-
PDF
- Split View
-
Views
-
Cite
Cite
Marc Troussellier, Claude Courties, Philippe Lebaron, Pierre Servais, Flow cytometric discrimination of bacterial populations in seawater based on SYTO 13 staining of nucleic acids, FEMS Microbiology Ecology, Volume 29, Issue 4, August 1999, Pages 319–330, https://doi.org/10.1111/j.1574-6941.1999.tb00623.x
- Share Icon Share
Abstract
Flow cytometric (FCM) counts of bacteria stained with SYTO 13, a cyanine dye, were highly correlated with DAPI epifluorescence microscopic counts in coastal seawater samples. Fluorescence intensity of stained cells appeared to depend on nucleic acid content and on the polarization of cell membranes. Right angle light scatter values of bacterial populations were clearly related to cell size. By FCM analysis of SYTO 13-stained samples from a batch mesocosm of Mediterranean seawater, several bacterial clusters having different apparent sizes and physiological status were discriminated showing that FCM analysis allows key cell categories to be followed for understanding bacterial community dynamics.
1 Introduction
Applications of flow cytometry (FCM) in marine microbiology are rapidly growing [1]. FCM appeared first as a new way to obtain rapid and accurate estimations of bacterial numbers in aquatic ecosystems, which may supersede classical epifluorescence microscopy. However, until recently the application of FCM to bacterioplankton studies was mainly limited by the use of DAPI or Hoechst, two nucleic acid dyes which need UV excitation rarely available on the most common flow cytometers [2].
The simultaneous development of (i) relatively low-cost bench-top flow cytometers using small argon lasers as light sources and (ii) new generations of high-affinity nucleic acid stains excitable by this specific laser line (488 nm) may lead to the development of efficient protocols in the field of aquatic bacterial ecology.
Among these new dyes, SYTO 13 has been successfully applied to bacterial enumeration in lakes and pure cultures [3,4]. Moreover, SYTO 13 a priori offers the advantages of (i) staining live and fixed cells [4] and (ii) staining both DNA and RNA with similar quantum yields [5]. Therefore, this dye may allow not only enumeration of bacterial cells but also discrimination of cells having different physiological and/or growing status. Using DNA-specific staining protocols, different authors were able to discriminate at least two or three bacterial groups with different apparent DNA contents [2,6]. One may hypothesize that dyes targeting both DNA and RNA sites can have a higher discrimination power leading to larger ‘cytometric diversity’ when applied to natural bacterial communities.
Here, SYTO 13 was validated for flow cytometric enumeration of bacteria in coastal seawater samples and used to discriminate bacterial cell groups within natural communities. Then, we analyzed changes in total bacterial numbers, relative cell size and nucleic acid contents through flow cytometric analysis of SYTO 13-stained samples from a batch mesocosm of Mediterranean seawater. Our results show that several cell groups having different apparent sizes and physiological status can be discriminated. These bacterial cell groups exhibited different temporal behaviors which were related to a variety of functional cellular pools corresponding to (i) small and inactive cells, (ii) more or less large, active and grazing-edible cells, and (iii) large protozoan grazing-resistant cells.
2 Materials and methods
2.1 Comparison of SYTO 13 flow cytometric with DAPI microscopic counts
Mediterranean seawater samples were collected during May–June 1996 in the Banyuls-sur-Mer Bay (42°31′ N, 3°11′ E) and in different coastal areas located near Montpellier (43°25′ to 43°38′ N and 3°38′ to 3°55 E), including beach sites and eutrophic brackish lagoons, to obtain samples with different bacterial densities. Samples were collected 50 cm under the surface in sterile vials, refrigerated and processed within 2 h after collection. The effect of fixation on cell counts, fluorescence and scatter characteristics was tested on some coastal samples. For these samples, aliquots of 1 ml were stained with SYTO 13 (5 μM) before and after fixation with formaldehyde (2% final concentration) for 30 min at room temperature (22±2°C).
2.2 Specificity of SYTO 13 staining
Strain CIP 60.62T of Salmonella typhimurium (Collection Institut Pasteur, Paris, France) was grown at 37°C in trypticase soy broth (TSB) medium (bioMérieux, Marcy-l'Etoile, France). Growth was monitored spectrophotometrically at 600 nm, and 30-ml samples were taken at different stages of the growth curve.
To check DNA staining efficiency, an aliquot (10 ml) of each sample was fixed, for at least 15 min, with 2% (v/v) formaldehyde (final concentration). Fixed cells were incubated (37°C, 60 min) in the presence of RNase A (type 1A, Sigma R-4875) at 400 Kunitz units ml−1 and RNase B (Sigma R-5750) at 500 Kunitz units ml−1[2] or not incubated. Ribonucleases were rendered free of DNase [7]. After RNA digestion, cells were stained with SYTO 13 (5 μM, 15 min) and analyzed by flow cytometry (see below). In an attempt to specifically stain RNA, preliminary experiments were performed using DNase I (type 4, Sigma D-5025) at 200 Kunitz units ml−1 (37°C, 60 min). This DNase treatment resulted in a large fluorescence background and could not be applied alone or combined with RNase prior to staining. Consequently, the efficiency of RNA staining by SYTO 13 was evaluated indirectly: the difference between fluorescence values of SYTO 13-stained cells not treated and treated with RNase, which was expected to be the consequence of RNA staining, was compared to cell rRNA content estimated by fluorescent in situ hybridization (FISH). Cells were hybridized at 46°C for 2 h in 50 μl of hybridization buffer (0.9 M sodium chloride, 20 mM Tris-HCl, 100 μg of polyadenylic acid ml−1, 10% of a Denhardt solution (100×, Sigma), 0.01% sodium dodecyl sulfate, pH 7.2) containing 1 ng μl−1 of oligonucleotide probe (EUB338 or non-EUB338 (negative control), Eurogentec, Seraing, Belgium) labeled at the 5′ end with fluorescein isothiocyanate according to Lebaron et al. [8].
2.3 Mesocosm experiment
To analyze changes in bacterial ‘cytometric diversity’ a mesocosm experiment was carried out during June 1996, with surface water collected in the Banyuls-sur-Mer Bay. The mesocosm (fiberglass rectangular tank; 60 cm high, 60 cm wide, 110 cm long) was filled with 300 l of seawater filtered through a 200-μm nylon mesh to remove large particles (metazoans and detritus). Temperature was controlled (18–19°C) by placing the mesocosm into a larger tank which was continuously fed with natural seawater pumped from the shore. The mesocosm was illuminated by a bank of five fluorescent tubes (36 W, 120 cm) in a 12-h light:12-h dark cycle. The water was continuously kept in circulation with a pump. Samples collected for flow cytometric analyses were immediately processed without fixation, while those collected for protozoa counts were preserved (see Section 2.7).
2.4 Microscopic counts of DAPI-stained bacteria
Subsamples for DAPI counts [9] were fixed (see Section 2.1). After staining with 4′,6-diamidino-2-phenylindole (DAPI; 2.5 μg ml−1, 30 min) and filtering through black polycarbonate membrane filters (0.2 μm pore size, 25 mm, Nuclepore), cells were counted with an Olympus BH-2 epifluorescence microscope (100-W mercury lamp, filter set U, UG-1, DM400, and L420). For each sample, a minimum of 20 fields (≥600 cells) were counted and averaged [10].
2.5 Flow cytometric analysis of SYTO 13-stained bacteria
For flow cytometric analyses, 1-ml subsamples were directly incubated with SYTO 13 at a final concentration of 5 μM for 10–15 min at room temperature in the dark. This dye concentration was chosen from preliminary experiments showing a better discrimination of cells against the fluorescence background than with 2.5 μM, as used for freshwater samples [4]. For each subsample, three replicate counts were performed with a FACSCalibur flow cytometer (Becton Dickinson, San Jose, CA, USA) equipped with an air-cooled argon laser (488 nm, 15 mW). Stained bacterial cells, excited at 488 nm, were enumerated according to their right angle light scatter (RALS) and green fluorescence (FL1) collected at 530/30 nm. These cell parameters were recorded on a four-decade logarithmic scale mapped onto 1024 channels. Before bacterial cells were enumerated, background noise of both water and particles, and electronic noise due to the voltage applied on the green fluorescence detector (450 V) were gated out. First, to eliminate part of the background noise, a threshold of 32 channels was applied to the trigger signal (green fluorescence). Then, a window was drawn around the supposed bacterial cells to exclude the remaining background. On the other hand, in a seawater sample, bacteria coexist with phytoplanktonic cells which are also stained by the dye. Picocyanobacteria, the most numerous autotrophic population detected in the mesocosm experiment, have RALS and FL1 distributions which may overlap those of bacteria. Thus, during each flow cytometric analysis of bacteria, picocyanobacteria were simultaneously detected and enumerated according to their green and orange (phycoerythrin) fluorescence. CellQuest software (Becton Dickinson) was then used to exclude picocyanobacteria from the ‘bacterial window’, and to provide mean values of bacterial cellular parameters.
Fluorescent beads (0.94 μm) (Polysciences Inc., Warrington, PA, USA) were systematically added to each sample. The ratio of mean FL1 or RALS of a cellular population to that of 0.94-μm beads was used to normalize cell fluorescence emission and scatter values among samples.
The precise volume analyzed and subsequent estimations of cell concentrations were calculated by measuring the remaining volume and subtracting it from the initial subsample volume (1 ml). Since part of the measured volume was used for flushing the sample line, the outer sleeve of the sample injection port of the flow cytometer was removed.
In order to calibrate the flow cytometric scatter measurements, bacterial cell sizes were estimated from epifluorescence examination (at 1250× magnification) of DAPI-stained cells (see above) of some fixed samples. Randomly selected cells (100 cells) were classified into length and width classes (0.5-μm class intervals up to 3 μm, 1-μm class intervals between 3 and 10 μm, and 5-μm class intervals above 10 μm) using an eyepiece graticule. Cell volume was calculated assuming that the shape of bacteria was spherical or cylindrical with hemispheric ends [11]: V=(πw2/4) (l−w/3), where w is the width and l is the length of the cell.
Several bacterial cell populations were discriminated from RALS and FL1 cytograms obtained for each sample (see Section 3). From one sample to another, these populations were not exactly located at the same place on cytograms. Thus, in order to objectively group the different populations observed in all analyzed samples, a clustering method (Ward's algorithm [12]) was applied on all the identified populations. Each population was characterized by its RALS and FL1 mean values. Clustering was carried out with the ‘R package’[13].
2.6 Phytoplankton
Using unstained subsamples, phytoplanktonic cells, excited at 488 nm, were detected and enumerated by FCM according to their RALS and their orange (585/42 nm) and red fluorescence (>650 nm) emissions related to phycoerythrin and chlorophyll pigments, respectively. Cell concentrations were calculated as for bacteria (see Section 2.4 above).
2.7 Protozoa
The abundance of protozoa was determined by epifluorescence microscopy (Leitz, Laborlux D) after DAPI staining. Water samples (20 ml) were preserved with glutaraldehyde (0.5% final concentration) and stained with DAPI (1 μg ml−1, final concentration) for 15 min. Stained protists were collected by filtration on 0.8-μm Nuclepore black filters. Filters were mounted on microscope slides and stored at +4°C until examination. Pico-sized (<2 μm in diameter) and nano-sized (2–20 μm in diameter) microorganisms were identified, counted and measured at a magnification of 1250× and 625×, respectively, while micro-sized (20–200 μm in diameter) microorganisms were analyzed at a magnification of 125×. A minimum of 100 organisms per filter were counted. Autotrophic species were distinguished from heterotrophs by the red autofluorescence of chlorophyll a observed under blue light excitation [14]. Data presented in this paper only concern heterotrophic protozoa.
3 Results
3.1 Comparison of SYTO 13 and DAPI counts
FCM analysis of SYTO 13-stained samples resulted in a clear discrimination of bacterial cells from the fluorescent background even in the case of fixed samples (Fig. 1). FCM enumeration of SYTO 13-stained bacteria from unfixed coastal seawater samples was compared to DAPI epifluorescence counts (EFM) (n=33, Fig. 2). The relationship between the two counting methods was highly significant (R2=0.918, P≤0.0001). The intercept (b) of the linear model (EFM counts=a FCM counts+b) was not significantly different from zero (P=0.4027), and the slope (a) was not significantly different from 1 (a=1.049, P≤0.0001). A similar relationship was found between cytometric counts of fixed and nonfixed samples (R2=0.908, P≤0.0001; b∼0, P=0.799; a∼1, P≤0.0001; n=15) (data not shown).
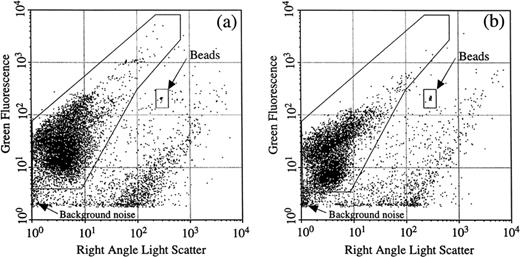
Flow cytometric analysis of fresh (a) and fixed (b) (formaldehyde, 2% final concentration) subsamples of coastal seawater stained with SYTO 13. The x and y axes show right angle light scatter (RALS) and green fluorescence (FL1) values (arbitrary units), respectively. Large windows on the left were drawn around fluorescent bacterial cells. Fluorescent microbeads (0.94-μm diameter) (small windows, on the upper right) were used as internal standard. Signal processing was triggered on green fluorescence (FL1).
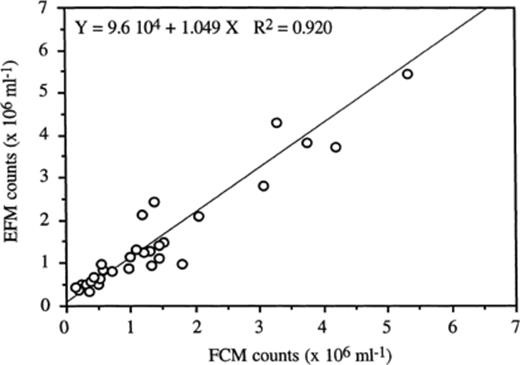
Relationship between microscopic counts of DAPI-stained bacterial cells (EFM) vs. flow cytometric counts of SYTO 13-stained cells (FCM) from 33 samples of coastal Mediterranean seawaters.
3.2 Fluorescence and light scattering of fixed and unfixed cells
Generally, unfixed bacterial cells showed a brighter fluorescence than fixed cells (ratio>1; Fig. 3a). The shift in fluorescence was not the same from one sample to another and there was no significant correlation between fluorescence of unfixed and fixed cells. Compared to FL1, RALS showed limited changes in fixed relative to unfixed samples (ratio∼1; Fig. 3b).
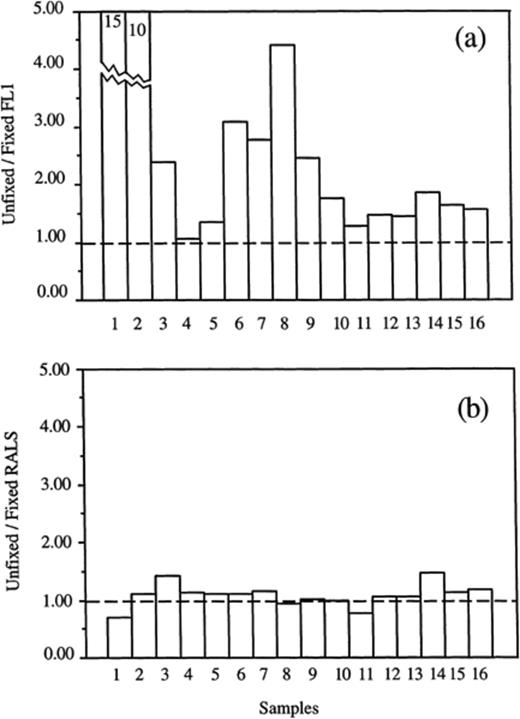
Effects of fixation (formaldehyde, 2% final concentration) on mean fluorescence (FL1) and RALS of bacterial cells from 16 samples of coastal Mediterranean seawaters. Results are plotted as the ratio of FL1 (a) or RALS (b) values for unfixed and fixed subsamples.
3.3 Specificity of SYTO 13 for fixed cells of S. typhimurium
Fluorescence of S. typhimurium cells treated (SST) or not (SSNT) with RNases and stained with SYTO 13, or obtained with the FISH technique, at different stages of the growth curve are presented in Table 1. We noted that cells which contain RNA (SSNT) showed a higher fluorescence than cells treated with RNases (SST) and that all the different fluorescence values increased at the beginning of the exponential phase and declined toward stationary phase. Taking into account all the analyzed samples during the growth experiment (n=12), a highly significant correlation (r=0.959; P≤0.0001) was obtained between SST and SSNT fluorescence. A significant correlation (r=0.875; P=0.0002; n=12) was also found between the fluorescence values of cells hybridized with the FISH technique and those obtained by computing the difference between SSNT and SST fluorescences.
Fluorescence values from nucleic acids of S. typhimurium cells at different stages of the growth curve
Growth phase | Time (h) | OD | SSNT | SST | FISH |
Inoculum | 0 | 0.056 | 0.121 | 0.054 | 0.0017 |
Exponential | 1 | 0.098 | 0.614 | 0.112 | 0.0064 |
Exponential | 3 | 0.626 | 0.197 | 0.065 | 0.0038 |
Stationary | 6 | 1.290 | 0.074 | 0.039 | 0.0006 |
Growth phase | Time (h) | OD | SSNT | SST | FISH |
Inoculum | 0 | 0.056 | 0.121 | 0.054 | 0.0017 |
Exponential | 1 | 0.098 | 0.614 | 0.112 | 0.0064 |
Exponential | 3 | 0.626 | 0.197 | 0.065 | 0.0038 |
Stationary | 6 | 1.290 | 0.074 | 0.039 | 0.0006 |
OD: optical density; SSNT and SST: fluorescence of SYTO 13-stained cells not treated and treated by RNase, respectively; FISH: fluorescence of cells hybridized with the FISH technique.
Fluorescence values from nucleic acids of S. typhimurium cells at different stages of the growth curve
Growth phase | Time (h) | OD | SSNT | SST | FISH |
Inoculum | 0 | 0.056 | 0.121 | 0.054 | 0.0017 |
Exponential | 1 | 0.098 | 0.614 | 0.112 | 0.0064 |
Exponential | 3 | 0.626 | 0.197 | 0.065 | 0.0038 |
Stationary | 6 | 1.290 | 0.074 | 0.039 | 0.0006 |
Growth phase | Time (h) | OD | SSNT | SST | FISH |
Inoculum | 0 | 0.056 | 0.121 | 0.054 | 0.0017 |
Exponential | 1 | 0.098 | 0.614 | 0.112 | 0.0064 |
Exponential | 3 | 0.626 | 0.197 | 0.065 | 0.0038 |
Stationary | 6 | 1.290 | 0.074 | 0.039 | 0.0006 |
OD: optical density; SSNT and SST: fluorescence of SYTO 13-stained cells not treated and treated by RNase, respectively; FISH: fluorescence of cells hybridized with the FISH technique.
3.4 Relationship of scatter intensity and cell size


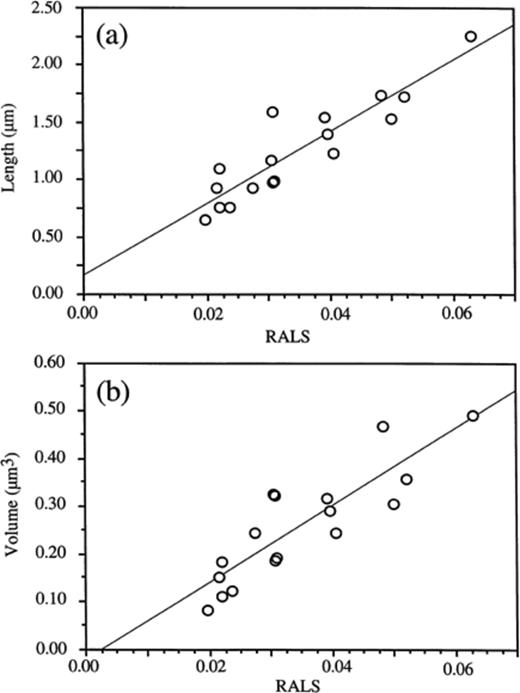
Relationship between mean standardized RALS values and mean cell length (a) or volume (b).
The 95% confidence intervals (lower and upper) for intercepts in Eqs. 1 and 2 (−0.116, +0.457 and −0.113, +0.073, respectively) showed that these intercepts were not significantly different from zero.
3.5 Discrimination of bacterial populations in seawater samples
SYTO 13 staining was applied to unfixed samples from a mesocosm experiment using Mediterranean seawater. Total phytoplanktonic abundance decreased during the experiment (Fig. 5a), mainly as the result of the disappearance of picocyanobacteria observed through phytoplanktonic FCM analysis (data not shown). During the course of incubation, flow cytometric bacterial total counts showed first a slow but regular increase (∼3-fold in 3–4 days), followed by a rapid decrease (Fig. 5a). Then, after bacterial cell abundances reached a maximum value, protozoa counts increased sharply and decreased when bacterial cell numbers fell to their initial value or below.
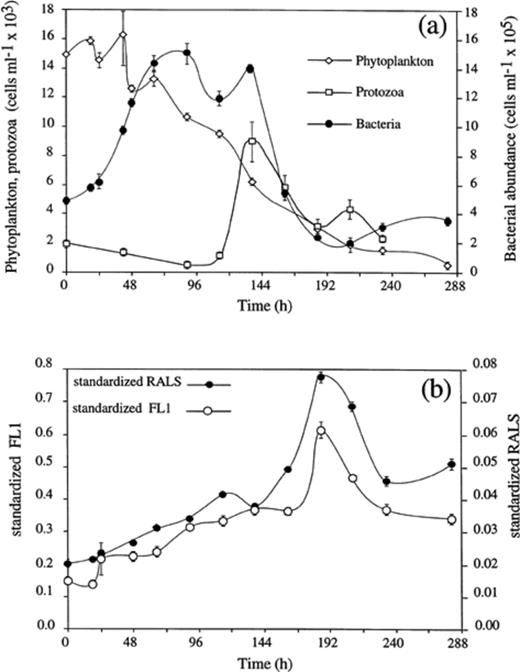
Changes in phytoplanktonic, protozoan, and bacterial abundances during batch incubation of Mediterranean seawater (a). Mean standardized RALS and FL1 values for bacterial cells in the same batch incubation are also reported (b). Error bars are standard deviations (n=3).
Mean RALS and FL1 values of bacterial cells increased during growth, reached maximum values after the shift in bacterial numbers, and then showed a limited decrease (Fig. 5b).
Combining RALS and FL1 signals, different cell populations were detected (Fig. 6). The overall trend was an increase in the proportion of cells with large RALS and FL1 values. The hierarchical clustering method allowed the identification of seven main clusters with different FL1 and RALS mean values (Fig. 7). A strong linear relationship between FL1 and RALS mean values of the different clusters was found (r=0.993, P<0.0001). The slope (a=7.21) clearly indicates that the range of variation of FL1 was larger than that of RALS. From the linear regression model established between RALS and length (Eq. 1), estimates of mean cell length among FCM bacterial populations ranged from 0.32 μm to 15 μm.
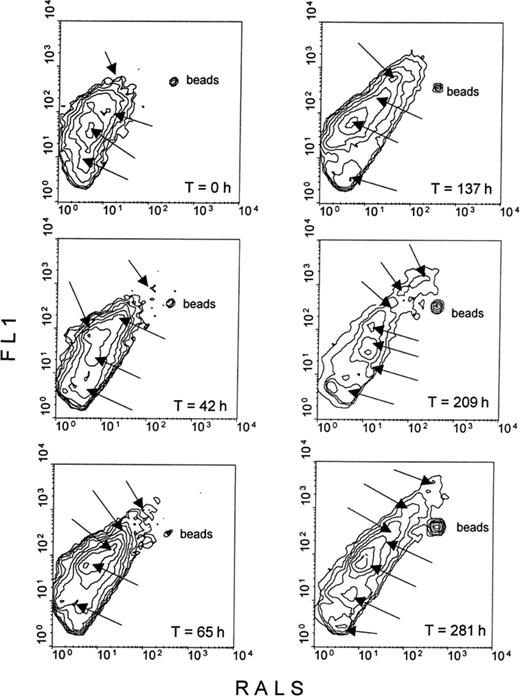
Flow cytometric analysis of seawater samples collected at different times during batch incubation of Mediterranean seawater, and stained with SYTO 13. Successive contours from outer to inner levels correspond to bacterial cell abundance increase. Arrows indicate the different bacterial populations that were discriminated. Fluorescent microbeads (0.94-μm diameter) (small spot, on the upper right) were added as internal standard.
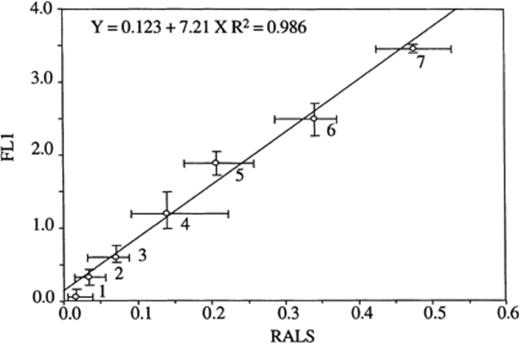
Clusters of bacterial populations obtained from hierarchical clustering. Each cluster is reported according to its mean standardized RALS and FL1 values (○). The maximum and minimum RALS and FL1 mean values observed among the different cell populations within each cluster are also indicated by horizontal and vertical bars. Numbers associated with clusters correspond to cluster numbers used in the text.
In initial seawater samples, the dominant cluster (cluster 1, 75% of total counts, Fig. 8a) exhibited low RALS and FL1 values (Fig. 7). Two other clusters (clusters 2 (22%) and 4 (2%)) had larger RALS and FL1 values (Fig. 7). During seawater incubation, four additional clusters were detected (Fig. 8a). Clusters 4–7 exhibited high RALS and FL1 values (Fig. 7) and low frequencies, and were lumped together for simplification.
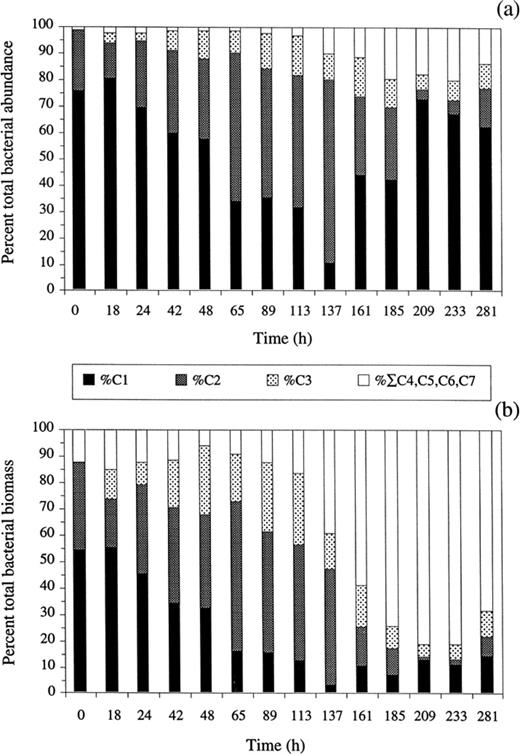
Proportions (%) of the different clusters in total populations during batch incubation of Mediterranean seawater. Proportions were computed with reference either to total number (a) or to apparent biomass (b) (see text for explanation).
Computation of the correlation among the abundances of each cluster and total counts showed that changes in total abundances were significantly correlated with changes in clusters 2 (r=0.945, P≤0.0001) and 3 (r=0.902, P≤0.0001), less correlated with cluster 1 (r=0.593, P≤0.01), and not at all with the group of clusters 4, 5, 6 and 7. Thus, global changes in bacterial community abundances were mainly the consequence of changes in clusters 2 and 3 which have higher RALS and FL1 values than the initial dominant cluster. The apparent net increase rates of clusters 2 and 3 computed during their increase phase were not significantly different (∼0.046 h−1). They were clearly higher than the apparent net increase rate of the sum of all the different clusters (∼0.020 h−1).
4 Discussion
FCM counts of SYTO 13-stained bacteria in unfixed seawater samples were not significantly different from those obtained with fixed samples by the traditional DAPI microscopic counting method (Fig. 2). Similar results were reported for lake waters by del Giorgio et al. [4]. These authors also showed a shift in the fluorescence emission of fixed relative to unfixed bacterial cells from lake samples (by an average of −67%) similar to that reported in this study for seawater samples (average of −50%). Opposite results, i.e. an increase of the fluorescence of fixed relative to unfixed cells, have been obtained with DAPI-stained bacteria from coastal bacterioplankton [15]. This difference can be explained by differences in electro-chemical properties of the dyes. SYTO 13, like all cyanine dyes, has a net positive charge [5] and is attracted by negatively charged nucleic acids. The penetration of cyanine dyes in living cells is facilitated by the transmembrane electrochemical potential [16], and this property is often used for viability measurement [16–18] Thus, the attraction of SYTO 13 may be reduced in fixed cells with depolarized membranes leading to a lower fluorescence intensity of stained cells. This decrease was not expected for an uncharged dye such as DAPI.
SYTO 13 staining of fixed Salmonella cells was shown to be sensitive and dependent on both DNA and RNA contents of bacterial cells. Thus, fluorescence of SYTO 13-stained cells may be related to both DNA and RNA contents of fixed bacterial cells, and also to membrane polarization when applied to live cells. These cellular characteristics have been shown to be affected in different ways when bacteria were subjected to adverse conditions such as nutrient starvation [19,20]. Consequently, the potential range of fluorescence values of SYTO 13-stained cells may be larger than when these cells were stained with a DNA-specific fluorochrome, leading to an easier discrimination of cells with different physiological states within natural communities.
RALS values were less affected by fixation than fluorescence values (Fig. 3). Thus, size estimations from RALS measurements may be obtained from unfixed or fixed samples, provided that calibration is realized through comparison of RALS and size values. Calibration results obtained in this study should only be considered estimations, because (i) the method used to determine cell size (eyepiece graticule) is not very precise, and (ii) the reported relationships between cell size and RALS were based on a limited number of sized cells (n=100) when compared to the large number of cells (20 000–40 000) from which the mean RALS values were computed. However, because of this large number of cells that can be characterized by FCM for a given sample, we can obtain more accurate estimations of the distribution of cell size (or other cell characteristics) in bacterial communities.
On the basis of simultaneous measurements of light scattering and fluorescence of cells stained with other nucleic acid stains, it was not possible to discriminate more than two or three groups of bacterial cells [2,6]. These results were obtained using DNA-specific staining. In this study, using SYTO 13 staining of unfixed samples from Mediterranean seawater stored in a mesocosm, it was possible to discriminate a larger number of bacterial cell clusters and to follow their dynamics (Fig. 8). This higher discrimination power was likely due to the binding affinity of SYTO 13 for both DNA and RNA and to its sensitivity to polarized membranes. Interestingly, we observed a strong relationship between mean FL1 and RALS values of the different discriminated clusters (Fig. 7). So, considering that FL1 changes were proportional to physiological changes, and RALS to cell size, this relationship may be seen as an additional support to Stevenson's hypothesis that small bacteria are dormant while larger bacteria are more likely to be active [21].
Bacterial cells with an apparent low nucleic acid content and small relative sizes (cluster 1) showed very limited changes in numbers. Although we cannot exclude a perfect matching between growth and grazing processes, and thus a ‘hidden’ dynamics of this cell group, it is more probable that this result indicates a limited role of small bacteria in bacterioplankton dynamics as already reported in the literature [22–24]. At the same time, cells with higher nucleic acid contents and sizes (clusters 2 and 3) exhibited strong dynamics. During the increase in total cell numbers, their apparent net increase rates (0.04–0.05 h−1) were not significantly different, and are quite similar to the apparent specific growth rates observed by Gasol et al. [21] for the largest bacterial cell size classes in a northwestern Mediterranean bay (Bay of Blanes). Bacteria belonging to these clusters also showed the highest disappearance rate, suggesting a strong grazing pressure on this fraction of bacterial cells. The behavior of these cells is consistent with the process of selective removal of active cells by planktonic grazers described by del Giorgio et al. [25].
A third kind of bacterial cell group, with very large FL1 and RALS values (clusters 4, 5, 6 and 7), was also detected. These cells appeared in low numbers when total numbers reached the maximum values. We hypothesized that these clusters correspond to the occurrence of large grazing-resistant bacteria [26]. Their low numbers do not preclude that they may represent a significant part of the total bacterial biomass. We tried to evaluate this point through comparison of apparent bacterial biomass estimates deduced from abundance of each cluster multiplied by their respective mean RALS value (ni RALSi) for cluster 1, joined clusters 2 and 3 (n2 RALS2+n3 RALS3) and 4, 5, 6 and 7 (n4 RALS4+n5 RALS5+n6 RALS6+n7 RALS7). Changes in these apparent biomasses are illustrated in Fig. 8b. Clearly, clusters 6 and 7, which do not contribute to the initial biomass, increased in their relative contribution to represent more than 50% of the total biomass after the increase of grazing pressure on the bacterial community.
In conclusion, FCM analysis of bacterial communities stained with SYTO 13 allows a rapid and easy enumeration of bacterial cells in aquatic samples. Recently, other new nucleic acid-specific dyes have been shown to be well suited for the enumeration of bacterioplankton in natural aquatic ecosystems [8]. Some of these new dyes (SYTO 9, SYBR-II) yielded higher fluorescence signals than SYTO 13 [8], which may help to better discriminate bacterial cells from fluorescence noise. However, we have shown that, using SYTO 13, it was possible to discriminate and to follow the dynamics of the different bacterial clusters which may be considered key cellular categories in understanding bacterial community dynamics: small inactive cells, larger active and grazer-edible cells, and grazing-resistant cells. Because of the large number of cells that FCM can characterize, this method is well suited to obtain accurate measurements of these different bacterial groups. However, these different groups, discriminated on the basis of their apparent size and nucleic acids content, might represent either the same or different bacterial species. A further step could be to use cell sorting to analyze some morphological, physiological or phylogenetic characteristics of these bacterial populations.
Acknowledgements
This study was supported by Contract MAS3-CT96-0047 from the European Community (ELOISE project).
References