-
PDF
- Split View
-
Views
-
Cite
Cite
Omid Saleh Ziabari, Binshuang Li, Nate B Hardy, Jennifer A Brisson, Aphid male wing polymorphisms are transient and have evolved repeatedly, Evolution, Volume 77, Issue 4, 1 April 2023, Pages 1056–1065, https://doi.org/10.1093/evolut/qpad024
- Share Icon Share
Abstract
Polymorphic phenotypes have long been used to examine the maintenance of genetic variation within and between species. Most studies have focused on persistent polymorphisms, which are retained across species boundaries, and their positive effects on speciation rates. Far less is known about the macroevolutionary impacts of more transient polymorphisms, which are also common. Here we investigated male wing polymorphisms in aphids. We estimated the phylogenetic history of wing states across species, along with several other traits that could affect wing evolution. We found that male wing polymorphisms are transient: they are found in only ~4% of extant species, but have likely evolved repeatedly across the phylogeny. We reason that the repeated evolution of transient polymorphisms might be facilitated by the existence of the asexual female wing plasticity, which is common across aphids, and would maintain the wing development program even in species with wingless males. We also discovered that male wingedness correlates positively with host plant alternation and host plant breadth, and that winged morphs and wing polymorphisms may be associated with higher speciation rates. Our results provide new evolutionary insights into this well-studied group and suggest that even transient polymorphisms may impact species diversification rates.
Introduction
Genetic polymorphisms describe the co-occurrence of distinct forms within populations such that the rarest morph cannot persist by recurrent mutation alone (Ford, 1945). Familiar examples include banding patterns in snail shells (Jones et al., 1977), heterostyly in plants (Kappel et al., 2017), and industrial melanism in peppered moths (Ford, 1965). These polymorphisms are likely to be adaptive, as deleterious morphs are quickly purged and neutral morphs are rare and slow to spread (Fisher, 1930), and similar polymorphisms often recur between species (Jamie & Meier, 2020).
A polymorphism can either be transient, when a new allele spreads through the population and replaces the other, or persistent, when it is maintained by a balance of selective forces. Transient polymorphisms are typically found within single species because of their short-lived nature. Balanced polymorphisms, on the other hand, can persist across species, and have long been used to study the maintenance of genetic variation (Ford, 1965). Polymorphism persistence across species can arise by shared ancestry or due to introgression re-introducing variation lost during speciation (Jamie & Meier, 2020). In some cases, closely related species are polymorphic, but the variation has arisen independently in each species due to new mutations. The relative abundance of transient versus persistent polymorphisms and the specific factors that promote one versus the other remain an open area of inquiry in evolutionary biology (see review Jamie & Meier, 2020).
An association between polymorphisms and elevated speciation rates has been found in multiple taxonomic groups, lending support to early theoretical work that proposed that adaptive polymorphisms may lead to rapid evolution, subsequent divergence, and speciation (Valen, 1965; West-Eberhard, 1986). But whether persistent polymorphisms promote speciation or if the process of speciation facilitates the persistence of polymorphism remains unclear. These ideas were developed and tested primarily with respect to color polymorphisms, and these models of speciation range from visual cues driving sexual selection to crypsis-promoting range expansions (Forsman et al., 2008; Galeotti & Rubolini, 2004; Gray & McKinnon, 2007). Studying less functionally versatile polymorphisms can simultaneously clarify relevant macroevolutionary processes while also exploring if these relationships hold for other types of polymorphisms.
Here our focus is on a particularly well-studied type of polymorphism: dispersal polymorphism in insects, wherein flight-capable and flight-incapable morphs co-occur within a species. Although insects are ancestrally winged, flight polymorphisms and winglessness have evolved repeatedly across a variety of insect orders (Roff, 1986; Zera & Denno, 1997). The alternative dispersal morphs display a suite of life-history traits that have fitness tradeoffs. Wingless females, for example, almost universally have higher fecundity and attain reproductive maturity faster (Harrison, 1980; Roff, 1986). The dispersal ability of wingless individuals is, however, limited; if suitable habitat patches are distant, only winged morphs can reach them. But flight is energetically expensive (Zera et al., 1994) and risky: winged morphs are susceptible to high predation and they must find suitable habitats (Jaenike, 1990).
The occurrence of flight polymorphisms across the majority of insect orders indicates that they have arisen many times, but what is not clear is how long they persist once they appear. Most investigations of flight polymorphisms have focused on their mechanistic basis, and/or tradeoffs between morphs, exploring one species at a time without the benefit of a broader evolutionary context (e.g., Denno & Grissell, 1979; Harrison, 1979). Rarely, the evolution of the polymorphisms has been considered among a few, closely related species, although the focus is often specifically on the loss of flight (Ikeda & Sota, 2011; Ikeda et al., 2012). An opportunity, therefore, arises for providing deeper insight into flight polymorphism evolution by examining occurrences at the macroevolutionary level.
Our purpose here is to use aphids for simultaneously studying polymorphism persistence and the effect of polymorphism on species diversification. Many aphid species have been intensively studied for their phenotypically plastic, female-specific wing plasticity. With these females, adverse environmental conditions result in winged females that can disperse, while optimal conditions produce wingless females with higher reproductive output (Miyazaki, 1987). The two morphs, therefore, co-occur in populations. This wing plasticity occurs during the spring and summer months among asexual females in virtually all aphid species. A lesser-known and more taxonomically variable wing polymorphism within aphids occurs in males. Males appear only in the fall and mate with sexual females to produce eggs for an overwintering diapause. Monographs have described species that have polymorphic, monomorphic winged, or monomorphic wingless males, but this information has never been examined within an evolutionary framework. It is, therefore, unclear how common or persistent male wing polymorphisms are in this group. The male polymorphism is genetically determined in the pea aphid (Caillaud et al., 2002), but whether or not all male polymorphisms are genetically determined is unknown. The presence of wing polymorphisms at different times in the life cycle (asexual females in spring and summer versus males in the fall) provides a chance to investigate if the female wing morph state associates with the male morph state. And if the states are not coupled, then the opportunity arises to discover the ecological factors that drive sex-specific wing morph evolution.
Here we use a phylogenetic framework to investigate the evolution of aphid male wing polymorphisms. We first use the vast aphid monograph resources to characterize individual species as having polymorphic, winged, or wingless males. We then use explicit models of phenotypic evolution to estimate ancestral phylogenetic wing states on a well-resolved, previously published (Hardy et al., 2015) phylogeny to ask three questions: (1) are aphid male wing polymorphisms transient or persistent?; (2) does the male polymorphism influence species diversification?; and (3) what ecological factors drive the rate of transition between wing states, and do they favor a particular wing state or the maintenance of polymorphism? These questions address fundamental aspects of the evolution of polymorphisms, especially in terms of characterizing the types of genetic variation (standing variation or new mutations) that underlie adaptations in natural populations.
Materials and methods
Data collection
We determined the winged, wingless, or polymorphism state of males for species primarily from the Blackman and Eastop monographs (aphidsonworldplants.info, Blackman & Eastop, 1994, 2000) by extracting taxonomic descriptions from the website to a searchable database of species and genera descriptions using custom Python and R scripts. These data were manually verified with other aphid monographs, aphid trap data from the Rothamsted Insect Survey, specimen collections (pers. comm., Andy Jenson), and other published studies (see Supplementary references for a full source list). Additionally, some higher-level taxonomic groups only have species that produce wingless males. For these, we did not rely on individual species descriptions, but rather coded all species within those groups accordingly. These groups are the families Anoeciidae, Hormaphididae, Mindaridae, Pemphigidae, and Thelaxidae (Heie, 1980; ~500 species); and two genera outside of these families (Davatchiaphis and Symydobius, with nine species, Supplementary Table S4). Also, there are 10 genera of aphids in Aphidinae that only have species producing winged males (~150 species, Supplementary Table S4) and were thus coded as winged. Altogether, we recovered male wing descriptions for 1,885 species out of 4,644 species (see Supplementary Table S10).
To begin to investigate the ecological factors that may drive wing state transitions, we collected information on host alternation, diet breadth, plant host type, and ant tending mainly from aphidsonworldplants.org. For host alternation and ant tending, we searched for keywords, e.g., the words “heteroecious” or “alternation” alone, or the words “ant” and “tend” in the same sentence, but coded no trait description as no trait (e.g., not tended by ants). For diet breadth, we cross-referenced the Tracheophyta NCBI taxonomic database (for vascular plants and mosses) with plant families and genera with aphid host descriptions to quantify the host plant families used. The diet breadth data are not a complete record but serve as a relative measure of the degree of host specialization. Host plant type was characterized as woody, herbaceous, or variable (a combination of both) using the Global Woodiness dataset (Fitzjohn et al., 2014). For analyses, the host type combined “woody” and “variable” together. For host-alternating species migrating between a primary and secondary host, the host plant breadth and host plant type was described for the secondary hosts only because the primary host reflects a constraint of foundresses more than the host repertoire of the summer asexual females (and males develop on the secondary hosts). Lastly, for host-alternating species we characterized if migrants were winged asexual females (searching “sexuparae” in descriptions, where an asexual female produces both males and sexual females on the primary host) or male migrants (searching for “gynoparae,” where the migrating female only produces egg-laying females, requiring male migrants to arrive at the primary host separately).
To characterize female wing states, the female wing plasticity was defined as species producing both winged and wingless female morphs, regardless of when they occur in the life cycle. In general, monographs assume females have both wing morphs, so we looked for cases where the description explicitly states a morph was missing (in contrast to male morphs, where it’s always been recognized that male states differ between species). We searched the Blackman and Eastop monograph descriptions for all female morphs using the keywords “alatae” and “apterae” which refer to the winged and wingless morphs of asexual females, respectively. The vast majority of species display both female wing morphs in asexual females. But 17 genera (~200 species, ~3% of species) primarily have winged asexual females (see Supplementary Table S4), but even in these species, other wingless female morphs are still produced (e.g., wingless egg-laying females). We found only one species which is described as having no winged females (Quadrartus agrifoliae).
Phylogenetic analysis of male wing evolution
The analyses in this article used the phylogeny estimated by Hardy et al., 2015. This fossil-calibrated phylogenetic tree has a wide representation of the diverse aphid lineages, and deeper nodes are congruent with other aphid phylogenies (Nováková et al., 2013; Ortiz-Rivas et al., 2004; von Dohlen & Moran, 1995), but see Owen and Miller, 2022. For each analysis, the data were trimmed to include only taxa with character data (resulting in 450 of the 584 species on the tree).
We estimated the ancestral male wing states of internal nodes using the ace function in the R package ape (Paradis & Schliep, 2019). We set the variable type as “discrete” and evaluated the relative fit of different discrete-trait models using log-likelihoods and Akaike weights. We evaluated six models with different transition rates between states (see Supplementary Table S1). Each model allowed different transition rates between states. We mapped the states of the best-fit model onto the maximum clade credibility tree.
To estimate both the time spent in each state and the number of transitions that occurred between wing states we simulated phylogenetic character histories. We used various discrete-trait models with the function make.simmap in the R package phytools (Revell, 2012). We set the transition matrix Q to “empirical” to fit a continuous-time reversible Markov model and ran 1,000 simulations for each of the 100 trees sampled from the posterior distribution (Hardy et al., 2015) to account for phylogenetic uncertainty. For some models (“ERtr” and “ARDtr”), some tree topologies showed obvious outliers and the increased simulation time reflected this (days versus hours for normal trees); these tree topologies were omitted for that model. We also simulated models under maximum parsimony to estimate these transition rates. Parsimony models have been shown to be more robust to the among-lineage rate variation that is known to affect Mk models as implemented in the ape package (King & Lee, 2015). We used the phytools function make.simmap to implement a maximum parsimony framework by using a very small prior (alpha = 1, beta = 1e4), and obtained transition estimates as above (see Supplementary Figure S3).
Wingless to winged state transitions (reversions) were estimated using a custom R script that found taxa with a high probability of gaining wings from a wingless background. A consensus of reversing species was obtained by comparing reversion searches on various tree topologies (100 trees from the posterior) and all six discrete-trait models. The most consistent reversing species were those that were identified regardless of the model used (present in all six models) and present in >50% of the tree topologies.
Speciation rates
We used the R package SecSSE (Herrera-Alsina et al., 2019) to test the association of male wing states and diversification rates. The state-dependent speciation and extinction (SSE) frameworks detect the dependence of diversification rates on traits (Maddison et al., 2007). But these models have been shown to yield false positives when there is rate heterogeneity that is unliked to the focal trait (Rabosky & Goldberg, 2015) as state-dependent models absorb rate variation resulting in a better fit than the null model. Recent methods resolve this issue by allowing a hidden state (HiSSE) to affect diversification rates (Beaulieu & O’Meara, 2016), but this model is limited to binary traits. We used the SecSSE framework as it extends the HiSSE model to simultaneously infer state-dependent diversification across more than two observed traits while still accounting for possible concealed states. Taxa with missing male wing information were coded as “NA,” so all 584 species of the phylogeny were used in this analysis.
We implemented the SecSSE model according to the package documentation. We coded the “examined” traits, male wing states, as winged, polymorphic, or wingless (states “1,” “2,” and “3,” respectively). We used the same number of “concealed” states as examined states as recommended (but see Supplementary Table S11), and coded them as “A,” “B,” and “C.” We defined three models with different speciation rates. The first is the constant-rates (“CR”) model where speciation rates (λ) are equal, regardless of the trait state (λ1A = λ2A = λ3A = λ1B = λ2B = λ2B = λ1C = λ2C = λ3C). The second model is a concealed trait-dependent (“CTD”) model where speciation rates are allowed to vary, but only between concealed states (λ1A = λ2A = λ3A ≠ λ1B = λ2B = λ2B ≠ λ1C = λ2C = λ3C). The last model is the examined trait-dependent (“ETD”) model where speciation rates are allowed to vary by examined state (λ1A = λ1B = λ1C ≠ λ2A = λ2B = λ2C ≠ λ3A = λ3B = λ3C). To reduce the computational burden, we set the transition rates of dual transitions to zero as they are unlikely to occur (e.g., from state 1 to 2 and state A to B). We also set transitions between monomorphic male wing states to zero (e.g., winged to and from wingless), requiring polymorphism to be the intermediate state (we removed this constraint in Supplementary Table S7, see below). Extinction rates were fixed for all models. This results in 28 parameters for the CTD and ETD models, and 26 for the CR model. We included a sampling frequency to account for missing species in our phylogeny as 450/4,644 species. Finally, we used Akaike weights to perform model selection.
We also simulated 327 tip randomizations on the same tree and estimated the ETD model likelihood values for each permutation (see Supplementary Figure S6) to evaluate whether our model fit was inflated as suggested by Rabosky and Goldberg, 2015. In an intermediate model between all wing states with the same rate and polymorphism as a transient state (Supplementary Table S7), we removed the constraint that wing states can transition to wingless states directly, and vice versa. We also used MiSSE, an extension of the HiSSE framework (Vasconcelos et al., 2022), to estimate trait-independent tip rates across the tree in Supplementary Figure S7, as implemented by Vasconcelos et al., 2022. In two additional SecSSE models, we explored clade-dependent diversification by clipping off the Aphidinae (Supplementary Table S8, and see Supplementary Figure S1 groups), and by exploring diversification rates in non-host-alternating species (Supplementary Table S9). Lastly, we also evaluated a simpler SecSSE model in Supplementary Table S11 where we used a two-rate model instead of the three rates of Supplementary Table S5. We did this by combining the wing and polymorphic speciation rates in the ETD 2-rates model (coded as “W/P” and “WL,” justifying this union as both rates are similar in Supplementary Table S5), and comparing a CTD model with only two rates (coded as “A” and “B”).
Evolutionary models of correlated ecological traits
We compiled several ecological traits that could impact wing states including host alternation, diet breadth, host type, and ant tending. We used various statistical models to assess the relationships between male wing evolution and ecological traits while accounting for phylogenetic relatedness. Discrete-trait values with more than two states were reduced to binary values to maximize statistical power. Wing states were coded as winged or wingless (with polymorphisms coded as winged); host alternation as whether a species alternates hosts or not; diet breadth as the number of host plant families occupied; host type as woody or herbaceous, with variable types coded as woody; and ant tending as tended or not, missing data coded as not. Note that while Figure 1C shows a discrete categorization of diet breadth to illustrate the range of variation, below we use the continuous variable of the count of host plant families recorded, which only includes the polyphagous species.
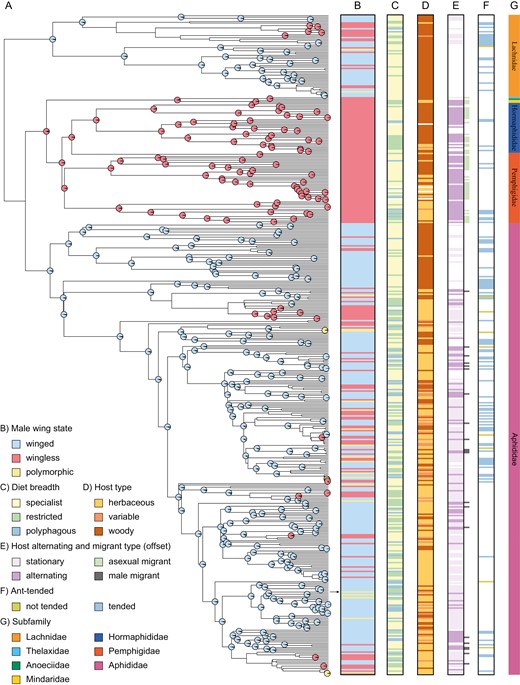
Male wing and life-history traits mapped onto the maximum clade credibility tree. (A) Phylogeny from (23) with the best-fit model of internal node ancestral reconstructions shown only for high-support nodes (>90%) for a single male wing state. Colors correspond to the male wing states shown in column B. Legend factors correspond to adjacent columns with different life-history and ecological traits mapped along the phylogeny. (B) Shows male wing state. The black arrow denotes the male wing polymorphic pea aphid, Acyrthosiphon pisum. (C) Diet breadth is binned into discrete classes of specialist, restricted, or polyphagous describing whether aphid species feed on a single genus of a host plant, within a family of a host plant, or many families of host plants, respectively. (D) Host plant type is shown, with woody plants in brown, herbaceous plants in beige, and a combination of both (variable) in burgundy. For host alternators, only the secondary hosts are described. (E) Host alternation is shown, with species described either as host alternating or not. Adjacent horizontal lines outside the black outline denote whether the host-alternating species has an asexual migrant (sexuparae that will birth a male back on the primary host) or a male migrant (birthed by a gynoparae on the secondary host, that needs wings to fly back to the primary host). (F) Ant tending is indicated by blue bars, and explicitly described not-tended species with a yellow bar. (G) Shows the primary aphid taxonomic subfamilies.
Host plant alternation refers to the ~10% of all aphid species that switch hosts for asexual reproduction in the summer and sexual reproduction in the fall (Eastop, 1977). Host-alternating species with male migrants must have winged males, so we expected a correlation between alternation and winged males. But some migrants are asexual, winged females that then produce both sexual females and males back on the primary host (sexuparae). The other type of host alternators migrates separately as males and asexual females that produce only sexual females back on the primary host (gynoparae), so males must have wings for the migration. We were able to categorize the type of host alternation for 81 species (Figure 1E, offset). All 19 species with male migrants for host alternation have winged males, but the remaining 62 species use asexual migrants (in Pemphigidae and Hormaphididae) and all have wingless males (Figure 1B, E, G).
To estimate phylogenetic trait associations, we combined ecological factors into a single multivariate model where the male wing state was the response variable. We used the R package MCMCglmm (Hadfield, 2010) to fit a phylogenetic generalized linear mixed model (PGLMM). Inverse-Wishart priors were set for the phylogenetic and residual variance (V = 1, nu = 0.002). The Markov chain Monte Carlo (MCMC) analysis was run for 10 million iterations, storing samples every 2,000 iterations. To fit threshold models, we used threshML via the R wrapper RthreshML from the Rphylip package (Revell & Chamberlain, 2014). The MCMC chain was run for 10,000,000 generations, and a burn-in of 5 million.
Results
To classify individual aphid species as producing solely winged, solely wingless, or polymorphic males, we compiled a total of ~1,900 male wing morph descriptions from published works; this is out of ~4,600 total aphid species. We found a near-equal proportion of monomorphic winged and monomorphic wingless species (48.5% and 48.8%, respectively); the remaining 2.7% are polymorphic. We mapped these male wing morph states onto the tips of the phylogeny presented by Hardy et al., 2015 and pruned taxa without wing state descriptions. Of the 450 species retained, 56% are monomorphic winged, 40% are monomorphic wingless, and 4% have polymorphic males.
We reconstructed the male wing states of ancestral nodes. Figure 1A shows the reconstructions from the best-fit model of character evolution, with tip states in Figure 1B. To illustrate key transitions, we only show nodes with greater than 90% support for a single state, but see all nodes in Supplementary Figure S1.
Male wing polymorphism is a transient, intermediate state
To examine patterns of male wing state evolution across the aphid phylogeny, we simulated character histories and identified the types of transitions that occurred (see Methods section for the models of evolution used). Two main results regarding the polymorphism emerged from this analysis. First, we found that polymorphic species are sparsely distributed across the tree and there is no clade of polymorphic species (Figure 1B), suggesting that these polymorphisms evolved independently. Second, we observed that wing polymorphism is the least common of the three wing states (winged, wingless, or polymorphic; Figure 2A). Moreover, our best-fitting model, which is also the most biologically realistic, is one that requires winged and wingless transitions to go through an intermediate, polymorphic state (Supplementary Table S1). The wing polymorphism is a transient state in this model because we restrict transitions from winged and wingless states explicitly, and time spent in a polymorphic state is consistently the least across tree topologies (Supplementary Figures S2 and S3).
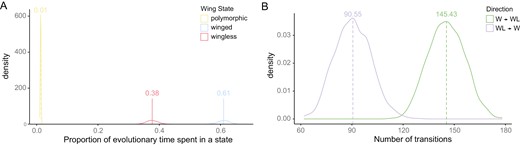
Estimates from stochastic character mapping simulations. (A) The proportion of evolutionary time spent in a single state estimated from the best-fit model. Distributions show simulation replicates on a single tree. The mean for proportions is shown above the distribution. Time spent in a polymorphic state is shown in yellow, as winged in blue, and as wingless in red. (B) The number of transitions estimated for the best-fit model is shown from a single tree topology. Wing loss (W->WL; W = winged, WL = wingless) is shown in green, and wing gain (WL->W) is shown in purple. Distributions show simulation replicates on a single tree. The mean for transitions is shown above distribution. Note that the best-fit model (“ARDtr”) necessitates all transitions between monomorphic wing states to pass through an intermediate polymorphic state (see Supplementary Table S1), such that these transitions more accurately show the gain of polymorphisms from winged (green) or wingless (purple) backgrounds. See Supplementary Figure S2 for mean estimates across all posterior tree topologies.
We also observed that transitions from winged to wingless males happened much more often than the reverse (Figure 2B, see Supplementary Figures S3 and S4 transitions across tree topologies, and Supplementary Figure S8 for transition distributions for all gains/loses of polymorphism). That wing gains occur at all is particularly interesting because wing loss is presumably an easier developmental genetic transition compared to wing gain. We therefore further explored individual instances of wing gains, calling these cases “reversions” because aphids are ancestrally winged (Heie, 1987). The bolded species in Figure 3 identify potential cases of reversions. These species have winged males emerging from wingless ancestors, regardless of the underlying model used to reconstruct internal nodes; these reversions were also recovered in a majority of the tree topologies (>50%, see Supplementary Table S2). We were able to examine one species (Brachycaudus lateralis) in the context of an independently-derived aphid phylogeny (Jousselin et al., 2010), which again showed wingless ancestors giving rise to a winged descendent (Supplementary Figure S4). We conclude that transitions from wingless to winged males are comparatively rare, but do happen.
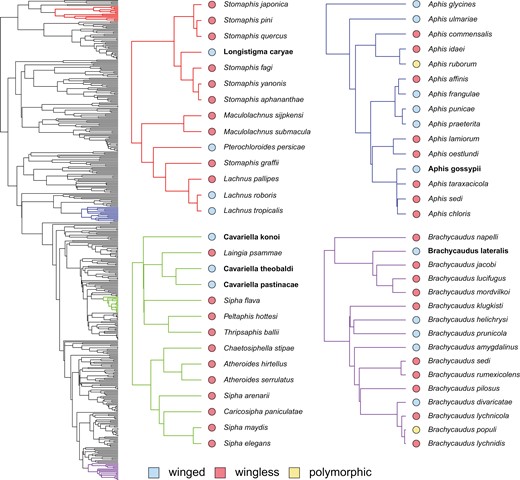
Wing reversions on the maximum clade credibility tree (same as Figure 1A). Bolded species highlight the reversals that occur across all six models of character evolution and in the majority of tree topologies. The red tree shows the reversing Longistigma caryae species in the Lachnidae subfamily; the blue tree shows Aphis gossypii in the Aphis genus; the green tree shows several Cavariella species; and the purple tree shows Brachycaudus lateralis in the Brachycaudus genus.
Speciation rates are higher among polymorphic and winged species
Using state-dependent speciation and extinction diversification models, we tested the relationship between male wing state and speciation rate by fitting a range of trait-dependent and trait-independent diversification models. Wing state-dependent speciation rates were the best-supported model (see Methods section for full details and support criteria). We found that speciation rates of polymorphic and winged male species were higher than rates of wingless species (Supplementary Tables S5 and S6).
Given the many caveats associated with various models exploring diversification rates, we pursued several other analyses to be comprehensive. We explored an intermediate model that allowed transitions between winged and wingless states directly to assess whether the wing polymorphism is actually transient. We found that the transient model (Supplementary Table S5) was better supported than the model with independent states (Supplementary Table S7). We also considered whether tip rates across the tree were clade-specific when estimated without specifying any traits using a MiSSE framework (see Methods section for details)., We found no clade-specific patterns (Supplementary Figure S7) to suggest tree topology alone inflated tip rates, but we note this does not improve the state-associated pattern’s robustness. We also explored two additional models on subsets of the tree, one with the Aphidinae clade pruned from the tree to see if the signals of diversification are inflated in that group (see Supplementary Table S8 and groupings in Supplementary Figure S1); the second subset explored only the non-host-alternating species (see Supplementary Table S9) to rule out whether diversification rates are driven by host alternation itself. We found the trait-dependent models were no longer the best fit in either case. In the non-host-alternating model, this may either reflect clade-dependent diversification rates or statistical issues of power (as we pruned out 60% of our full tree). However, the trait-independent MiSSE model turnover rates suggest there aren’t clade-specific effects, making it likely to be an issue of power. In the latter, non-alternating subset, state-dependent and -independent models have equal fit similarly reflecting statistical issues of power (as we pruned out 35% of our tree). Lastly, we also compared a simpler SecSSE model with two rates instead of three by merging together the winged and polymorphic speciation rates into one rate in the ETD model. Comparing this two-rate ETD model to a two-rate CTD model (Supplementary Table S11) still supports the ETD model as well as the speciation rate estimates. Altogether, our analyses suggest a relationship between male wing state and diversification, but the robustness of these models may be limited by the methods employed.
Male wing state evolves independently of asexual female wing state
Genetic correlations between the sexes may couple the evolution of wing state evolution even if morph-specific pressures differ by sex (Lande, 1980). Because wing plasticity polymorphism is so widespread among aphids, monographs often don’t explicitly mention its presence for a species. While some species do have asexual females that are monomorphic (see Supplementary Table S4), when we include all life cycle stages (e.g. foundresses or sexual females) the female wing plasticity is found across all major groups (Supplementary Figure S4), consistent with an understanding that it is ancestral to aphids (Braendle et al., 2006; Brisson, 2010). The existence of male wing state variation in the presence of invariable female plasticity supports the hypothesis that sex-specific wing states are independent. This is especially evident in the clade of the non-Aphidinae (Pemphigidae, Hormaphididae, and Thelaxidae subfamilies) which only have wingless males, whereas females display both winged and wingless morphs (Supplementary Figure S4). Thus, we conclude that male wing morph evolution is decoupled from female wing states.
Male wing states correlate with host plant breadth and host alternation
Several ecological factors affect winged or winglessness in female aphids, but their effects on males are generally unknown. These factors are host plant alternation (alternator or not), diet breadth (number of different plant families used), ant tending (tended or not), and host plant type (woody or herbaceous). Host-alternating species with male migrants must be winged, so we expected to observe a positive correlation between host plant alternation and winged males. For diet breadth, we predicted a positive correlation between increased breadth and male wingedness, reasoning that winged males would increase mating success with other winged females on different host plants. For species tended by ants, the mutualism would benefit aphids that stay close to their colony more than those that disperse (Johnson, 1959; Yao, 2012), so we predicted a positive correlation between winglessness and ant tending. We did not have an expectation for host plant type: herbaceous plants are more short-lived and therefore dispersal ability may be favored (Groeters, 1989; Roff, 1990), but movement on large, architecturally complex trees can require flight (Dixon, 1984; Waloff, 1983).
We found that only host plant alternation and host plant breadth had significant correlations with wing state (multivariate phylogenetic generalized linear model, p < .0005 for both factors, Supplementary Table S3) and it was in the direction expected: winged males exhibited host alternation and had a wider diet breadth. These directions were also supported in an independent approach with Felsenstein’s thresholding model (Felsenstein, 2005, see Supplementary Table S3).
Discussion
Here we examined wing polymorphisms in male aphids within a macroevolutionary framework. Our primary results showed that wing polymorphisms are transient and that polymorphic and winged species have an elevated speciation rate. Below we discuss how these results compare with the literature, and how they provide broader insights about polymorphism evolution.
First, aphid male wing polymorphisms are transient and likely arose independently by new mutations. Most macroevolutionary studies of polymorphic phenotypes have focused on persistent polymorphisms (reviewed in Jamie & Meier, 2020), wherein polymorphic taxa cluster together as clades of related species. In these cases, polymorphism has persisted across speciation via shared ancestry or introgression, where the independent origins of these polymorphisms are unlikely. In aphids, however, the evolutionary distance between polymorphic species makes it very likely that polymorphisms have evolved independently by new mutations. This contrast invites questions about why some species can rely on new mutations to re-introduce lost variation and why some cannot, instead favoring the maintenance of ancestral variation. Future studies contrasting these types of cases will help illuminate the factors that constrain when mutational forces can target alternative morph evolution.
The de novo evolution of the male wing polymorphism may be facilitated by the prevalence of the female wing plasticity (Supplementary Figure S5). Within all species having the female plasticity, there is a developmental program that produces a winged morph, and a developmental program that produces a wingless morph. If males lose one of the morphs in any particular species, the developmental program would still exist and be active in the females. Under this framework, the wing and wingless morph developmental programs remain intact across species, while a male-specific mechanism of activating that program evolves. This may explain how the winged state can arise from a wingless state (Figure 2B), and/or how male wing polymorphisms can repeatedly emerge. This type of sex-specific developmental modularity can potentially cheat Dollo’s law of irreversibility, which states that once lost, traits won’t re-emerge (“cheating” because it’s never really lost in females (Gould, 1970)). This kind of redeployment of developmental modules is a general feature of morphological evolution (Carroll, 2008).
Second, we discovered that winged and polymorphic species have a higher speciation rate than wingless species. Other investigations in insects have shown accelerated speciation rates in wingless species, attributing this pattern to the decreased gene flow and concurrent buildup of differentiation between populations (Ikeda et al., 2012; Smith & Farrell, 2006; Zera & Denno, 1997). Here we instead found that wingless species have the lowest rates, and that winged and polymorphic species have elevated rates (Supplementary Table S5). The primary reason for this difference in aphids is likely because, as noted in the introduction, dispersal capabilities are maintained by asexual, winged females during the spring and summer months. Across a patchy environment, even if a species solely produces nondispersive wingless males, there is no opportunity for population substructure to arise solely due to a lack of dispersal in the males. Note that this relationship between dispersal and the sexes changes dramatically in the autumn because the egg-laying sexual females (oviparae) are virtually all wingless across aphid species, leaving males as the only dispersive morph during that time.
While the higher diversification rates are at odds with patterns shown in other insects, they are in line with the broader literature associating phenotypic polymorphisms to higher speciation rates (Corl et al., 2018; Hugall & Stuart-Fox, 2012). But the direction of causation is unclear when polymorphic species cluster together, and it remains challenging to distinguish whether polymorphism facilitates speciation or whether speciation tends to retain polymorphic species (see review 6). The models of speciation retaining polymorphisms require rapid radiations where incomplete divergence enables introgression to re-introduce lost morphs. In aphids, male polymorphisms do not persist and are not found in groups of related species. This pattern argues that the direction of causation in aphids is that polymorphisms promote speciation. One model of how this could occur is that wing polymorphisms could facilitate the exploitation of a new ecological niche, and this niche expansion leads to rapid evolution, subsequent divergence, and then speciation (Forsman et al., 2008; Jaenike, 1990; Valen, 1965; West-Eberhard, 1986).
One aphid species model reveals more microevolutionary dynamics of the male wing polymorphism. In the pea aphid (Acyrthosiphon pisum; black arrow in Figure 1A), the genetic basis of polymorphism is known: it is a single locus with 120 kb insertion on the wingless allele that is possibly as old as 10 million years (Li et al., 2020). Interestingly, the pea aphid is actually a species complex of host-plant-associated biotypes (Peccoud, Simon, et al., 2009) and each displays a range of winged allele frequencies from zero to near 100% (Li et al., 2020). In this species, it’s possible that different morphs have been lost within lineages but then regained by introgression, given the gene flow that happens among some biotypes (Peccoud, Ollivier, et al., 2009). This use and re-use of ancestrally divergent alleles could allow rapid adaptation of males when environmental conditions change, paralleling the use and re-use of ancestrally ancient (~200,000 ya) long- and short-winged alleles in saltmarsh beetles across habitats (Van Belleghem et al., 2018). The presence of the biotype radiation in the pea aphid may also turn out to be an illuminating example of how male wing polymorphism results in higher rates of speciation, especially in conjunction with host plant specialization. This is supported by the polymorphism being four times older than the earliest estimates of the biotype radiation (~500,000 years ago, Fazalova et al., 2020). In this scenario, wing polymorphism evolved and then facilitated the move to new or underutilized plant hosts; ensuing specialization promoted monomorphism of both types among descendent lineages. Thus, transience at the macroevolutionary level may be persistent at a more microevolutionary level, potentially driving incipient speciation of the pea aphid complex.
Our study also revealed many transitions between wing states across species. We identified some factors correlated with these wing states: wider diet breadth and host alternation (with male migrants) tended to associate with winged males (Supplementary Table S3). But other, less easily measurable factors like inbreeding and mate competition are also likely driving aphid wing states. For inbreeding, males mating with their sisters is the genetic equivalent of self-fertilization due to XO sex determination. Wingless males incapable of migration will promote inbreeding, and winged males, capable of migration, ensure outcrossing. For local mate competition, Moran (1993) predicted that the balance between the strength of local mate competition in males and local resource competition in females would affect both the male wing states and the degree of sex-ratio bias. Winged males are expected when the strength of local resource competition among females is stronger than local mate competition in males; this would also promote male-biased sex ratios. In contrast, wingless males would be expected when local mate competition is stronger than local resource competition; this would promote female-biased sex ratios. Most aphid species have wingless sexual females which compete for local resources. Winged or wingless males will result in either high or low mate competition, respectively, relative to resource competition.
An example of these factors at play can be found in the large clade of wingless males in Figure 1. These are all host-alternating species that produce a winged female migrant that disperses to the primary host plant and gives live birth to sexual females and males. This means that the primary host will harbor a mix of different aphid clones, which will in turn result in low intra-clone mate competition, low inbreeding levels, and high resource competition. Moran’s model (Moran, 1993) predicts that under these conditions, males would be wingless and sex ratios would be female-biased; this is exactly the case (sex ratios reported in Foster & Benton, 1992; Moran, 1993; Yamaguchi, 1985).
In conclusion, the developmental modularity that decouples male and female wing morph determination enables the parallel evolution of the male wing polymorphism through new mutations. While this explains how the male polymorphism has evolved repeatedly, reversibly, and independently, the conditions which prolong the duration of a polymorphism long enough to lead to divergence require more species-level analyses. The relationship between male wing transitions as a response to inbreeding pressures may also help explain how aphid species can rely on new mutations to re-introduce lost variation. Nevertheless, our comparative approach in these analyses highlights the importance of new mutations in the production of new wing polymorphisms, as well as providing support to the extent that polymorphisms can potentially act as precursors of speciation. In the context of the type of genetic variation that selection acts upon to promote adaptations, this work supports a model where developmental modularity can filter new, large-effect mutations to drive adaptation.
Data availability
All scripts and data have been deposited on Dryad (https://doi.org/10.5061/dryad.z612jm6gd).
Author contributions
O.S.Z., B.L., and J.A.B. conceived the project, O.S.Z., B.L., and N.B.H. analyzed data, and O.S.Z., N.B.H, and J.A.B wrote and reviewed the manuscript.
Conflict of interest: The authors declare no conflict of interest.
Acknowledgments
We are grateful to Andy Jenson, who provided us with his aphid slide collection information and for helpful discussion. This research was supported by awards R01GM116867 and R35GM144001 from the National Institute of General Medical Sciences to J.A.B. and award #1939268 from the National Science Foundation Graduate Research Fellowship to O.S.Z. We are grateful to collaborators and staff who have contributed data from the Rothamsted Insect Survey, a National Capability, funded by the Biotechnology and Biological Sciences Research Council under the Core Capability Grant BBS/E/C/000J0200.