-
PDF
- Split View
-
Views
-
Cite
Cite
M. Esther Puente, Gina Holguin, Bernard R. Glick, Yoav Bashan, Root-surface colonization of black mangrove seedlings by Azospirillum halopraeferens and Azospirillum brasilense in seawater, FEMS Microbiology Ecology, Volume 29, Issue 3, July 1999, Pages 283–292, https://doi.org/10.1111/j.1574-6941.1999.tb00619.x
- Share Icon Share
Abstract
Inoculation of axenic black mangrove seedlings in seawater for 8 days with either the terrestrial halotolerant plant growth-promoting bacterium Azospirillum halopraeferens or with Azospirillum brasilense produced heavy colonization of the root surface. The colonization pattern was different for the two strains. A. halopraeferens yielded mainly single cells embedded in a thick sheath, whereas A. brasilense produced primarily microaggregates. A. brasilense cells were anchored to the root surfaces and to themselves by a network of fibrillar material. Both bacterial strains survived in seawater (approximately 104 colony forming units per ml) for more than 30 days, for 70 days in saline water (A. brasilense) and colonized mangrove roots at a very high population density. A. halopraeferens was a better root surface colonizer, whereas the A. brasilense population was greater in the entire root. This work is the initial stage of studies designed to assess the feasibility of using terrestrial plant growth-promoting bacteria for the inoculation of marine plants.
1 Introduction
A variety of methods have been used to study root colonization by Azospirillum sp. including bacterial count methods, scanning and transmission electron microscopy [1–3], coupled with gold labelling [4], light microscopy aided by immunofluorescence [5], the use of dyes [6], the GUS-reporter system [7] and confocal laser microscopy used with fluorescent oligonucleotides [8] and other molecular methods [9]. All of these studies were done on terrestrial plant roots under nonosmotic stress conditions for the added bacterium using fresh water for the growing plants, while no studies have been performed in the presence of a high concentration of salt (i.e. > 1%).
Mangroves are tropical and subtropical trees that are the major component of coastal marine-lagoon ecosystems and serve as feeding, spawning and reproductive areas for numerous economically and ecologically important marine species [10]. In the rainy tropics, mangroves generally self-revegetate after clearcutting. In some instances, revegetation is aided by the propagules sown by forest management personnel [11]. However, in semi-arid tropics, following clearcutting, mangroves hardly ever revegetate [12]. There, the plants must first overcome a salt stress that inhibits seedling establishment [12]. Mangroves in semi-arid tropics are considered to be nutrient deficient ecosystems because they contain very low levels of nitrogen and phosphorus ([13], Vazquez, Holguin, Puente, Lopez-Cortes and Bashan, 1998, submitted, Biol. Festil Soils). This apparent nutrient deficiency notwithstanding, these ecosystems flourish with no obvious signs of nutrient deficiency. Nitrogen fixation in mangrove sediments, in the rhizosphere [13,14] and associated with aerial roots [15,16] may provide the nitrogen necessary for plant growth, and phosphate-solubilizing microorganisms may supply plants with a sufficient amount of phosphorus [17]. The bacterial species that facilitate nitrogen fixation and phosphate solubilization in association with mangroves in semi-arid tropical regions of the world are not well-characterized, although some of the organisms that are involved in these processes have been identified [13,16,18], Vazquez et al., 1998 submitted, Biol. Festil Soils). Other bacteria that might enhance the plant growth by mechanisms other than nitrogen fixation and phosphorus solubilization, such as those organisms that have been shown to promote the growth of terrestrial crop plants [19–22], are unknown in this ecosystem.
It was previously observed that mangrove seedlings usually grow better after inoculation with the diazotrophic filamentous cyanobacteria Microcoleus chthonoplastes [15,23]. Based on this observation, it was reasoned that mangrove seedlings might also benefit by being inoculated with plant growth-promoting bacteria (PGPB) [24]. PGPBs have been reported to stimulate revegetation of temperate forests [22,23,25,26]. Azospirillum species are well-known PGPBs that facilitate the growth of a wide range of terrestrial plant species [19,27]. Of the five species of Azospirillum, one species, A. halopraeferens, is halotolerant (3% salt, [28]) and the other four species exhibit varying levels of tolerance to salt. Besides A. halopraeferens, A. brasilense has the highest salt tolerance of the azospirilla [29]. A. brasilense can tolerate 2% NaCl [30]. Its salt tolerance is regulated by the intracellular accumulation of osmolytes [31,32]. Both A. brasilense and A. lipoferum have been isolated from seawater-impacted soils [33], saline calcareous soil [34], saline paddy soils [35], mangrove leaves [36] and seaweeds (H.C. Derx, unpublished, 1949, cited in [37]). To the best of our knowledge, there are no literature reports describing the inoculation of Azospirillum onto marine plants or into seawater [19,27] where tropical seawater contains approximately 3.3% salt. The present study is an initial step in the deliberate establishment of an association between a terrestrial PGPB and a marine tree, assessing the ability of two salt tolerant Azospirillum strains to survive and to colonize mangrove roots in seawater.
2 Materials and methods
2.1 Bacteria, plants and growth conditions
A. brasilense Cd (DSM 7030, Braunschweig, Germany) and A. halopraeferens AU10 (kindly provided by B. Reinhold-Hurek, Max-Planck Institute, Marburg, Germany) were cultured by the standard methods for these species using N-free OAB medium for cultivation of A. brasilense Cd and OAB supplemented with 1% NaCl for A. halopraeferens AU10 [28,38]. They were prepared for inoculation at the optimal concentration of 106 colony forming units (cfu) ml−1 as previously described [39], but in filtered sterile seawater.
Bacterial survival studies were conducted by incubating 5.2×108 cfu ml−1A. brasilense and 4.57×109 cfu ml−1A. halopraeferens in seawater in the absence of any plant material. High bacterial numbers were used to avoid that the entire Azospirillum populations will disappear during long incubation periods. Preliminary observations showed a high mortality rate of both Azospirillum species in seawater. Seawater was obtained from the experimental aquaculture facility of CIB (La Paz, Mexico). Briefly, tropical seawater was pumped from the sea into a large cistern and later pumped into a large sedimentation tank to eliminate denser particles. The seawater was filtered through a 5-mm mesh filter and, while flowing at a very low speed, subjected to 40 W of UV irradiation using Life Gard lamps (Rainbow Plastics Filter Division, El Monte, CA). This procedure eliminates most sea-dwelling organisms. Finally, the seawater was sterilized by standard autoclaving, with no salt precipitation. The seawater used contained only traces of organic matter and N, P and K ([13], Vazquez et al. 1998, submitted, FEMS Microbiol. Ecol.). Similarly, A. brasilense Cd was incubated in sterile saline water (4633 μmos cm−2, containing no detectable NPK) obtained from a well in the CIB campus.
Black mangrove seedlings (Avicennia germinans (L.) Stern) were axenically grown from propagules collected from the wild in September 1996 and inoculated with Azospirillum strains in flasks containing seawater as previously described for sand cultures of filamentous cyanobacteria [23]. Control seedlings were treated identically but were not inoculated. Precautions described earlier [23] were taken to ensure the axenity of the mangrove culture before and throughout the study because the strains did not have electron microscopy markers. To ensure contamination-free cultures during sampling periods, uninoculated control plants were sampled at each sampling time. The seedlings were macerated by a tissue homogenizer (described later) and the macerate was plated on Nutrient Agar (Difco) plates. At no sampling time was any contaminant of the inoculated Azospirillum species detected. The two species of Azospirillum were re-isolated routinely during the entire study and their identity was confirmed by a comparison to the original strains.
2.2 Bacterial counts
A. brasilense cells were counted by the Plate-Count method on Nutrient Agar [38] (Difco, Detroit, MI, USA) and A. halopraeferens on Nutrient Agar supplemented with 1% NaCl because it has a growth requirement for NaCl [28]. Roots were macerated by placing 5 mm of root-tip segments into 5 ml 0.85% NaCl and homogenizing the suspension at 4000 rpm for 30 s at 26±2°C in a tissue homogenizer (Polytron Brinkmann Instruments, NY, USA) prior to determining the number of bacterial cells. The remaining macerated root tissue was filtered through Whatmann no. 1 filter paper for a dry weight (dw) determination. Since a significant proportion of the bacteria that were examined were embedded in a sheath layer on the root surfaces (see Section 3), the Plate-Count method could not differentiate between colonies originating from single cells or from small aggregates of cells. Therefore, the bacterial populations that were measured should be considered as minimum population levels.
2.3 Scanning electron microscopy (SEM)
Samples of root tissue were excised from test plants for 8 consecutive days and prepared for SEM observation as follows. The roots were fixed with 2.5% glutaraldehyde (Sigma, St. Louis, MO, USA) in 0.2 M cacodylate buffer, pH 7.2, for 2 h at 28°C under vacuum of 760 mm Hg. The samples were rinsed once in the same buffer. Then, the roots were dehydrated by passage through increasing concentrations of ethanol in water. The final wash was in 100% acetone. The samples were dried in a critical point dryer (Denton, DCP-1, Cherry Hill, NJ, USA) in a CO2 atmosphere. The dried samples were affixed to stubs with conductive, self-sticking adhesive tabs and coated with 30 nm gold film (Polarun, Watford, UK) before being examined by SEM (Hitachi S-570, Japan) at 60 kV.
2.4 Experimental design and statistical analysis
The entire experiment was replicated five times. For every SEM sample, five root segments were taken from the 5-mm tip of each root. For measurements of the survival of bacteria in seawater, five replicates were used. The results were analyzed by One-way Analysis of Variance (ANOVA) and by Student's t-test at P≤0.05. Mortality graph fitting was done by CurveExpert (Freeman-Teresa Software, Clemson, SC, USA).
3 Results and discussion
Because mangroves are being deforested on an alarming scale [40], it is imperative to consider strategies for their preservation and reforestation. Especially in semi-arid regions of the world where mangroves do not efficiently reforest themselves, artificial reforestation might be a possible solution. As a starting point, it is assumed that mangrove seedlings might benefit from artificial inoculation with PGPB as do many other plant species [19,41–43]. Because the possible beneficial features of the native microflora of semi-arid mangroves are unknown, we propose, as a first step, establishing a mangrove-PGPB association using well-known, non-specific, salt tolerant Azospirillum strains.
Incubation of A. halopraeferens in sterile seawater resulted in a continuous decrease in the bacterial population over a period of 6 days. The population then stabilized at a level of at least 104 cfu ml−1 for up to 20 days (Fig. 1b). Under similar incubation conditions, the mortality curve of A. brasilense showed a comparable but more drastic decrease in the population after 1 day in seawater. After an additional small decrease in the bacterial count, the population eventually stabilized after 5 days at a level of approximately 6×104 cfu ml−1 for up to 30 days (Fig. 1a). A similar pattern occurred when A. brasilense was incubated in saline water, but the population survived for 70 days (Fig. 1c).
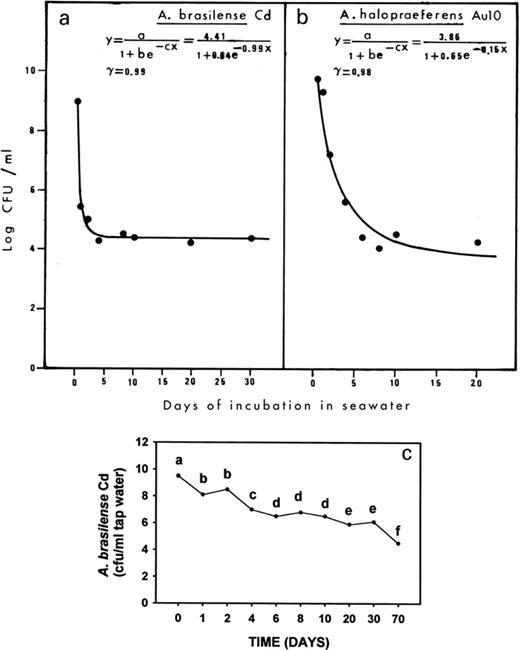
a, b: Mortality curves of A. halopraeferens AU10 and A. brasilense Cd in seawater. The significance of the curve is represented by r. c: Mortality of A. brasilense Cd in saline water. Points denoted with a different letter differ significantly at P≤0.05 using one-way ANOVA.
Hartmann et al. [44] showed that natural selection of salt tolerant natural variants or mutants of A. brasilense Sp-7 (a strain almost identical to strain Cd used in this study) occurred upon salt stress. Obviously, the surviving populations of over 104 cfu ml−1 seawater for both Azospirillum species are resistant to the salt stress. However, it is as yet unknown whether this resulted from a physiological adaptation of the population to salt or represents a selection of a more salt tolerant subpopulation. Other studies have demonstrated that when A. brasilense Cd and A. lipoferum DSM 1842 were incubated in distilled water, no decrease in their population occurred after 8 days (Castellanos, Ascencio, Bashan, 1998, unpublished). In N-free semi-solid medium containing 3% NaCl, A. halopraeferens was grown and survived for 7 days [28]. Transfer of OAB-cultivated A. brasilense Cd into OAB medium supplemented with 2% NaCl did not affect the population level for 4 days [30].
A different survival pattern was observed when bacteria were inoculated onto the roots of mangrove plants in seawater. In this case, both strains survived well on roots. Their populations reached high numbers (i.e. approximately 6×109 cfu g−1 root dw) after 4 days (Fig. 2). Although a small decrease in the population occurred with the time, population levels remained at about 108 cfu g−1 dw for 8 days. This is in comparison to colonization levels of about 104 cfu g−1 roots of wheat [45] or about 106 cfu g−1 roots for several vegetables [42]. Generally, the total number of bacteria colonizing the root was greater for A. brasilense than for A. halopraeferens (Fig. 2). Concomitantly, colonization patterns of both Azospirillum strains were monitored daily by SEM. Because the cultures were axenic, no other microorganisms were found on the surface of the roots during the course of the experiment (Fig. 3a, b).
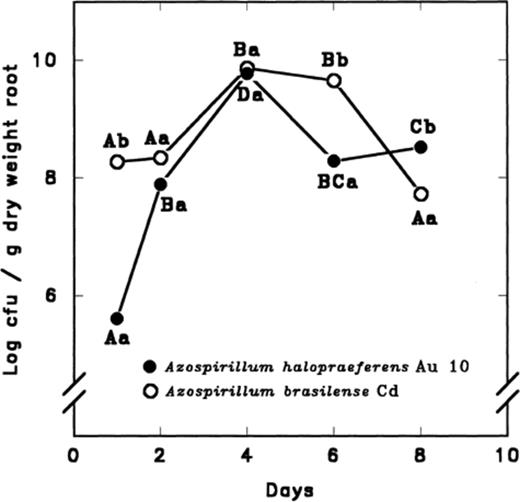
Colonization of black mangrove seedling roots by A. halopraeferens AU10 and A. brasilense Cd in seawater. Each point on each curve, separately, that is denoted by a different letter differs significantly at P≤0.05 using one-way ANOVA. Different lower case letters indicate a significant difference at P≤0.05 between the bacterial species at each sampling time using the Student's t-test. Initial inoculum was 106 cfu ml−1 for each strain.
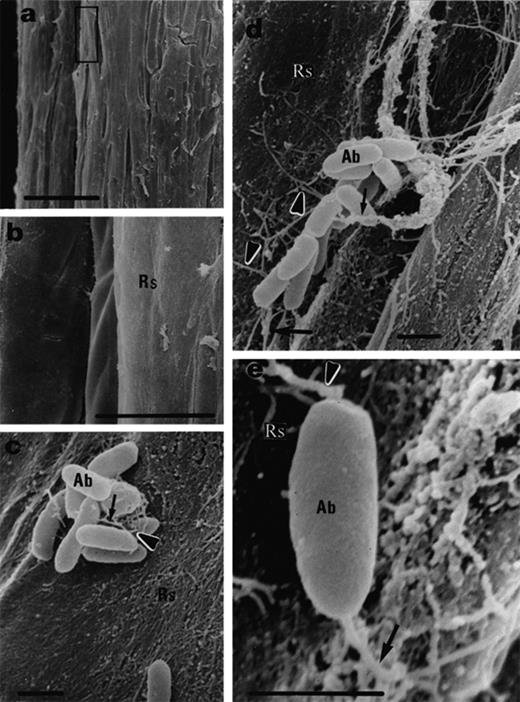
Black mangrove root colonization by A. brasilense Cd, 3 days after inoculation. a: non-inoculated roots. b: Magnification of (a) showing no bacteria on the root surface. c, d: Small colonies exhibit short (c) and long (d) fibrils (arrows). e: A single cell attaches to the root surface by fibrils (arrows). Abbreviations: Ab, A. brasilense Cd; Rs, root surface; Bars represent 100 μm (a), 10 μm (b) and 1 μm (c, d, e).
The root surface colonization by both A. brasilense and A. halopraeferens increased with time. After three days of inoculation, the population of A. brasilense Cd was composed mainly of small colonies in which the cells were connected to one another by short fibrils (i.e. approximately 1–3 μm) (Fig. 3c, d, arrows). In addition, some single bacteria were observed where these bacteria were connected to the root surface by fibrils that were approximately 1 μm long (Fig. 3e, arrows). After 4–8 days, A. brasilense Cd cells were no longer detected on the root surface, however, the total population of A. brasilense Cd cells detectable by bacterial plate counts was high, suggesting that the cells had colonized the interior of the root. Another possible explanation for the disappearance of A. brasilense Cd might be that the observed heavy mucilaginous covering was even more pronounced after extended growth of the bacteria on roots. Thus, it can obscure the presence of any bacterial cells residing on the root surface. In this possible explanation, the bacteria did not penetrate into the roots.
For A. halopraeferens, the colonization pattern was different from that observed with A. brasilense. A. halopraeferens was a better colonizer of the root surface. After three days of bacterial inoculation of mangrove seedlings, most bacteria were found as single cells embedded in a thick mucilaginous sheath on the root surface (Fig. 4a, b). In addition, many bacteria were detected beneath the sheath (Fig. 4c, arrows). In numerous areas along the root, a halo (thin arrows) surrounding the bacteria was detected. Here, the bacteria were connected to the sheath by short fibrils (thick arrows). The small bacterial aggregates were connected to the root surface by fibrils in a manner similar to that observed for A. brasilense (Fig. 4e, f, arrows). This pattern of binding remained unchanged throughout the course of the experiment (Fig. 5). The origin of these halos is as yet unknown. The possibility that these are artifacts caused by the SEM sample preparation was ruled out because an identical SEM sample preparation of black mangrove roots inoculated with the cyanobacteria M. chthonoplastes yielded no halos [23].
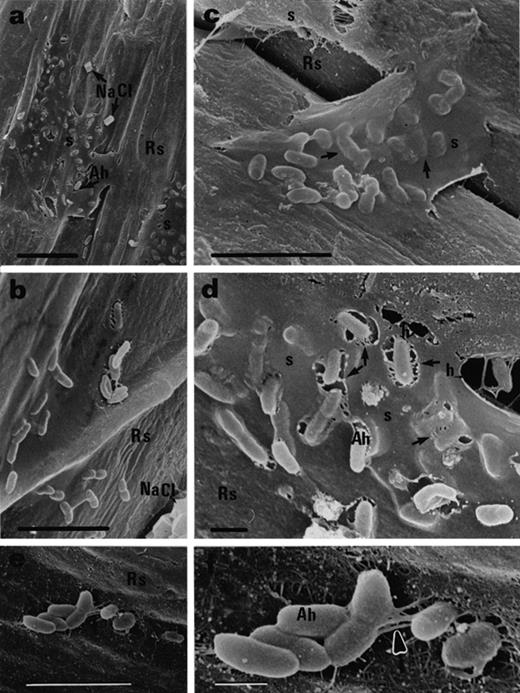
Black mangrove root colonization by A. halopraeferens AU10, 3 days after inoculation. a, b: Single cells embedded in a thick mucilaginous sheath. Note NaCl crystals on the root surface. c: Single cells reside under the sheath (arrows). d: Halos in the sheath (thin arrows) surrounding the bacteria which are connected to the surface by fibrils (thicker arrows). e: Small bacterial aggregates on the root surface. f: Magnification of (e) showing fibrils (arrow) connecting the aggregate to the root surface. Abbreviation: Ah, A. halopraeferens AU10; Rs, root surface; s, sheath; h, halo. Bars represent 10 μm (a), 5 μm (b, c, e) and 1 μm (d, f).
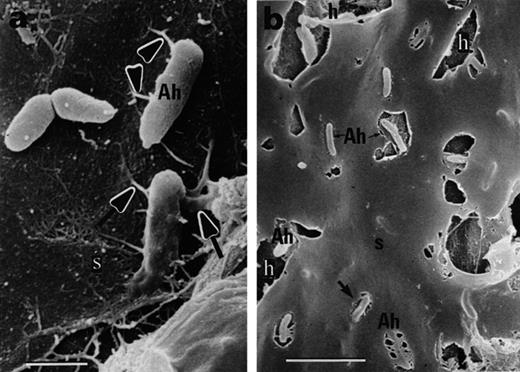
Black mangrove root colonization by A. halopraeferens AU10, 6 days after inoculation. a: Single cells on the root surface connected by short fibrils (arrows). b: Large halos in the sheath surrounding the bacteria. Abbreviation: Ah, A. halopraeferens AU10; s, sheath. Bars represent 1 μm (a) and 5 μm (b).
Cyanobacterial colonization of mangroves results in the production of a thick mucilaginous sheath after addition of the inoculum [23]. In the present study, a similar sheath was observed when mangroves were inoculated with A. halopraeferens. At present, little is known regarding the nature or the origin of this sheath. It may be root mucigel or bacterial exopolysaccharide, which Azospirillum is known to produce in large quantities [46,47], or a mixture of the two. It seems quite reasonable that the colonization of the surface and root interiors as well as the embedding in the mucilage layer results in an efficient osmoprotection, because Azospirillum itself has only a limited potential for cellular osmoprotection by osmolyte production [32,44].
Because of the short duration of these root colonization experiments (8 days) and the necessity to kill the plants after each sampling to maintain axenic cultures, no effect on the plant growth by inoculation was measured. Nevertheless, there are indications that black mangrove seedlings can derive fixed nitrogen after inoculation with the diazotrophic cyanobacteria M. chthonoplastes[15].
It was previously reported that Azospirillum can anchor to the surfaces of both terrestrial plant species [48] and sand particles [49] through the use of extended fibrils. As demonstrated in this study, Azospirillum appears to bind to mangrove roots in a similar manner. Species of Azospirillum have been reported to colonize the roots of a wide variety of plant species [19,27,50]. This study extends the range of host plants for this genus to one species of marine mangroves.
In summary, this study demonstrates that inoculation of mangroves with terrestrial halotolerant Azospirillum strains in seawater is feasible. The bacteria survived in seawater and were capable of colonizing the root surfaces and possibly root interiors.
4 Acknowledgments
This study is dedicated to the memory of our first English editor, the late Dr Roy Bowers, and to the memory of the late Mr Avner Bashan from Israel. We acknowledge the excellent technical help of Mr Dale Weber with the scanning electron microscopy and the advice of Dr Vladimir Lebsky. This study was partially supported by Consejo Nacional de Ciencia y Tecnologia (CONACyT), Mexico contracts number 2634 P-N, number 26262-B and 28362-B to G.H and Y.B. and by the Natural Science and Engineering Research Council of Canada to B.R.G.
References