-
PDF
- Split View
-
Views
-
Cite
Cite
Miguel A. Cruz, Sara Magalhães, Élio Sucena, Flore Zélé, Wolbachia and host intrinsic reproductive barriers contribute additively to postmating isolation in spider mites, Evolution, Volume 75, Issue 8, 1 August 2021, Pages 2085–2101, https://doi.org/10.1111/evo.14286
- Share Icon Share
Abstract
Wolbachia are maternally-inherited bacteria that induce cytoplasmic incompatibility in many arthropod species. However, the ubiquity of this isolation mechanism for host speciation processes remains elusive, as only few studies have examined Wolbachia-induced incompatibilities when host populations are not genetically compatible. Here, we used three populations of two genetically differentiated colour forms of the haplodiploid spider mite Tetranychus urticae to dissect the interaction between Wolbachia-induced and host-associated incompatibilities, and their relative contribution to postmating isolation. We found that these two sources of incompatibility act through different mechanisms in an additive fashion. Host-associated incompatibility contributes 1.5 times more than Wolbachia-induced incompatibility in reducing hybrid production, the former through an overproduction of haploid sons at the expense of diploid daughters (ca. 75% decrease) and the latter by increasing the embryonic mortality of daughters (by ca. 49%). Furthermore, regardless of cross direction, we observed near-complete F1 hybrid sterility and complete F2 hybrid breakdown between populations of the two forms, but Wolbachia did not contribute to this outcome. We thus show mechanistic independence and an additive nature of host-intrinsic and Wolbachia-induced sources of isolation. Wolbachia may contribute to reproductive isolation in this system, thereby potentially affecting host differentiation and distribution in the field.
In the last decades, it has become increasingly clear that species are not fixed entities and that populations exhibit different degrees of reproductive isolation between them (Hendry et al. 2000; Powell et al. 2013; Burri et al. 2015; Supple et al. 2015). Indeed, hybridization is taxonomically widespread, and ample variation in the extent and permeability of various reproductive barriers occurs both within and between species (Pinto et al. 1991; Mallet 2008; Hendry et al. 2009; Nosil et al. 2009). Moreover, theoretical studies show that stable partial reproductive isolation can be relatively common (reviewed by Servedio and Hermisson 2020).
Partial reproductive isolation between lineages (i.e., differentiated populations or incipient species) can evolve in both sympatry and allopatry due to divergent (including disruptive; Rueffler et al. 2006) sexual and/or ecological selection, and/or as a result of stochastic processes (Schluter 2001, 2009; Turelli et al. 2001; Bolnick and Fitzpatrick 2007; Maan and Seehausen 2011; Nosil 2012). Additionally, in arthropods, variable degrees of reproductive isolation, between and within lineages, can result from infection by different cytoplasmically inherited bacterial reproductive manipulators (Duron et al. 2008; Engelstädter and Hurst 2009; Brucker and Bordenstein 2012; Faria and Sucena 2015), among which Wolbachia is the most widespread (Weinert et al. 2015). This endosymbiont can induce various phenotypes of reproductive manipulation in its hosts, the most common being cytoplasmic incompatibility (CI; Werren et al. 2008; Engelstädter and Hurst 2009). CI is a conditional lethality phenotype resulting in increased embryonic mortality of offspring from crosses between infected males and uninfected females (or females harbouring an incompatible strain). Thus, Wolbachia-induced CI can lead to substantial barriers to gene flow between individuals with different infection status, and could act as an agent of speciation (Laven 1959; Werren 1998; Bordenstein et al. 2001; Telschow et al. 2005b; Jaenike et al. 2006; Miller et al. 2010).
In particular, when different host populations are infected with incompatible strains of Wolbachia, bidirectional CI (i.e., both directions of crosses are incompatible) may prevent or reduce gene flow between such populations (Bordenstein et al. 2001). This will promote genetic divergence even in the face of relatively high levels of migration, under low levels of Wolbachia transmission, and with incomplete CI ( that is less than 100% offspring mortality; Telschow et al. 2002a, 2002b, 2005a). In addition, theory predicts and empirical studies confirm that bidirectional CI can lead to reinforcement, which further corroborates its role in speciation processes (e.g., Bordenstein et al. 2001; Telschow et al. 2005b). In contrast, gene flow restricted to one direction of crosses may be insufficient to promote speciation as in the case of unidirectional CI occurring between infected and uninfected populations (or between populations infected with different Wolbachia strains, where only one can rescue CI induced by the other). Moreover, unidirectional CI favours the rapid invasion of host populations by a single Wolbachia strain, progressively eliminating the action of CI. In such case, unidirectional CI fails to produce a persistent barrier to gene flow, leading to the subsequent elimination of genetic differences between diverging populations (Hurst and Schilthuizen 1998; Werren 1998; Weeks et al. 2002; Bordenstein 2003). Nevertheless, infection polymorphisms, with populations composed of infected and uninfected hosts, or hosts infected with unidirectionally compatible Wolbachia strains, are often found in natural populations of many host species (e.g., Vavre et al. 2002; Keller et al. 2004; Zhang et al. 2013; Hamm et al. 2014; Zélé et al. 2020b). This observed pattern is consistent with theoretical predictions that unidirectional CI can contribute to host divergence at moderate migration rates and stabilize such polymorphisms (Telschow et al. 2002a, 2007). Given enough time under such polymorphism, Wolbachia-induced unidirectional CI may drive reproductive character displacement in uninfected hosts, further reinforcing reproductive isolation between uninfected and infected hosts in sympatry, as well as with uninfected allopatric populations (i.e., “cascade reinforcement”; Jaenike et al. 2006). Finally, given its high prevalence in natural populations, Wolbachia-induced CI is likely to co-occur with other (host-associated) isolation mechanisms, thereby potentially driving host speciation processes more forcefully than when acting alone (Telschow et al. 2014).
The fact that natural populations of many organisms often display variable degrees of reproductive isolation (Scopece et al. 2010; Jennings et al. 2011; Corbett-Detig et al. 2013; Harrison and Larson 2014) offers an excellent opportunity to address the role of Wolbachia-induced CI in ongoing speciation processes. Still, this has been addressed in few systems, and three different, contrasting scenarios have been described: (1) Wolbachia did not induce CI in interspecific crosses (e.g., in Gryllus and Drosophila; Maroja et al. 2008; Gazla and Carracedo 2009; Cooper et al. 2017); (2) Wolbachia alone was responsible for postmating isolation between species through bidirectional CI (e.g., in Nasonia; Bordenstein et al. 2001); (3) Wolbachia and host genetic factors acted jointly, either in the same direction of crosses (e.g., some of the crosses between populations of Panonychus mori; Gotoh et al. 2005), or in the opposite direction, thereby complementing each other in establishing bidirectional postmating isolation between species (e.g., in Drosophila and Aedes; Shoemaker et al. 1999; Dean and Dobson 2004; see also Gebiola et al. 2016 for CI induced by Cardinium in Encarsia). When both sources of incompatibility jointly reduce gene flow between genetically differentiated host populations, a full factorial design is necessary to precisely quantify the relative contributions of Wolbachia-induced and host genetic barriers to postmating isolation, and to determine whether these effects are additive or interacting. To our knowledge, this has not been implemented yet, which is at odds with the relevance of such data to better understand the exact role of Wolbachia in ongoing processes of speciation in arthropods.
Tetranychus spider mites constitute an excellent system to address the interplay between symbiont-induced and host-associated reproductive incompatibilities. Indeed, they are arrhenotokous haplodiploids (i.e., males are haploid, arising from unfertilized eggs, and females are diploid, arising from fertilized eggs; Helle and Bolland 1967), which allows assessing fertilization failure by measuring sex ratios. Spider mites are also often infected with different CI-inducing (or non-inducing) Wolbachia strains, whose prevalence greatly varies in natural populations (ranging from 0 to 100%; Gotoh et al. 2003, 2007; Zhang et al. 2016; Zélé et al. 2018a). As in other haplodiploid species (see Breeuwer and Werren 1990; Vavre et al. 2001), Wolbachia-induced CI can have two different consequences in spider mites, depending on the population tested (e.g., Perrot-Minnot et al. 2002; Gotoh et al. 2003). In most cases, as in diploid species, eggs from uninfected females fail to hatch when fertilized by sperm from Wolbachia-infected males, but CI affects only the female offspring because males arise from unfertilized eggs (Female mortality—FM-CI-type incompatibility; Breeuwer 1997; Vala et al. 2002; Gotoh et al. 2007; Xie et al. 2010; Suh et al. 2015; Bing et al. 2019; Zélé et al. 2020b). In other cases, Wolbachia-induced CI leads to complete elimination of the paternal set of chromosomes after fertilization of the egg, which successfully develops as a viable haploid male instead of female (male development—MD-type incompatibility; Vala et al. 2000; Perrot-Minnot et al. 2002; Gotoh et al. 2003). In both cases, the penetrance of CI (i.e., the number of embryos affected) greatly varies among populations (from 0 to more than 90% for FM-type and from 0 to 100% for MD-type CI; Perrot-Minnot et al. 2002; Vala et al. 2002; Gotoh et al. 2007; Xie et al. 2010; Suh et al. 2015; Zélé et al. 2020b). Finally, Wolbachia can also cause hybrid breakdown in Tetranychus urticae, with an increased mortality of F2 recombinant males produced by F1 hybrid females that escape CI (Vala et al. 2000). To date, however, the origin (i.e., Wolbachia strain, host genetic background, or both) of such variability in CI patterns and penetrance is still unknown in spider mites.
Regardless of Wolbachia manipulation, variable degrees of reproductive isolation have been found both between and within Tetranychus species (e.g., Keh 1952; Takafuji and Fujimoto 1985; Navajas et al. 2000; Sato et al. 2015; Clemente et al. 2016; Knegt et al. 2017), including between two recently diverged colour forms of the well-studied species T. urticae (Chen et al. 2014; Matsuda et al. 2018). These two closely related forms have a worldwide distribution (Migeon and Dorkeld 2020), they share the same host plant range (Auger et al. 2013), and they can even be found on the same individual plant (Lu et al. 2017; Zélé et al. 2018a). Therefore, they naturally co-occur and possibly often interact in the field. Due to complete reproductive isolation among some populations of the two forms, they were historically described as separate species (T. urticae and T. cinnabarinus, for the “green” and the “red” form, respectively; Boudreaux 1956; Van de Bund and Helle 1960; Helle and Van de Bund 1962; Smith 1975). Nevertheless, due to similarity in morphological and biological traits (e.g., aedeagus shape, life cycle, host plant range; Auger et al. 2013), and given that many populations of the two forms are not fully reproductively isolated (Murtaugh and Wrensch 1978; Dupont 1979; de Boer 1982a, 1982b; Sugasawa et al. 2002), subsequent studies reclassified them as semispecies (Goka et al. 1996) or members of the same species (Dupont 1979; Fry 1989; Gotoh et al. 1993; Auger et al. 2013). Taken together, these studies thus suggest that speciation is currently ongoing in this species complex, but the role played by Wolbachia-induced CI in such process is as yet unknown. Indeed, almost all studies addressing reproductive isolation in this system predate the identification of Wolbachia in spider mites by Breeuwer and Jacobs (1996), and, to our knowledge, only two studies have been conducted since then. One of these showed partial incompatibility (interbreeding was performed for five generations) between a Wolbachia-uninfected red-form population and a green-form population infected by a non-CI-inducing strain (Sugasawa et al. 2002). The other study showed full reproductive isolation between one green-form population and two red-form populations, but Wolbachia infection was not assessed (Lu et al. 2017).
Here, we assessed the interplay and the relative contribution of Wolbachia-induced CI and host-associated incompatibilities (HI) on postmating isolation between three naturally Wolbachia-infected populations, two from the red form and one from the green form of T. urticae. We performed all possible crosses between Wolbachia-infected and Wolbachia-free populations in a full-factorial design and measured the embryonic and juvenile mortality of the offspring, as well as the proportion of males and females produced from each cross, over two generations.
Materials and Methods
SPIDER MITE POPULATIONS
Three different populations of spider mites, all collected in Portugal and naturally infected with Wolbachia, were used in this study. Two populations, “Ri1” and “Ri2,” belong to the red form of T. urticae and share the same ITS2 rDNA and COI mtDNA sequences. The third population, “Gi,” belongs to the green form of T. urticae and differs from the former two populations in both ITS2 rDNA and COI mtDNA (cf. detailed information in Supporting information Box S1). The Wolbachia strains infecting Ri1 and Ri2 are mutually compatible but induce different levels of CI despite identical MLST profiles (Zélé et al. 2020b). The Wolbachia strain infecting Gi, however, slightly differs from the former two based on MLST and whether it induces CI in this population was heretofore unknown. Since field collection (cf. Supporting information Box S1), these populations were reared in the laboratory under standard conditions (24 ± 2°C, 16/8 h L/D) at very high numbers (ca. 500-1000 females per population) in mite-proof cages containing bean plants (Phaseolus vulgaris, cv. contender seedlings obtained from Germisem, Oliveira do Hospital, Portugal).
ANTIBIOTIC TREATMENTS
After collection, subsets of Gi, Ri1, and Ri2 populations were treated with antibiotics to obtain the corresponding Wolbachia-free populations Gu, Ru1, and Ru2. For logistical reasons, the populations Gu and Ru2 used in each of the two experiments reported here were created from two different antibiotic treatments. For Experiment 1, Gu was obtained from a treatment performed in November 2013, and Ru1 and Ru2 from treatments performed in February 2014. Briefly, 100 Gi and 30 Ri1 or Ri2 adult females were installed in petri dishes containing bean leaf fragments, placed on cotton soaked in a tetracycline solution (0.1%, w/v) for three successive generations (Breeuwer 1997; Zélé et al. 2020b). For Experiment 2, Ru1 came from the previous antibiotic treatment but Gu and Ru2 were obtained from new treatments performed in September 2016 and January 2017, respectively. In this case, 300 Gi or Ri2 adult females were installed in petri dishes containing fragments of bean leaves placed on cotton soaked in a rifampicin solution (0.05%, w/v) for one generation (Gotoh et al. 2005; Zélé et al. 2020a). All antibiotic treatments were performed in the same standard conditions as population rearing (24 ± 2°C, 16/8h L/D). After treatment, Wolbachia-free populations were maintained without antibiotics in the same mass-rearing conditions as the Wolbachia-infected populations for a minimum of three generations to avoid potential side-effects of antibiotics (Ballard and Melvin 2007; Zeh et al. 2012; O'Shea and Singh 2015). Subsequently, pools of 100 females from each population were checked by multiplex PCR as described by Zélé et al. (2018b) to confirm their Wolbachia infection status before performing the experiments.
EXPERIMENT 1: F1 PRODUCTION AND VIABILITY
The combined effect of Wolbachia- and host-associated incompatibilities on offspring production was investigated by performing all crosses between Wolbachia-infected and uninfected individuals from all populations in a full factorial design. These crosses were organized into five different categories, each with a different purpose (cf. Table 1).
Category . | Type of crosses . | Crosses (♀ × ♂) . |
---|---|---|
| Intrapopulation crosses using ♀ and ♂ with the same infection status | Ru1 × Ru1 and Ri1 × Ri1 Ru2 × Ru2 and Ri2 × Ri2 Gu × Gu and Gi × Gi |
B - Test for Wolbachia-induced CI only | Intrapopulation crosses using uninfected ♀ and infected ♂ | Ru1 × Ri1 Ru2 × Ri2 Gu × Gi |
C - Test for HI only, in absence of Wolbachia | Interpopulation crosses using uninfected ♀ and uninfected ♂ | Ru1 × Ru2 or Gu Ru2 × Ru1 or Gu Gu × Ru1 or Ru2 |
D - Test for Wolbachia-induced CI-HI interaction | Interpopulation crosses using (un)infected ♀ and infected ♂ | Ru1 or Ri1 × Ri2 or Gi Ru2 or Ri2 × Ri1 or Gi Gu or Gi × Ri1 or Ri2 |
E - Test for HI only in presence of Wolbachia but without CI, to verify that infection itself does not affect HI* | Interpopulation crosses using infected ♀ and uninfected ♂ (incl. intrapopulation controls) | Ri1 × Ru2 or Gu Ri2 × Ru1 or Gu Gi × Ru1 or Ru2 (Ri1 × Ru1, Ri2 × Ru2, Gi × Gu) |
Category . | Type of crosses . | Crosses (♀ × ♂) . |
---|---|---|
| Intrapopulation crosses using ♀ and ♂ with the same infection status | Ru1 × Ru1 and Ri1 × Ri1 Ru2 × Ru2 and Ri2 × Ri2 Gu × Gu and Gi × Gi |
B - Test for Wolbachia-induced CI only | Intrapopulation crosses using uninfected ♀ and infected ♂ | Ru1 × Ri1 Ru2 × Ri2 Gu × Gi |
C - Test for HI only, in absence of Wolbachia | Interpopulation crosses using uninfected ♀ and uninfected ♂ | Ru1 × Ru2 or Gu Ru2 × Ru1 or Gu Gu × Ru1 or Ru2 |
D - Test for Wolbachia-induced CI-HI interaction | Interpopulation crosses using (un)infected ♀ and infected ♂ | Ru1 or Ri1 × Ri2 or Gi Ru2 or Ri2 × Ri1 or Gi Gu or Gi × Ri1 or Ri2 |
E - Test for HI only in presence of Wolbachia but without CI, to verify that infection itself does not affect HI* | Interpopulation crosses using infected ♀ and uninfected ♂ (incl. intrapopulation controls) | Ri1 × Ru2 or Gu Ri2 × Ru1 or Gu Gi × Ru1 or Ru2 (Ri1 × Ru1, Ri2 × Ru2, Gi × Gu) |
Category . | Type of crosses . | Crosses (♀ × ♂) . |
---|---|---|
| Intrapopulation crosses using ♀ and ♂ with the same infection status | Ru1 × Ru1 and Ri1 × Ri1 Ru2 × Ru2 and Ri2 × Ri2 Gu × Gu and Gi × Gi |
B - Test for Wolbachia-induced CI only | Intrapopulation crosses using uninfected ♀ and infected ♂ | Ru1 × Ri1 Ru2 × Ri2 Gu × Gi |
C - Test for HI only, in absence of Wolbachia | Interpopulation crosses using uninfected ♀ and uninfected ♂ | Ru1 × Ru2 or Gu Ru2 × Ru1 or Gu Gu × Ru1 or Ru2 |
D - Test for Wolbachia-induced CI-HI interaction | Interpopulation crosses using (un)infected ♀ and infected ♂ | Ru1 or Ri1 × Ri2 or Gi Ru2 or Ri2 × Ri1 or Gi Gu or Gi × Ri1 or Ri2 |
E - Test for HI only in presence of Wolbachia but without CI, to verify that infection itself does not affect HI* | Interpopulation crosses using infected ♀ and uninfected ♂ (incl. intrapopulation controls) | Ri1 × Ru2 or Gu Ri2 × Ru1 or Gu Gi × Ru1 or Ru2 (Ri1 × Ru1, Ri2 × Ru2, Gi × Gu) |
Category . | Type of crosses . | Crosses (♀ × ♂) . |
---|---|---|
| Intrapopulation crosses using ♀ and ♂ with the same infection status | Ru1 × Ru1 and Ri1 × Ri1 Ru2 × Ru2 and Ri2 × Ri2 Gu × Gu and Gi × Gi |
B - Test for Wolbachia-induced CI only | Intrapopulation crosses using uninfected ♀ and infected ♂ | Ru1 × Ri1 Ru2 × Ri2 Gu × Gi |
C - Test for HI only, in absence of Wolbachia | Interpopulation crosses using uninfected ♀ and uninfected ♂ | Ru1 × Ru2 or Gu Ru2 × Ru1 or Gu Gu × Ru1 or Ru2 |
D - Test for Wolbachia-induced CI-HI interaction | Interpopulation crosses using (un)infected ♀ and infected ♂ | Ru1 or Ri1 × Ri2 or Gi Ru2 or Ri2 × Ri1 or Gi Gu or Gi × Ri1 or Ri2 |
E - Test for HI only in presence of Wolbachia but without CI, to verify that infection itself does not affect HI* | Interpopulation crosses using infected ♀ and uninfected ♂ (incl. intrapopulation controls) | Ri1 × Ru2 or Gu Ri2 × Ru1 or Gu Gi × Ru1 or Ru2 (Ri1 × Ru1, Ri2 × Ru2, Gi × Gu) |
Ten days prior to the onset of the experiment (day −10), age cohorts were created for each infected and uninfected population, by allowing three groups of 100 mated females (i.e., “female cohorts”) and four groups of 25 virgin females (i.e., “male cohorts”) to lay eggs during 3 days on detached bean leaves placed on water-soaked cotton. Eight days later (day −2), female nymphs undergoing their last moulting stage (“quiescent females” hereafter) were randomly collected from each female cohort and placed separately on bean leaf fragments (ca. 9 cm2) to obtain virgin adult females with similar age. Virgin males used in the experiment were directly obtained from the male cohorts. On the first day of the experiment (day 0), one virgin female and one virgin male were installed together on 2.5 cm2 bean leaf discs for 3 days before being discarded (day 3). The number of unhatched eggs was counted 5 days later (day 8), and the numbers of dead juveniles, adult males and females were counted 12 days later (day 15). Raw data obtained in this experiment are presented in the Supporting Information Fig. S1.
The experiment was conducted in a growth chamber with standard conditions (24 ± 2°C, 60% RH, 16/8 h L/D). Most types of crosses were performed simultaneously, each with 50 independent replicates distributed within two experimental blocks performed one day apart (i.e., 25 replicates per block). However, given the high number of possible types of crosses (i.e., 36 combinations) and associated workload, the crosses of category E (Test for HI only, using infected females and uninfected males) were performed ca. 23 months later with minor differences in the methodology (cf. details in Supporting information Box S2). Therefore, data obtained with this latter category were analyzed separately and are provided in the Supporting information Table S1, Figs. S2 and S3.
To calculate the overproduction of F1 males in the brood (MD-type incompatibility; Breeuwer and Werren 1990; Navajas et al. 2000; Vala et al. 2000; Vavre et al. 2001), the embryonic mortality of fertilized offspring (i.e., only females in haplodiploids, hence, FM-type incompatibility; Vavre et al. 2000; Vala et al. 2002; Gotoh et al. 2007; Suh et al. 2015; Zélé et al. 2020b), and to control for potential incompatibilities affecting juvenile viability, we used indexes adapted from Poinsot et al. (1998; see also Cattel et al. 2018; Zélé et al. 2020b). These indexes allow computing the proportion of offspring potentially affected by each type of incompatibility (proportion of sons for MD-type incompatibility, embryonic death of daughters for FM-type incompatibility, and dead juveniles in the brood for juvenile inviability) relative to the control crosses, thereby accounting for background variation.
For the FM-type incompatibility index (FMcorr), FMobs = number of unhatched eggs/[number of unhatched eggs + number of F1 females], and CCFM is the mean embryonic mortality observed in the control crosses (i.e., the average of FMobs from control crosses, hence, correcting for variation in background embryonic mortality of F1 females). Moreover, to avoid biases arising from very low numbers of F1 females produced in some interpopulation crosses due to MD-type incompatibilities (cf. above and results), all females that produced less than two daughters were removed from statistical analyses of FMcorr (cf. final sample sizes in Supporting information Table S1).
For the juvenile inviability index (JMcorr), JMobs = number of dead juveniles/total number of eggs, and CCJM the mean juvenile mortality observed in control crosses (i.e., the average of JMobs from control crosses, hence, correcting for variation in background juvenile mortality of both F1 males and females).
EXPERIMENT 2: F1 FERTILITY AND F2 VIABILITY
To assess the fertility of F1 offspring obtained from interpopulation crosses and potential inviability of F2 offspring (i.e., hybrid breakdown; de Boer 1982b; Vala et al. 2000; Sugasawa et al. 2002), all crosses performed in Experiment 1, except those involving Ru2 and Ri2 (because they yielded results similar to Ru1 and Ri1), were repeated under mass-mating to obtain large numbers of individuals 13 days prior to the onset of the experiment (day −13). For each cross, 100 virgin females were placed with 100 males (obtained from age cohorts as described for Experiment 1) on an entire bean leaf to produce F1 offspring of the same age. These offspring were used separately to test for F1 female fertility and viability of their offspring (test 1 below) and F1 male fertility and viability of their offspring (test 2 below).
The experiment was conducted in a growth chamber with standard conditions (24 ± 2°C, 16/8 h L/D). In the first test of experiment 2, F1 females from all types of cross were tested simultaneously within four experimental blocks (with a maximum of 25 females per cross tested in each block), while in the second test, uninfected and infected F1 males (i.e., sons of uninfected or infected females, respectively, independently of the male that mated with these females) were tested (and thus analyzed) separately. Uninfected F1 males were tested within three experimental blocks (with a maximum of 30 males per cross tested in each block); and infected F1 males within two experimental blocks (with a maximum of 24 males per cross tested in each block). The number of replicates in each test was limited to the number of F1 offspring that could be obtained from the mass-mating crosses (cf. final sample sizes in Supporting information Table S2). Raw data obtained in this experiment are presented in Supporting information Fig. S4.
Test 1: F1 female fertility and F2 viability
Quiescent F1 females were collected from each mass-mating cross and installed on 9 cm2 bean leaf fragments 2 days prior to the beginning of the experiment (day −2) to emerge as adults while remaining virgin. They were then isolated on 2.5 cm2 bean leaf discs on the first experimental day (day 0), and allowed to lay eggs for 4 days, after which they were discarded and the number of eggs laid was counted (day 4). The number of unhatched eggs was counted 5 days later (day 9), and the number of dead juveniles and adult males was counted 12 days later (day 16; as mothers were virgin, they could only produce sons).
As F1 female fertility corresponds to their ability to lay a normal number of eggs (Navajas et al. 2000), we estimated both the proportion of ovipositing females and the daily oviposition of these females, taking into account their daily mortality (i.e., total number of eggs laid by each female/total number of days each female was alive). Hybrid breakdown was assessed as male embryonic and juvenile mortality accounting for variation in background mortality (i.e., not related to hybrid breakdown). The corresponding mEMcorr and mJMcorr indexes were calculated with the same general formula used in Experiment 1.
For the male embryonic mortality index (mEMcorr), mEMobs = number of unhatched eggs/total number of eggs, and CCmEM is the mean embryonic mortality observed in control crosses (i.e., the average of mEMobs from control crosses, hence, correcting for variation in background embryonic mortality of F2 males).
For the male juvenile mortality index (mJMcorr), mJMobs = number of dead juveniles/total number of eggs, and CCmJM is the mean juvenile mortality observed in control crosses (i.e., the average of mJMobs from control crosses, hence, correcting for variation in background juvenile mortaity of F2 males).
Test 2: F1 male fertility and F2 viability
In haplodiploid species, sons inherit a single chromosome from each maternal chromosome pair. Thus, in absence of reproductive anomalies, they should be fully compatible with females from their maternal population. However, because MD-type incompatibilities may also lead to the production of aneuploid males, such backcrosses could result in inviable offspring . To test this, adult F1 males were collected from each mass-mating cross and placed on 9 cm2 bean leaf fragments 2 days prior to the beginning of experiment (day −2) to avoid sperm depletion. On the first experimental day (day 0), each male was installed with one virgin female (obtained from age cohorts created as in Experiment 1) from the same population as its mother on a 2.5 cm2 bean leaf disc. Four days were given for the individuals to mate and for the females to lay eggs before both males and females were discarded (day 4). The number of unhatched eggs was counted 5 days later (day 9), and the numbers of dead juveniles, adult males and adult females were counted 12 days later (day 16).
As F1 male fertility corresponds to their ability to sire a normal proportion of offspring (i.e., F2 females), we estimated both the proportion of males siring daughter(s) and the sex ratio (here calculated as the ratio of females to males because haploid males only sire daughters) in the adult offspring of the females they mated with. Hybrid breakdown was assessed as F2 female embryonic and juvenile mortality accounting for variation in background mortality. Indexes were thus calculated as above.
For the F2 female embryonic mortality index (fEMcorr), fEMobs = number of unhatched eggs/[number of unhatched eggs + number of F2 females] and CCfEM is the mean embryonic mortality observed in control crosses (i.e., the average of fEMobs from control crosses, hence, correcting for variation in background embryonic mortality of F2 females).
For the F2 female juvenile mortality index (fJMcorr), fJMobs = number of dead juveniles/[number of dead juveniles + number of F2 females] and CCfJM is the mean juvenile mortality observed in control crosses (i.e., the average of fJMobs from control crosses, hence, correcting for variation in background juvenile mortality of F2 females).
STATISTICAL ANALYSES
Results
F1 OFFSPRING PRODUCTION AND VIABILITY
Reciprocal crosses between naturally Wolbachia-infected or Wolbachia-free populations of the red (Ri1, Ri2, Ru1 and Ru2) and green (Gi and Gu) forms of T. urticae allowed testing for the effects of Wolbachia-induced CI only, HI only, and the combined effect of both sources of incompatibility (cf. Methods and Table 1). Overall, we found no significant differences in juvenile mortality among crosses (see Fig. 1, Supporting information Tables S1 and S3), but ample variation in embryonic mortality (i.e., proportion of unhatched eggs) and/or in male production, both leading to an important decrease in female production (Fig. 1 and Supporting information Fig. S2). To identify the sources of such variation (i.e., Wolbachia-induced CI and/or HI), we determined which crosses were affected by MD-type incompatibilities (male development; i.e., overproduction of males resulting from fertilization failure and/or paternal genome elimination) and by FM-type incompatibilities (female embryonic mortality resulting from paternal genome fragmentation). Then, we assessed the consequences of the two types of incompatibility for the resulting proportion of F1 hybrids (only females in haplodiploids).
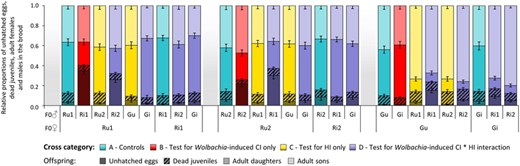
Summary of the development of T. urticae eggs resulting from intra- and interpopulation crosses between Wolbachia-infected and -uninfected mites. Bar plots represent mean ± S.E. relative proportions of unhatched eggs (i.e., embryonic mortality), dead juveniles (i.e., juvenile mortality), adult daughters and sons for each type of cross. Mothers are displayed on the bottom level of the x-axis and fathers on the top level. Note that crosses between infected females and uninfected males (cross category E; Supporting information Fig. S2) recapitulate the pattern observed in crosses between uninfected females and uninfected males (cross categories A and C).
Overproduction of males (MD-type incompatibility)
Overall, we found an overproduction of males (i.e., higher values of the MDcorr index; cf. Methods) in all interpopulation crosses involving females from the green-form population (ca. 46.4 to 64.3%) relative to all other crosses (ca. 5.6 to 21.5%; Main cross effect: χ226 = 460.70, P < 0.0001; model 1.1, Fig. 2A for crosses of categories A to D). Moreover, the level of MD-type incompatibility in these interpopulation crosses involving green-form females was not affected by Wolbachia infection (contrasts among all interpopulation crosses using Gu or Gi♂, regardless of Wolbachia infection in males: χ25 = 7.69, P = 0.17). In contrast, we found no overproduction of males in any of the interpopulation crosses involving red-form females (contrasts among all crosses with low MDcorr including the controls and regardless of Wolbachia infection in both males and females: χ220 = 26.11, P = 0.16). Finally, the analysis of crosses involving Wolbachia-infected females and uninfected males (i.e., crosses of category E; Supporting information Fig. S3A) recapitulated the pattern observed in crosses involving uninfected females and males (i.e., crosses of categories A and C), further showing that Wolbachia infection in females does not affect MDcorr. Indeed, as before, higher values of MDcorr were found for interpopulation crosses involving green-form females (ca. 57.9 to 64.5%) as compared to all other crosses (ca. 5.9 to 30.3%; Main cross effect: χ28 = 174.26, P < 0.0001; model 1.2; Supporting information Table S2). Taken together, these results revealed an overproduction of males due to HI between green-form females and red-form males, with Wolbachia infection playing no role in this outcome.
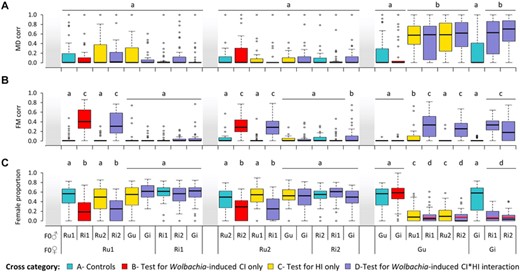
Overproduction of males, female embryonic mortality, and resulting hybrid production in intra- and interpopulation crosses using Wolbachia-infected and -uninfected mites. (A) Boxplot of the proportion of males produced in all crosses relative to that in control crosses (MDcorr). (B) Boxplot of the proportion of unhatched eggs relative to females, accounting for the basal level of this proportion observed in control crosses (FMcorr). (C) Proportion of F1 adult females (i.e., hybrids) in the brood. Horizontal red bars displayed within boxes for crosses affected by both MD- and FM-type incompatibilities indicate predicted values of female proportion (FPpred) under the assumption that the two types of incompatibilities have an independent effect on hybrid production. Mothers are displayed on the bottom level of the X-axis and fathers on the top level. Identical or absent superscripts above the boxes indicate nonsignificant differences at the 5% level among crosses. Note that crosses between infected females and uninfected males (cross category E; Supporting information Fig. S3) recapitulate the pattern observed in crosses between uninfected females and uninfected males (cross categories A and C).
Hybrid (female) embryonic mortality (FM-type incompatibility)
Overall, we found higher levels of female embryonic mortality relative to controls (FMcorr index; cf. Methods) in all crosses using Wolbachia-infected red-form males, either crossed with uninfected red-form females (as found by Zélé et al. 2020b), or with green-form females independently of their Wolbachia infection status (from 22.2 to 42.7% on average; Main cross effect: χ226 = 506.20, P < 0.0001; model 1.3; Fig. 2B). In addition, there were no significant differences among these crosses (χ27 = 8.76, P = 0.27; despite a tendency for Ri1 males to induce higher levels of FM-type CI than Ri2 males: 35 vs 29% on average), which shows that the Wolbachia strain infecting the green-form population did not rescue (even partially) CI induced by Wolbachia infection in red-form males. All other crosses resulted in no (or low) female embryonic mortality (from 0.2 to 10.5% on average; Contrasts among all these crosses with low FMcorr: χ216 = 19.99, P = 0.22). Thus, these results show that FM-type incompatibilities between populations are due to Wolbachia infection in males from the two red-form populations, and not to that in males from the green-form population, with the same penetrance in inter- and intrapopulation crosses (hence regardless of HI).
Consequences of MD- and FM-type incompatibilities for hybrid (female) production
As a result of the MD- and FM-type incompatibilities described above, we also found ample variation in the proportion of females produced across crosses (Main cross effect: χ226 = 966.45, P < 0.0001; model 1.7; Fig. 2C). Contrast analyses further revealed four statistically different proportions depending on the type of crosses: (1) ca. 51% daughters produced in compatible crosses (i.e., unaffected by both incompatibilities; Contrasts among these crosses: χ216 = 21.22, P = 0.17); (2) ca. 26% daughters produced in crosses affected by FM-type incompatibilities only (Contrasts among these crosses: χ23 = 2.98, P = 0.40; ca. 49% decrease compared to compatible crosses: χ21=187.67, P < 0.0001); (3) ca. 13% daughters produced in crosses affected by MD-type incompatibilities only (Contrasts among these crosses: χ21 = 0.04, P = 0.84; ca. 75% decrease compared to compatible crosses: χ21=292.02, P < 0.0001; and ca. 76% decrease when using crosses of category E, to test for HI using infected females and uninfected males: χ28 = 278.23, P < 0.0001; model 1.8; Supporting information Fig. S3C); and (4) ca. 9% daughters produced in crosses affected by both FM- and MD-type incompatibilities (Contrasts among these crosses: χ23 = 3.57, P = 0.31; ca. 82% decrease compared to compatible crosses: χ21 = 606.40, P < 0.0001).
Both types of incompatibility appeared to have lower consequences on hybrid production when combined than when acting alone. Indeed, we found around 31% decrease in hybrid production due to FM-type incompatibility when comparing groups (3) and (4) (crosses affected by MD-type incompatibilities only vs crosses affected by both FM- and MD-type incompatibilities; χ21 = 7.49, P = 0.03) and close to 65% decrease in hybrid production due to MD-type incompatibility when comparing groups (2) and (4) (crosses affected by FM-type incompatibilities only vs crosses affected by both FM- and MD-type incompatibilities; χ21 = 141.97, P < 0.0001). However, this was only a consequence of their cumulative effects. Indeed, we found a perfect fit between the observed and the predicted proportions of F1 females for crosses affected by both MD- and FM-type incompatibilities, calculated assuming that both affect hybrid production with the same strength when acting either alone or combined (Fig. 2C; goodness-of-fit test: Gu♀xRi1♂: χ247 = 14.30, P = 1; Gu♀xRi2♂: χ247 = 8.46, P = 1; Gi♀xRi1♂: χ247 = 13.90, P = 1; and Gi♀xRi2♂: χ248 = 7.37, P = 1). Thus, these results show that MD- and FM-type incompatibilities, here due to HI and Wolbachia-induced CI, respectively (see above), are independent, so that their effects are additive, with the former contributing 1.5 times more to reducing hybrid production than the latter (ca. 75 and 49% less hybrids produced, respectively).
F1 OFFSPRING FERTILITY AND VIABILITY OF THE F2
To estimate the effects of Wolbachia-induced CI and HI on the fitness of F1 offspring obtained from all aforementioned crosses (except those involving Ru2 and Ri2 populations, cf. Methods), we assessed the fertility of virgin F1 females and of F1 males backcrossed to females from their maternal population, and both embryonic and juvenile mortality of the resulting F2 offspring (i.e., hybrid breakdown; de Boer 1982b; Sugasawa et al. 2002).
Fertility of F1 females and viability of their offspring (Test 1)
The proportion of virgin F1 females that laid at least 1 egg differed significantly depending on the crosses they resulted from (χ215 = 214.26, P < 0.0001; model 2.1; Fig. 3A). While most females resulting from all intrapopulation crosses oviposited (ca. 96% on average; Contrasts among intrapopulation crosses; χ27 = 8.42, P = 0.30), more than 99% of those resulting from interpopulation crosses were unable to lay eggs. Moreover, although six hybrid females (over a total of 760), all resulting from crosses between males and females with the same Wolbachia infection status (either both infected, or both uninfected), were found to be fertile, they laid very few eggs (average daily oviposition of 0.63 ± 0.15 compared to 6.37 ± 0.09 for females resulting from intrapopulation crosses; cf. Supporting information Table S3), with no significant difference among interpopulation crosses (Contrasts among interpopulation crosses; χ27 = 8.59, P = 0.28).
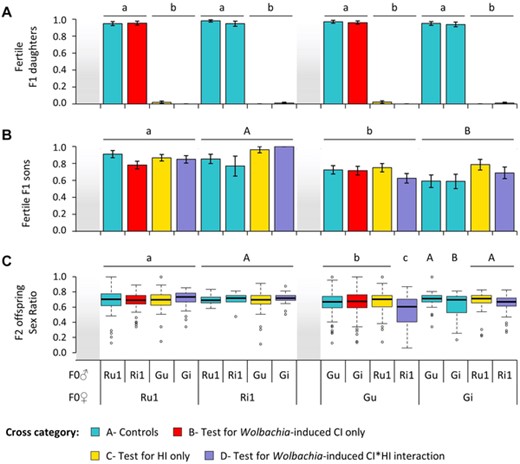
Proportion of fertile F1 female and male offspring resulting from intra- and interpopulation crosses using Wolbachia-infected and -uninfected mites, and sex-ratio of F2 offspring resulting from backcrosses of F1 males. Average proportion (± S.E.) of (A) fertile F1 females (i.e., proportion of females laying at least 1 egg) and (B) fertile F1 males (i.e., proportion of males siring at least 1 daughter when mated with a female from the same population as their mother). (C) Boxplot of sex ratio (daughters to sons) of F2 offspring sired by F1 males. Mothers are displayed on the bottom level of the X-axis and fathers on the top level. Identical or absent superscripts above bars or boxes indicate nonsignificant differences at the 5% level among crosses.
None of the few eggs laid (15 in total) by the six fertile hybrid females hatched (Supporting information Table S3), which corresponds to full F2 hybrid breakdown. In contrast, embryonic mortality (mEMcorr) of eggs laid by F1 females resulting from intrapopulation crosses was very low (ca. 5%), with only a very small increased mortality (ca. 2%) in the brood of F1 females from the Wolbachia-infected green-form population (Main cross effect: χ27 = 23.33, P = 0.001; model 2.3; Supporting information Fig. S5A). Similarly, juvenile mortality (mJMcorr) in the offspring (i.e., all F2 males) of virgin F1 females resulting from intrapopulation crosses was very low (below ca. 6%), and varied slightly depending only on their maternal origin (Main cross effect: χ27 = 18.57, P = 0.01; model 2.4; Supporting information Fig. S5B). Indeed, the offspring of infected green F1 females had higher juvenile mortality than the offspring of infected red-form females (independently of their grandfather; Contrasts between Gi and Ri1 females: χ21 = 12.53, P = 0.002), and the offspring of all uninfected F1 females displayed an intermediate mortality (Contrasts between Gu-Ru1 and Gi females: χ21 = 4.28, P = 0.17; Contrasts between Gu-Ru1 and Ri1 females: χ21 = 4.49, P = 0.17). These results thus show that, due to very high hybrid sterility (99% nonovipositing females) and complete hybrid breakdown, the red- and green-form populations studied here are, in fact, fully postzygotically isolated (i.e., no gene flow).
Fertility of F1 males and viability of their offspring (Test 2)
The proportion of F1 males siring at least one daughter (when backcrossed with a female from their maternal population) differed significantly depending on the crosses they resulted from (χ27 = 25.58, P < 0.001; model 2.5.1, and χ27 = 15.23, P = 0.03; model 2.5.2, for uninfected and infected males, respectively). However, this difference was mainly attributable to the maternal populations of these males and/or to the population of the females they mated with (i.e., as both are the same, it is not possible to disentangle their effects). Indeed, F1 males that mated with (and are sons of) green-form females were less fertile than those that mated with (and are sons of) red-form females (ca. 17.39 and 25.97%, for uninfected and infected males, respectively; cf. Fig. 3B).
The maternal population of fertile F1 males also affected the proportion of daughters they sired, but only when they were uninfected by Wolbachia (χ27 = 42.10, P < 0.0001; model 2.8.1; Fig. 3C). In this case, we found that F1 males that mated with (and are sons of) red-form females sired on average more offspring (ca. 69%) than F1 males that mated with (and are sons of) green-form females (ca. 55% for those mated with infected red-form males; χ21 = 32.13, P < 0.0001; and ca. 65% for those mated with all other males; χ21 = 8.96, P = 0.008). We also found some differences in the sex-ratio of offspring resulting from crosses using infected F1 males (χ27 = 15.19, P = 0.03; model 2.8.2), but this effect was only due to a higher variance (but not median) in the Gi♀xGi♂ control cross, and no difference was found among all other crosses (χ26 = 9.93, P = 0.13; Fig. 3C).
Finally, neither embryonic mortality (fEMcorr) nor juvenile mortality (fJMcorr) varied among the offspring of infected F1 males (Main cross effect on fEMcorr: χ27 = 5.58, P = 0.59; model 2.6.2, and on fJMcorr: χ27 = 11.68, P = 0.11; model 2.7.2). Although both varied among the offspring of uninfected F1 males (Main cross effect on fEMcorr: χ27 = 26.31, P < 0.001; model 2.6.1, and on fJMcorr: χ27 = 22.64, P = 0.002; model 2.7.1), this variation is not attributable to Wolbachia-induced CI or HI. Indeed, a higher embryonic mortality (ca. 7% on average) was found only in the offspring of uninfected F1 males that mated with (and are sons of) green-form females compared to those that mated with (and are sons of) red-form females (ca. 4% in average). In line with this, we found that juvenile mortality varied depending on both the maternal and the paternal populations of uninfected F1 males (or the females they mated with), but regardless of incompatibility (due to Wolbachia-induced CI and/or HI) between the parental populations (see Supporting information Fig. S6B).
Overall, these results indicate that F1 males resulting from all types of incompatible crosses do not suffer a reduction in fertility. This suggests that they are true hemiclones of their mother, thereby escaping both sources of incompatibility (Wolbachia-induced CI and HI).
Discussion
Using three populations of the two genetically differentiated colour forms of T. urticae, each naturally infected or cured from Wolbachia, we assessed the relative contribution of Wolbachia-induced CI and of host-associated incompatibilities (HI) to postmating isolation. Our results revealed that both sources of incompatibility reduced the production of F1 hybrid females in the same direction, albeit through different and independent mechanisms, and with HI contributing ca. 1.5 times more than Wolbachia-induced CI to this reduction. While HI led to ca. 75% fewer F1 hybrids and was associated with an increased production of males in crosses between green-form females and red-form males of either population, Wolbachia-induced CI led to ca. 49% fewer F1 hybrids and was due to an increased female embryonic mortality in crosses involving red-infected males (mated with uninfected females from any of the tested populations, or infected green females). Additionally, we found a Wolbachia-independent near-complete F1 hybrid female sterility and full F2 hybrid breakdown in all reciprocal crosses between the green- and the red-form populations.
EXPRESSION OF HOST-ASSOCIATED INCOMPATIBILITIES
Crosses among uninfected host populations in absence of Wolbachia infection confirmed that the two populations belonging to the red form of T. urticae were mutually compatible (Zélé et al. 2020b), but were fully isolated from the green-form population. We found three different types of postmating reproductive barriers between these populations: (1) a sharp and unidirectional (between females from the green-form population and males from the red-form populations but not in reciprocal crosses) reduction in F1 hybrid (female) production, concurrent with an increased production of F1 males (i.e., MD-type incompatibility); (2) near-complete F1 hybrid sterility (>99%) in all reciprocal crosses between the red and the green-form population; and (3) full F2 hybrid breakdown, as none of the few eggs produced by F1 hybrid females hatched.
MD-type incompatibilities, which result in an excess of F1 males at the expense of daughters, have already been reported between populations from the two colour forms of T. urticae (Murtaugh and Wrensch 1978; Sugasawa et al. 2002; Lu et al. 2017), as well as among populations of the same colour form (Navajas et al. 2000; Perrot-Minnot et al. 2004). In haplodiploids, this type of incompatibility can result from either fertilization failure or haploidization of fertilized F1 eggs. Partial haploidization of fertilized eggs is unlikely here, as males surviving such defect would be aneuploid, and thus, should produce fewer daughters, an outcome we did not find when testing F1 males. Moreover, there is yet no evidence that aneuploid embryos can actually be viable in spider mites. Conversely, complete haploidization of fertilized eggs is a plausible explanation, as Wolbachia can cause MD-type incompatibility in T. urticae (Vala et al. 2000; Perrot-Minnot et al. 2002; Gotoh et al. 2003), and this outcome was shown to result from paternal genome elimination following fertilization in haplodiploids (i.e., it restores haploidy and, thus, leads to the production of viable males; Breeuwer and Werren 1990; Tram et al. 2006). However, in spider mites, males are naturally produced from unfertilized eggs (i.e., arrhenotoky; Helle and Bolland 1967) and not from the elimination of the paternal genome in fertilized eggs (i.e., pseudoarrhenotoky; Nelson-Rees et al. 1980; Sabelis and Nagelkerke 1988). Therefore, fertilization failure resulting from a defect at any of the successive stages of the reproductive process in the female reproductive tract is another possible explanation for this type of incompatibility between populations (e.g., reduction in sperm transfer/storage, sperm ejection/dumping, reduced sperm activation or attraction to the egg, and sperm-egg incompatibility; Zeh and Zeh 1997; see also Takafuji and Fujimoto 1985; Perrot-Minnot et al. 2004). Moreover, although the results presented here do show that premating isolation between the two forms is incomplete (i.e., no hybrids would be produced in absence of mating), we cannot exclude the possibility that fewer copulations have occurred in these crosses. Only direct observations of copulations, of the fertilization process, and of early embryogenesis of the offspring in these crosses would allow testing these hypotheses.
Irrespective of the underlying mechanisms, we found both asymmetric (MD-type) and symmetric (F1 hybrid sterility and F2 hybrid breakdown) patterns of reproductive incompatibilities between spider mite populations of the two forms. In general, asymmetric incompatibilities have been tied to “Bateson-Dobzhansky-Muller incompatibilities” (BDMIs–negative epistatic interactions between alleles from independently evolving lineages) between autosomal loci and uniparentally inherited factors (e.g., maternal transcripts; Sawamura 1996; Turelli and Orr 2000; or cytoplasmic elements such as mitochondrial genes; Burton and Barreto 2012). In contrast, symmetrical patterns of incompatibilities are generally associated to BDMIs between nuclear genes inherited from both parents (Turelli and Moyle 2007). This suggests that MD-type incompatibilities are caused by cytonuclear interactions, whereas hybrid sterility and hybrid breakdown are mainly due to incompatibilities between nuclear genes. This is in line with some evidence from previous work using spider mites, albeit several of these studies also highlight a role for cytonuclear interactions in hybrid sterility and hybrid breakdown (Overmeer and van Zon 1976; de Boer 1982b; Fry 1989; Sugasawa et al. 2002; Perrot-Minnot et al. 2004).
EXPRESSION OF WOLBACHIA-INDUCED CI WITHIN AND AMONG POPULATIONS
Crosses between Wolbachia-infected males and uninfected females within and among populations showed that the Wolbachia strains infecting the two red-form populations induce imperfect FM-type incompatibility (ca. 22 to 43% female embryonic mortality) and are mutually compatible (as found by Zélé et al. 2020b). Here, we further showed that Wolbachia-induced CI has the same penetrance within and among host populations including the population from the green form. Conversely, the strain infecting the green-form population did not induce CI within or between populations, neither of the FM-type nor of the MD-type. Moreover, in contrast to some other non-CI-inducing Wolbachia strains in T. urticae (Vala et al. 2002), this strain did not rescue the CI induced by the strain infecting the red-form populations. Taken together, these results show a unidirectional pattern of Wolbachia-induced CI, which reduces hybrid production between the Wolbachia-infected red-form populations and the green-form population, regardless of Wolbachia infection in the latter. Finally, we found no evidence for hybrid breakdown (i.e., increased mortality of F2 offspring produced by F1 females escaping Wolbachia-induced CI) by any of the Wolbachia strains, suggesting that such effect is not a common feature in spider mites, or that it is restricted to strains inducing MD-type incompatibilities (Vala et al. 2000).
THE COMBINED EFFECTS OF INCOMPATIBILITY TYPES FOR HYBRID PRODUCTION AND GENE FLOW
In some systems, Wolbachia-induced CI may play a greater role than HI in reducing gene flow between hosts. For instance, complete postmating isolation due to bidirectional CI induced by Wolbachia has been found in interspecific crosses between the mosquitoes Aedes polyniensis and Aedes riversi (Dean and Dobson 2004), and between the parasitoid wasps Nasonia giraulti and Nasonia vitripennis (Breeuwer and Werren 1990, 1995), while only partial isolation was found in interspecific crosses upon Wolbachia removal (asymmetrical hybrid production and F2 hybrid breakdown, respectively). In other systems, however, CI induced by symbionts and host intrinsic factors can complement each other when acting in opposite directions, as found between Encarsia gennaroi and Cardinium-infected E. suzannae (Gebiola et al. 2016), or can act synergistically to reduce gene flow in the same direction. This was found between some populations of the spider mite P. mori, where Wolbachia-induced CI mainly results in haploidization of fertilized eggs and can increase existing MD-type incompatibilities between populations (Gotoh et al. 2005). However, the relative contribution of Wolbachia-induced CI and HI to postmating isolation was not quantified in such cases, let alone whether they have additive or interacting effects.
In our system, we found that both HI and Wolbachia-induced CI act to prevent the production of F1 hybrid offspring in crosses between green-form females and red-form males. Moreover, we showed that they act independently and additively, with HI contributing c.a. 1.5 times more than Wolbachia-induced CI to the reduction in F1 hybrid production. However, because all F1 hybrids were either sterile or produced inviable eggs, Wolbachia did not affect gene flow between the red- and green-form populations studied here. Nonetheless, these results suggest that CI induced by Wolbachia may have an important role in restricting gene flow between populations that are only partially isolated, which is a common phenomenon in T. urticae (e.g., Dupont 1979; Fry 1989; Navajas et al. 2000; Sugasawa et al. 2002; Perrot-Minnot et al. 2004). In particular, the effects of CI induced by Wolbachia may be considerable when MD-type incompatibilities between hosts are weaker (Murtaugh and Wrensch 1978; Navajas et al. 2000; Sugasawa et al. 2002), and/or when the two types of incompatibilities act in opposite directions (e.g., as found in Cardinium-infected Encarsia parasitoid wasps; Gebiola et al. 2016). Therefore, more studies using population pairs with variable degrees of postmating isolation, and assessing premating isolation, are needed to better understand the extent to which Wolbachia can hamper gene flow between natural populations of spider mites, and determine its exact role in the speciation processes currently ongoing in this system.
ECOLOGICAL IMPLICATIONS OF HOST-ASSOCIATED AND WOLBACHIA-INDUCED INCOMPATIBILITIES
Our results show strong reproductive interference (see Gröning and Hochkirch 2008; Burdfield-Steel and Shuker 2011) between the populations from the two forms of T. urticae used in our study, which may potentially impact their dynamics by favouring the green-form population. Indeed, green-form females mated with red-form males produce less (sterile) hybrid daughters but more (fertile) sons than red-form females mated with green-form males, and this overproduction of sons may have important consequences for the persistence of these populations. Moreover, despite our finding that F1 green-form males had a slightly lower fitness than F1 red-form males (i.e., lower fertility and higher embryonic mortality of their daughters), their overproduction should allow green-form females to transmit more genes (thereby mitigating the costs of heterospecific matings; Feldhaar et al. 2008). This should also increase, at the next generation, the probability of conspecific matings (e.g., as in Callosobruchus beetles; Kyogoku and Nishida 2012) for green-form females, and of heterospecific matings for red-form females, which may again favour the green-form population.
Wolbachia may also affect the balance of the interactions between these populations, both due to the direct effects of infection on host fitness (i.e., Wolbachia slightly increases the embryonic and juvenile mortality of F2 sons of green-form, but not red-form, F1 females), but also due to CI. Indeed, although CI induced by Wolbachia leads to embryonic mortality of hybrid daughters of green-form females, these daughters are sterile. Conversely, Wolbachia-induced CI leads to embryonic mortality of fertile daughters of red-form females, which may further disadvantage red-form females in populations that are polymorphic for Wolbachia infection (as often found in spider mites; Breeuwer and Jacobs 1996; Zhang et al. 2013; Zélé et al. 2018a). Note, however, that the effect of Wolbachia-induced CI between partially isolated populations of the two forms (e.g., de Boer 1982b; Sugasawa et al. 2002) may lead to different scenarios, as it could also affect fertile hybrid daughters produced by females of either form.
Such scenarios are likely to occur in natural populations of T. urticae, as incompatible populations (both of the same and of different colour forms) often co-occur in the field (Helle and Pieterse 1965; Lu et al. 2017), and the populations used in this study were collected in the same geographical area (cf. Supporting information Box S1). However, these scenarios will also depend on the strength and the symmetry of premating and postmating prezygotic reproductive barriers between populations (Sato et al. 2015, 2018; Gebiola et al. 2017; Clemente et al. 2018). Indeed, although one study reported no assortative mating between the colour forms of T. urticae (Murtaugh and Wrensch 1978), this may vary between populations, as found between T. urticae and T. evansi (Sato et al. 2014; Clemente et al. 2016). In line with this, contrasting results were found concerning the effect of Wolbachia on spider mite mating behavior (Vala et al. 2004; Rodrigues et al. 2018). Thus, to understand the implications of reproductive interference in this system, future studies should focus on prezygotic isolation between T. urticae populations, infected or not by Wolbachia.
Conclusions
Our results show that both host-associated and Wolbachia-induced incompatibilities in this system contribute to reducing hybridization between populations of the two T. urticae colour forms, the former via an overproduction of sons and the latter via embryonic mortality of daughters. Furthermore, these two types of incompatibility have additive effects in the same direction of crosses, which hints at a possible role of Wolbachia-induced incompatibilities in host population divergence and subsequent evolution of intrinsic reproductive barriers (e.g., as found in the Nasonia wasps; Bordenstein et al. 2001). Indeed, although the level of divergence between the populations studied here limits our understanding of the contribution by Wolbachia in this system (because they are either not or fully isolated), our results suggest that this reproductive manipulator may have a considerable effect between partially isolated populations and, thus, could play an important role in the processes of speciation currently ongoing in spider mites. Finally, our results raise important questions about the ecological consequences of Wolbachia-driven reproductive interference in arthropods, and call for further studies to understand its impact on the dynamics and distribution of natural populations from the same species, but also from closely related species.
AUTHOR CONTRIBUTIONS
Experimental conception and design of the first experiment: FZ with discussions with SM. Experimental conception and design of the second experiment: MC, FZ, SM, ES; Acquisition of data, statistical analyses, and writing of the first version of the manuscript: MC, FZ. Subsequent versions were written with input from SM and ES. Funding agencies did not participate in the design or analysis of experiments. All authors have approved the final version for publication.
ACKNOWLEDGMENTS
This work was funded by an FCT-ANR project (FCT-ANR//BIA- EVF/0013/2012) to SM and Isabelle Olivieri and by an ERC Consolidator Grant (COMPCON, GA 725419) to SM. MC was funded through an FCT PhD fellowship (SFRH/BD/136454/2018), and FZ through an FCT Post-Doc fellowship (SFRH/BPD/125020/2016) when performing the experiments. This is publication ISEM-2021-150 of the Institut des Sciences de l'Evolution. We are grateful to Salomé Clemente and Leonor Rodrigues for their help in collecting data for the first experiment, to Inês Santos for the maintenance of the spider mite populations and the plants, and to Inês Fragata and Fabrice Vavre for useful discussions and suggestions. This manuscript has been peer-reviewed and recommended by Peer Community In Evolutionary Biology (https://doi.org/10.24072/pci.evolbiol.100116), and we are very grateful to Jan Engelstädter, Wolfgang Miller and one anonymous reviewer for their helpful comments and suggestions. Finally, we are also very grateful to Gregory Hurst and three anonymous reviewers for helpful comments that improved the quality of this last version of the manuscript.
CONFLICT OF INTEREST
The authors declare that they have no conflict of interest with the content of this article.
DATA ARCHIVING
Full datasets are available in the Dryad data repository (https://doi.org/10.5061/dryad.3r2280gg5).
LITERATURE CITED
Associate Editor: T. Chapman
Handling Editor: G. D. Hurst