eppendorf tube. The cell suspension was heated to 96°C for 10min. The 30-μL reaction mixture contained 6μL 5 × Taq&Go (MP Biomedicals), 1.5μL of primer pair mix EubI-forward (5′-GAGTTTGATCCTGGCTCAG-3′) and 1492r-reverse (5′-TACGGYTACCTTGTTACGACTT-3′) both at a concentration of 10pmolμL−1 and 20–30ng template. The PCR products were purified with GeneClean Turbo Kit as recommended by the manufacturer. The fragments were sequenced using the reverse primer 1492r. For identification of related sequences, a database alignment using blast algorithm was performed.
Altogether, 58 representative strains were characterized by their BOX fingerprint using the BOXA1R primer (5′-CTACGGCAAGGCGACGCTGACG-3′) (Rademaker & De Bruijn, 1997). BOX groups were defined according to the similarity of BOX patterns and cluster analysis at 80%. To estimate this borderline, we used a higher proportion than evaluated for variability by analysing three independent replicates of the same strain (<89%).
Statistics
Computer-assisted evaluation of polyhydroxyalkanoate community profiles obtained by SSCP and BOX-PCR was performed using the gelcompar® program, version 4.1 (Applied Maths, Kortrijk, Belgium). Cluster analysis was performed with the unweighted pair group method with arithmetic average algorithm.
Nucleotide sequence accession numbers
Bacterial sequence accession numbers for sequences submitted to the EMBL nucleotide sequence database are FN313510–FN313537.
Results
Preliminary screening for polyhydroxyalkanoate-producing bacteria in plant-associated communities
To get an overview of the occurrence and diversity of polyhydroxyalkanoate-producing bacteria in plant-associated microenvironments, total community DNA and single-strain DNA derived from different plant species (oilseed rape, polygonum, olive, sugar beet, strawberry and grapevine) as well as from different microenvironments (phyllosphere, carposphere, rhizosphere and endosphere) were analysed for the occurrence of phaC genes. While no positive results were obtained for all aboveground microenvironments (phyllosphere and carposphere), in endo- and rhizosphere samples, the occurrence of phaC could be demonstrated. This was confirmed for all plant species. For example, total community DNA of 10 olive endospheres and 11 olive rhizospheres were tested on phaC genes. Interestingly, only in samples from rhizospheres (nine out of 10), but not in endosphere samples, phaC genes were detected.
Polyhydroxyalkanoate-producing bacteria in the rhizosphere of sugar beet, wheat and oilseed rape (Field trial 1)
In a comparative study, 180 bacteria were isolated from the rhizosphere of sugar beet, oilseed rape and wheat. We applied two methods to detect polyhydroxyalkanoate-producing organisms: microscopic analysis and PCR detection of the phaC gene. On comparing results obtained by PCR, sugar beet was found to be associated with a higher number (90%) of polyhydroxyalkanoate-producing bacteria than oilseed rape (32%) and wheat (31%) (Fig. 1a). Using the microscopic technique, less positive strains were found. However, also with this technique, the highest proportion was found in the rhizosphere of sugar beet. In general, a low correlation between results of both techniques was observed; only 25 isolates showed a correlation between intracellular granules and a positive PCR signal. Furthermore, 66 isolates carried the phaC gene but showed no visible polyhydroxyalkanoate accumulation during cultivation.
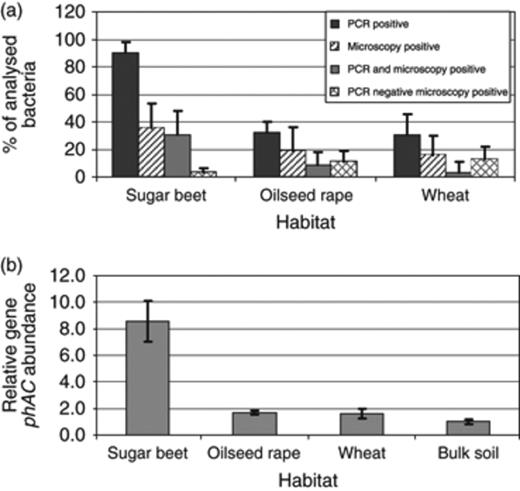
(a) Proportion of polyhydroxyalkanoate-producing bacteria isolated from the rhizosphere of sugar beet, oilseed rape and wheat (Field trial 1). They were screened for their capacity to form polyhydroxyalkanoates using PCR and microscopic methods. Error bars represent confidence intervals at P≤0.05. (b) Relative number of phaC gene copies in the rhizosphere of sugar beet, oilseed rape and wheat in comparison with bulk soil (relative copy number=1) from Field trial 1 determined by qRT-PCR from total genomic DNA. Error bars represent confidence intervals at P≤0.05.
In addition, for profiling and quantifying phaC in plant habitats, two cultivation-independent techniques were applied. The qualitative approach using qRT-PCR resulted in statistically significant differences between the samples from the analysed rhizospheres and those from bulk soil (Fig. 1b), whereas in the rhizosphere of wheat and oilseed rape, the abundance was approximately twofold, the copy number in the sugar beet rhizosphere was >8.5-fold enhanced. Using the qualitative approach, all SSCP profiles of polyhydroxyalkanoate synthesis gene phaC of the rhizosphere community showed a high diversity. The highest diversity was found in the rhizosphere of wheat and the lowest for the oilseed rape rhizosphere. Furthermore, these fingerprints were specific for each plant species. This is shown in Fig. 2 for the SSCP pattern of bacterial communities of oilseed rape, wheat and sugar beet, which form distinct groups according to cluster analysis.
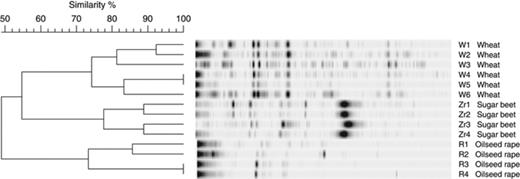
Cluster analysis of community fingerprints of polyhydroxyalkanoate-synthesizing bacteria in the rhizosphere of wheat, sugar beet and oilseed rape using the hierarchical method, unweighted pair group method with arithmetic mean, after calculation of the band-based Dice similarity coefficient. Independently analysed replicates of the treatments were marked 1–4. W, wheat; Z, sugar beet; R, oilseed rape.
Polyhydroxyalkanoate-producing bacteria in the rhizosphere of strawberry and oilseed rape at different stages of plant development and in comparison with bulk soil (Field trial 2)
It is well known that the rhizosphere communities show a strong shift during their plant developmental stages (Smalla et al., 2001). Therefore, in this field trial, the polyhydroxyalkanoate-producing bacterial community was analysed at three different growth stages of strawberry and oilseed rape plants (young, flowering and senescent plants). On comparing both host plants, no significant differences were found in the proportion of polyhydroxyalkanoate-producing bacteria regarding the plant development stage (data not shown). The proportion of positive tested bacteria ranged from 90% (young strawberry plants) to 36% (flowering oilseed rape plants). In this field trial, which was performed at two locations (Rostock and Braunschweig, Germany), the proportion of polyhydroxyalkanoate producers in the rhizosphere was also compared with those in bulk soil. Altogether, at both sites, the proportion of polyhydroxyalkanoate-producing bacteria was statistically and significantly enhanced in the rhizosphere in comparison with bulk soil (Fig. 3).
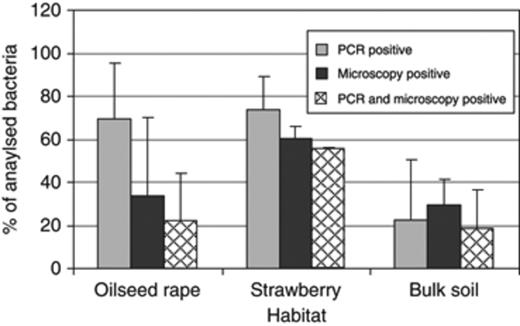
Proportion of polyhydroxyalkanoate-producing bacteria isolated from the rhizosphere of oilseed rape and strawberry in comparison with bulk soil from Braunschweig and Rostock (Germany) (Field trial 2). They were screened for their capacity to form polyhydroxyalkanoates using PCR and microscopic methods. Error bars represent confidence intervals at P≤0.05.
Identification and characterization of polyhydroxyalkanoate-producing bacteria
To identify polyhydroxyalkanoate-producing bacteria, 58 of the strains, which were positive in microscopic as well as molecular evaluation, were first characterized on the genotypic level using BOX-PCR. gelcompar was used for the comparison of BOX patterns. This is exemplarily shown in Fig. 4 for the isolates obtained from the rhizosphere of sugar beet, oilseed rape and wheat from the Deggendorf site (Field trial 1). Altogether, 23 different BOX profiles were obtained. According to the specific profile in BOX pattern, 30 bacterial isolates were selected for further partial sequencing of 16S rRNA genes. This selection and characterization of bacterial polyhydroxyalkanoate producers is shown in Table 1. Altogether, eight bacterial genera (Pseudomonas, Sinorhizobium, Variovorax, Lysobacter, Ensifer, Pantoea, Erwininia and Burkholderia) as well as several species could be identified.
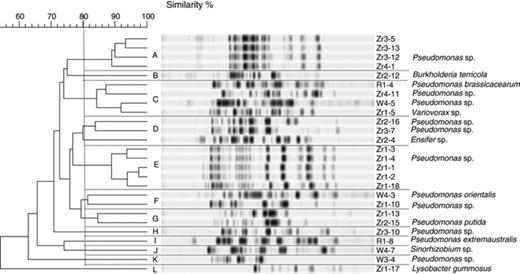
Dendrogram showing the relationship of isolates obtained from the rhizosphere of sugar beet, oilseed rape and wheat from the site in Deggendorf (Field trial 1), based on BOX-PCR fingerprints using cluster analysis using the unweighted pair group method and arithmetic averages.
List of polyhydroxyalkanoate-producing (positive in PCR and microscopy) bacteria isolated from sugar beet, oilseed rape and wheat
BOX group | Isolate no. | Microenvironment | Closest database match (EMBL no.) | % Homology |
C | R1-4 | Rhizosphere oilseed rape | Pseudomonas brassicacearum (FN313510) | 99 |
I | R1-8 | Rhizosphere oilseed rape | Pseudomonas extremaustralis (FN313511) | 99 |
K | W3-4 | Rhizosphere wheat | Pseudomonas sp. (FN313512) | 100 |
F | W4-3 | Rhizosphere wheat | Pseudomonas orientalis (FN313513) | 99 |
C | W4-5 | Rhizosphere wheat | Pseudomonas sp. (FN313514) | 100 |
J | W4-7 | Rhizosphere wheat | Sinorhizobium sp. (FN313515) | 100 |
E | ZR1-4 | Rhizosphere sugar beet | Pseudomonas sp. (FN313516) | 99 |
C | ZR1-5 | Rhizosphere sugar beet | Variovorax sp. (FN313517) | 100 |
F | ZR 1-10 | Rhizosphere sugar beet | Pseudomonas sp. (FN313518) | 100 |
L | ZR 1-17 | Rhizosphere sugar beet | Lysobacter gummosus (FN313519) | 99 |
D | ZR2-4 | Rhizosphere sugar beet | Ensifer sp. (FN313520) | 100 |
B | ZR2-12 | Rhizosphere sugar beet | Burkholderia terricola (FN313521) | 100 |
G | ZR2-15 | Rhizosphere sugar beet | Pseudomonas putida (FN313522) | 100 |
D | ZR2-16 | Rhizosphere sugar beet | Pseudomonas sp. (FN313523) | 98 |
D | ZR3-7 | Rhizosphere sugar beet | Pseudomonas sp. (FN313524) | 98 |
H | ZR3-10 | Rhizosphere sugar beet | Pseudomonas sp. (FN313525) | 99 |
A | ZR3-12 | Rhizosphere sugar beet | Pseudomonas sp. (FN313526) | 100 |
C | ZR4-11 | Rhizosphere sugar beet | Pseudomonas sp. (FN313527) | 98 |
M | LV2-2-10 | Rhizosphere sugar beet | Pseudomonas fluorescens (AJ969079) | 87 |
N | R1-2-4 | Rhizosphere sugar beet | Pseudomonas sp. (FN313528) | 100 |
O | Sel1-2-6 | Rhizosphere sugar beet | Pseudomonas sp. (FN313529) | 99 |
P | At1-1-2 | Rhizosphere sugar beet | Pseudomonas sp. (FN313530) | 100 |
Q | Phst2-1-6 | Rhizosphere sugar beet | Pseudomonas sp. (FN313531) | 99 |
R | At2-2-11 | Rhizosphere sugar beet | Pseudomonas sp. (FN313532) | 99 |
S | SDK1-2-10 | Rhizosphere sugar beet | Pseudomonas sp. (FN313533) | 99 |
S | SDK1-2-16 | Rhizosphere sugar beet | Pseudomonas sp. (FN313534) | 100 |
T | SDK1-2-18 | Rhizosphere sugar beet | Pantoea sp. (FN313535) | 99 |
U | SDK2-2-6 | Rhizosphere sugar beet | Pseudomonas sp. (AJ969083) | 100 |
V | SDK2-2-8 | Rhizosphere sugar beet | Pseudomonas sp. (FN313536) | 100 |
X | RE 1-3-41 | Rhizosphere sugar beet | Erwinia rhapontici (FN313537) | 99 |
BOX group | Isolate no. | Microenvironment | Closest database match (EMBL no.) | % Homology |
C | R1-4 | Rhizosphere oilseed rape | Pseudomonas brassicacearum (FN313510) | 99 |
I | R1-8 | Rhizosphere oilseed rape | Pseudomonas extremaustralis (FN313511) | 99 |
K | W3-4 | Rhizosphere wheat | Pseudomonas sp. (FN313512) | 100 |
F | W4-3 | Rhizosphere wheat | Pseudomonas orientalis (FN313513) | 99 |
C | W4-5 | Rhizosphere wheat | Pseudomonas sp. (FN313514) | 100 |
J | W4-7 | Rhizosphere wheat | Sinorhizobium sp. (FN313515) | 100 |
E | ZR1-4 | Rhizosphere sugar beet | Pseudomonas sp. (FN313516) | 99 |
C | ZR1-5 | Rhizosphere sugar beet | Variovorax sp. (FN313517) | 100 |
F | ZR 1-10 | Rhizosphere sugar beet | Pseudomonas sp. (FN313518) | 100 |
L | ZR 1-17 | Rhizosphere sugar beet | Lysobacter gummosus (FN313519) | 99 |
D | ZR2-4 | Rhizosphere sugar beet | Ensifer sp. (FN313520) | 100 |
B | ZR2-12 | Rhizosphere sugar beet | Burkholderia terricola (FN313521) | 100 |
G | ZR2-15 | Rhizosphere sugar beet | Pseudomonas putida (FN313522) | 100 |
D | ZR2-16 | Rhizosphere sugar beet | Pseudomonas sp. (FN313523) | 98 |
D | ZR3-7 | Rhizosphere sugar beet | Pseudomonas sp. (FN313524) | 98 |
H | ZR3-10 | Rhizosphere sugar beet | Pseudomonas sp. (FN313525) | 99 |
A | ZR3-12 | Rhizosphere sugar beet | Pseudomonas sp. (FN313526) | 100 |
C | ZR4-11 | Rhizosphere sugar beet | Pseudomonas sp. (FN313527) | 98 |
M | LV2-2-10 | Rhizosphere sugar beet | Pseudomonas fluorescens (AJ969079) | 87 |
N | R1-2-4 | Rhizosphere sugar beet | Pseudomonas sp. (FN313528) | 100 |
O | Sel1-2-6 | Rhizosphere sugar beet | Pseudomonas sp. (FN313529) | 99 |
P | At1-1-2 | Rhizosphere sugar beet | Pseudomonas sp. (FN313530) | 100 |
Q | Phst2-1-6 | Rhizosphere sugar beet | Pseudomonas sp. (FN313531) | 99 |
R | At2-2-11 | Rhizosphere sugar beet | Pseudomonas sp. (FN313532) | 99 |
S | SDK1-2-10 | Rhizosphere sugar beet | Pseudomonas sp. (FN313533) | 99 |
S | SDK1-2-16 | Rhizosphere sugar beet | Pseudomonas sp. (FN313534) | 100 |
T | SDK1-2-18 | Rhizosphere sugar beet | Pantoea sp. (FN313535) | 99 |
U | SDK2-2-6 | Rhizosphere sugar beet | Pseudomonas sp. (AJ969083) | 100 |
V | SDK2-2-8 | Rhizosphere sugar beet | Pseudomonas sp. (FN313536) | 100 |
X | RE 1-3-41 | Rhizosphere sugar beet | Erwinia rhapontici (FN313537) | 99 |
Arabic numerals represent the plots (1–4) and the strain number (1–16).
Letters represent the location or microhabitat: R, oilseed rape (Deggendorf, Germany); W, wheat (Deggendorf, Germany); ZR, sugar beet (Deggendorf, Germany); Sel, Seligenstadt, Germany (ZR); At, Attily, France (ZR); Phst, Hilprechtshausen, Germany (ZR); SDK Schoondijke, the Netherlands (ZR); LV, LaVeuve, France (ZR).
List of polyhydroxyalkanoate-producing (positive in PCR and microscopy) bacteria isolated from sugar beet, oilseed rape and wheat
BOX group | Isolate no. | Microenvironment | Closest database match (EMBL no.) | % Homology |
C | R1-4 | Rhizosphere oilseed rape | Pseudomonas brassicacearum (FN313510) | 99 |
I | R1-8 | Rhizosphere oilseed rape | Pseudomonas extremaustralis (FN313511) | 99 |
K | W3-4 | Rhizosphere wheat | Pseudomonas sp. (FN313512) | 100 |
F | W4-3 | Rhizosphere wheat | Pseudomonas orientalis (FN313513) | 99 |
C | W4-5 | Rhizosphere wheat | Pseudomonas sp. (FN313514) | 100 |
J | W4-7 | Rhizosphere wheat | Sinorhizobium sp. (FN313515) | 100 |
E | ZR1-4 | Rhizosphere sugar beet | Pseudomonas sp. (FN313516) | 99 |
C | ZR1-5 | Rhizosphere sugar beet | Variovorax sp. (FN313517) | 100 |
F | ZR 1-10 | Rhizosphere sugar beet | Pseudomonas sp. (FN313518) | 100 |
L | ZR 1-17 | Rhizosphere sugar beet | Lysobacter gummosus (FN313519) | 99 |
D | ZR2-4 | Rhizosphere sugar beet | Ensifer sp. (FN313520) | 100 |
B | ZR2-12 | Rhizosphere sugar beet | Burkholderia terricola (FN313521) | 100 |
G | ZR2-15 | Rhizosphere sugar beet | Pseudomonas putida (FN313522) | 100 |
D | ZR2-16 | Rhizosphere sugar beet | Pseudomonas sp. (FN313523) | 98 |
D | ZR3-7 | Rhizosphere sugar beet | Pseudomonas sp. (FN313524) | 98 |
H | ZR3-10 | Rhizosphere sugar beet | Pseudomonas sp. (FN313525) | 99 |
A | ZR3-12 | Rhizosphere sugar beet | Pseudomonas sp. (FN313526) | 100 |
C | ZR4-11 | Rhizosphere sugar beet | Pseudomonas sp. (FN313527) | 98 |
M | LV2-2-10 | Rhizosphere sugar beet | Pseudomonas fluorescens (AJ969079) | 87 |
N | R1-2-4 | Rhizosphere sugar beet | Pseudomonas sp. (FN313528) | 100 |
O | Sel1-2-6 | Rhizosphere sugar beet | Pseudomonas sp. (FN313529) | 99 |
P | At1-1-2 | Rhizosphere sugar beet | Pseudomonas sp. (FN313530) | 100 |
Q | Phst2-1-6 | Rhizosphere sugar beet | Pseudomonas sp. (FN313531) | 99 |
R | At2-2-11 | Rhizosphere sugar beet | Pseudomonas sp. (FN313532) | 99 |
S | SDK1-2-10 | Rhizosphere sugar beet | Pseudomonas sp. (FN313533) | 99 |
S | SDK1-2-16 | Rhizosphere sugar beet | Pseudomonas sp. (FN313534) | 100 |
T | SDK1-2-18 | Rhizosphere sugar beet | Pantoea sp. (FN313535) | 99 |
U | SDK2-2-6 | Rhizosphere sugar beet | Pseudomonas sp. (AJ969083) | 100 |
V | SDK2-2-8 | Rhizosphere sugar beet | Pseudomonas sp. (FN313536) | 100 |
X | RE 1-3-41 | Rhizosphere sugar beet | Erwinia rhapontici (FN313537) | 99 |
BOX group | Isolate no. | Microenvironment | Closest database match (EMBL no.) | % Homology |
C | R1-4 | Rhizosphere oilseed rape | Pseudomonas brassicacearum (FN313510) | 99 |
I | R1-8 | Rhizosphere oilseed rape | Pseudomonas extremaustralis (FN313511) | 99 |
K | W3-4 | Rhizosphere wheat | Pseudomonas sp. (FN313512) | 100 |
F | W4-3 | Rhizosphere wheat | Pseudomonas orientalis (FN313513) | 99 |
C | W4-5 | Rhizosphere wheat | Pseudomonas sp. (FN313514) | 100 |
J | W4-7 | Rhizosphere wheat | Sinorhizobium sp. (FN313515) | 100 |
E | ZR1-4 | Rhizosphere sugar beet | Pseudomonas sp. (FN313516) | 99 |
C | ZR1-5 | Rhizosphere sugar beet | Variovorax sp. (FN313517) | 100 |
F | ZR 1-10 | Rhizosphere sugar beet | Pseudomonas sp. (FN313518) | 100 |
L | ZR 1-17 | Rhizosphere sugar beet | Lysobacter gummosus (FN313519) | 99 |
D | ZR2-4 | Rhizosphere sugar beet | Ensifer sp. (FN313520) | 100 |
B | ZR2-12 | Rhizosphere sugar beet | Burkholderia terricola (FN313521) | 100 |
G | ZR2-15 | Rhizosphere sugar beet | Pseudomonas putida (FN313522) | 100 |
D | ZR2-16 | Rhizosphere sugar beet | Pseudomonas sp. (FN313523) | 98 |
D | ZR3-7 | Rhizosphere sugar beet | Pseudomonas sp. (FN313524) | 98 |
H | ZR3-10 | Rhizosphere sugar beet | Pseudomonas sp. (FN313525) | 99 |
A | ZR3-12 | Rhizosphere sugar beet | Pseudomonas sp. (FN313526) | 100 |
C | ZR4-11 | Rhizosphere sugar beet | Pseudomonas sp. (FN313527) | 98 |
M | LV2-2-10 | Rhizosphere sugar beet | Pseudomonas fluorescens (AJ969079) | 87 |
N | R1-2-4 | Rhizosphere sugar beet | Pseudomonas sp. (FN313528) | 100 |
O | Sel1-2-6 | Rhizosphere sugar beet | Pseudomonas sp. (FN313529) | 99 |
P | At1-1-2 | Rhizosphere sugar beet | Pseudomonas sp. (FN313530) | 100 |
Q | Phst2-1-6 | Rhizosphere sugar beet | Pseudomonas sp. (FN313531) | 99 |
R | At2-2-11 | Rhizosphere sugar beet | Pseudomonas sp. (FN313532) | 99 |
S | SDK1-2-10 | Rhizosphere sugar beet | Pseudomonas sp. (FN313533) | 99 |
S | SDK1-2-16 | Rhizosphere sugar beet | Pseudomonas sp. (FN313534) | 100 |
T | SDK1-2-18 | Rhizosphere sugar beet | Pantoea sp. (FN313535) | 99 |
U | SDK2-2-6 | Rhizosphere sugar beet | Pseudomonas sp. (AJ969083) | 100 |
V | SDK2-2-8 | Rhizosphere sugar beet | Pseudomonas sp. (FN313536) | 100 |
X | RE 1-3-41 | Rhizosphere sugar beet | Erwinia rhapontici (FN313537) | 99 |
Arabic numerals represent the plots (1–4) and the strain number (1–16).
Letters represent the location or microhabitat: R, oilseed rape (Deggendorf, Germany); W, wheat (Deggendorf, Germany); ZR, sugar beet (Deggendorf, Germany); Sel, Seligenstadt, Germany (ZR); At, Attily, France (ZR); Phst, Hilprechtshausen, Germany (ZR); SDK Schoondijke, the Netherlands (ZR); LV, LaVeuve, France (ZR).
Discussion
In our study, we show that plant habitats, especially the rhizosphere, are an interesting reservoir for polyhydroxyalkanoate-producing bacteria. This was shown by two independent strategies in a hierarchical experimental design. Using the cultivation-independent method SSCP of bacterial phaC genes, a high number of different bands were observed in all rhizosphere samples. In addition, the characterization of isolates results in a high proportion (up to 90%) of polyhydroxybutyrate-producing strains on plants.
In the study, different ecological aspects of polyhydroxyalkanoate-producing bacteria were analysed. Altogether, seven different plant species were included in the investigation. Clear plant-specific effects were found for the rhizosphere community. Root exudates are a key factor for the enrichment of specific microbial populations in the rhizosphere; this was shown using stable isotope probing in combination with microbial fingerprints by Haichar et al. (2008). Exudates consist of ions, free oxygen and water, enzymes mucilage and a diverse array of carbon-containing primary and secondary metabolites (Uren, 2000). Ten to 44% of the photosynthetically fixed carbon is excreted by the root (Bais et al., 2006). Organic acids, sugars, amino acids, lipids, coumarins, flavonoids, proteins, enzymes, aliphatics and aromatics are examples of the primary substances found at the soil–root interface. These substances are not continuously produced, but all of them can be used by bacteria to store the energy in polyhydroxyalkanoate granules.
In general, all applied techniques confirm our conclusions and showed the same tendencies. While the cultivation-independent methods to detect phaC genes in the environment worked well, discrepancies exist between the methods to determine polyhydroxyalkanoate production on the strain level. Here, microscopic techniques including staining and molecular techniques were applied. In general, molecular screening resulted in a higher proportion of positive strains. However, the occurrence of the phaC gene in the genome is not necessarily correlated with the in vitro production. This should be considered in the interpretation of the high percentages obtained. On the other side, the microscopic techniques also have their disadvantages. The medium developed for these investigations is not appropriate for all bacteria. In addition, despite modern microscopes, it is sometimes difficult to detect the granules in the single cells. Because of these problems, we decided to include only those microorganisms into further investigations, which were positive in both approaches. For the molecular approaches to detect polyhydroxyalkanoates in single strains and bacterial communities, different primers were applied. The primer set published by Sheu et al. (2000), which was used for PCR with single isolates, are supposed to target a broad range of phaC genes. However, because of the highly degenerated nature of the sequence, this primer caused unspecific amplicons, when total community DNA was used as template. For this reason, we decided to use a less universal and also less degenerated primer set published by Solaiman et al. (2000) to perform SSCP and qRT-PCR analyses.
This study was carried out to understand the ecological behaviour and reservoirs of polyhydroxyalkanoate-producing bacteria. In addition, new polyhydroxyalkanoate-producing bacterial species were detected: for example, Burkholderia terricola, Lysobacter gummosus, Pseudomonas extremaustralis, Pseudomonas brassicacearum and Pseudomonas orientalis. Our results confirm the hypothesis that the rhizosphere is an interesting and yet unexploited reservoir for polyhydroxyalkanoate producers, and an interesting bioresource not only for plant growth-promoting and antagonistic bacteria.
Acknowledgements
We thank Martin Koller for helpful discussions and Johanna Klostermann (Graz) for mentoring. Research was funded in frame of the fForte-Wissenschafterinnenkolleg ‘FreChe Materie’ by the Austrian Ministries BMVIT, BMWFJ and BM.W_f as well as from the Land Steiermark (Styria).
References
Author notes
Editor: Philippe Lemanceau