-
PDF
- Split View
-
Views
-
Cite
Cite
Tanmay Dixit, Eleanor M. Caves, Claire N. Spottiswoode, Nicholas P. C. Horrocks, Why and how to apply Weber's Law to coevolution and mimicry, Evolution, Volume 75, Issue 8, 1 August 2021, Pages 1906–1919, https://doi.org/10.1111/evo.14290
- Share Icon Share
Abstract
In mimicry systems, receivers discriminate between the stimuli of models and mimics. Weber's Law of proportional processing states that receiver discrimination is based on proportional, not absolute, differences between stimuli. Weber's Law operates in a variety of taxa and modalities, yet it has largely been ignored in the context of mimicry, despite its potential relevance to whether receivers can discriminate models from mimics. Specifically, Weber's Law implies that for a given difference in stimulus magnitude between a model and mimic, as stimulus magnitudes increase, the mimic will be less discriminable from their model. This implies that mimics should benefit when stimulus magnitudes are high, and that high stimulus magnitudes will reduce selection for mimetic fidelity. Whether models benefit from high stimulus magnitudes depends on whether mimicry is honest or deceptive. We present four testable predictions about evolutionary trajectories of models and mimics based on this logic. We then provide a framework for testing whether receiver discrimination adheres to Weber's Law and illustrate it using coevolutionary examples and case studies from avian brood parasitism. We conclude that, when studying mimicry systems, researchers should consider whether receiver perception conforms to Weber's Law, because it could drive stimulus evolution in counterintuitive directions.
Imagine that you are a bird and must identify a parasitic egg in your nest, as hosts of cuckoos or other brood parasites might do. The brood parasite lays eggs that closely mimic your own, differing only by having an extra spot compared to your own spotted eggs. As illustrated in Figure 1, having more spots on your own eggs makes it harder to recognize the parasitic egg, even though in both scenarios the absolute difference in degree of spottiness between your eggs and the parasitic egg is the same.
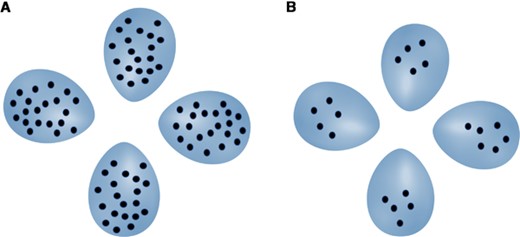
Two clutches of three host eggs and a parasitic egg. In each case, the parasitic egg (on the right in each clutch) has one more spot than each host egg. It is harder to identify the parasitic egg in panel A than in panel B, because the number of spots on each egg is higher in panel A. The parasite therefore benefits from situation (A), whereas the host benefits from situation (B). This is an illustration of Weber's Law, which states that differences between stimuli are less discriminable as stimulus magnitudes increase.
This example illustrates Weber's Law (Fechner 1966; Weber 1834), a principle of receiver perception that states that stimuli are processed proportionally rather than being compared in absolute terms. As Figure 1 shows, it is not the absolute difference between the number of spots on the host eggs and the parasitic egg that affects our judgment of the difference, as for both clutches this difference is one spot. Instead, we process the relative difference, known as “proportional processing.” For the clutch with five spots per egg (Fig. 1B), the relative difference in number of spots between host and parasitic eggs is , whereas for the clutch with 20 spots per egg (Fig. 1A), it is only . The higher magnitude of the stimulus in the latter case (more spots per egg) results in the same absolute difference in pattern between parasitic egg and host eggs being less noticeable. If, such as humans, hosts also process relative differences in spot number, then they benefit when their eggs have few spots, whereas parasites benefit when both host and parasitic eggs have many spots (Fig. 1A; see also Section 1). Rather than simply being a quirk of receiver perception, therefore, Weber's Law can have important consequences for the optimal strategies that natural selection should favor, and by extension the evolutionary trajectories of co-evolving species, as we will discuss in this article.
Such proportional, or nonlinear, processing may take other forms, such that the qualitative statement that “discriminability declines as stimulus magnitude increases” remains true, yet discriminability differs slightly from the precise relationship described by equation 1. This is true for the so-called “near-miss” to Weber's Law (where discriminability declines less sharply with stimulus magnitude than under Weber's Law; Augustin 2009; Guilford 1932; Nachev et al. 2013; Osman et al. 1980) and the “opposite-miss” to Weber's Law (where discriminability declines more sharply with stimulus magnitude than under Weber's Law; LaBarbera et al. 2020). Therefore, any effect on reduced discriminability of an increase in stimulus magnitudes will be stronger than Weber's Law under an opposite-miss scenario, and weaker than Weber's Law under a near-miss scenario. For clarity, we specifically discuss Weber's Law in this article; however, our arguments should be considered to apply to proportional processing (including the near-miss and opposite-miss scenarios) more broadly. We therefore use the terms “Weber's Law” and “proportional processing” interchangeably.
In a biological context, many behaviors fundamental to ecology and evolution involve discrimination between stimuli of the same type but differing in magnitude. There is evidence from a wide variety of taxa and ecological contexts that receivers discriminate such differences relative to the stimulus magnitude, perhaps because of how sensory cells convey information, and such that discrimination adheres to Weber's Law (Akre & Johnsen 2014). For example, discrimination between mating signals in frogs and prey cues in bats (Akre et al. 2011), group sizes in fish (Gómez-Laplaza & Gerlai 2011), quantities of food in coyotes (Baker et al. 2011), and pheromone concentrations in ants (Perna et al. 2012) have all been shown to follow Weber's Law. Weber's Law has also been explicitly incorporated into theories of group living, playing a role in group stability, social interactions, and group decision-making (Mann & Garnett 2015; Perna et al. 2019; Reina et al. 2018). Proportional processing by receivers can also influence the evolution of stimulus magnitudes. For example, lower quality or lower quantity floral nectars evolve when pollinating bats proportionally process quality or quantity, because bats are less able to discriminate quality or quantity as the magnitudes of these stimuli increase (Nachev et al. 2017). Similarly, Weber's Law has been suggested to limit the evolution of signals used in mate choice: because higher magnitude stimuli are more difficult to discriminate, larger or more elaborate male signals may have diminishing benefits in terms of attracting females (Akre et al. 2011; Akre & Johnsen 2014; Cohen 1984). This could result in selection for new signals to attract females (Akre & Johnsen 2014). Here, we extend this application of Weber's Law to signal evolution by considering signaling in coevolutionary interactions.
Despite its broad significance, Weber's law has not yet been explicitly incorporated into theoretical and empirical work on coevolution or mimicry (but see Speed 1999). Many coevolutionary systems, including parasite-host, predator-prey, and brood parasite-host systems, involve receivers discriminating mimics from models by detecting differences in the magnitude of stimuli (Malcolm 1990), as illustrated by the example in Figure 1. Such systems are ideal for studying evolutionary consequences of Weber's Law, because receiver perception modulates selection on signals of mimics and their models (Guilford & Dawkins 1991). Many theoretical frameworks used in modelling the evolution of mimicry, such as signal detection theory, do not incorporate relative differences between the stimuli of models and mimics, but instead tend to focus solely on absolute differences (Oaten et al. 1975; Speed & Ruxton 2010). In doing so, they may overlook effects of Weber's Law.
Here, we discuss how Weber's Law applies to mimicry systems. Specifically, we outline four testable predictions describing how Weber's Law could affect the (co-)evolutionary trajectories of mimicry systems, with these effects depending in part on whether mimicry is honest or deceptive. We then propose a framework for testing whether receiver discrimination follows Weber's Law, and highlight common pitfalls to avoid. Throughout, we illustrate our arguments with examples, focusing particularly on avian brood parasite-host systems. These provide excellent case studies for discussing how to test Weber's Law, as we outline in the second half of this article.
Coevolution: A process in which pairs or groups of interacting species or populations reciprocally affect each other's evolution.
Cue: A structure or behavior that elicits a response in an unintended receiver; in contrast to signals, cues have not evolved under selection for this function.
Detection: The process of noticing the presence or absence of an object or the occurrence of an event. This can occur alongside recognition; for example, recognition of which specific object is the odd one out.
Discrimination: The process of distinguishing (by detection or recognition) different stimuli of the same type.
Just Noticeable Difference (JND): The smallest stimulus difference that can be discriminated.
Numerosity: The quantity of a specific entity. This is always a positive integer.
Receiver: An organism that processes and responds to a cue or signal.
Recognition: The identification, by discrimination, of a specific object.
Signal: A structure or behavior that has evolved under selection to elicit a response in a receiver.
Stimulus: A signal or cue processed by a sensory system of a receiver, which causes the receiver to respond in some salient way.
Stimulus difference: The difference in magnitude between two stimuli of the same type.
Stimulus magnitude: The size of a stimulus. Stimulus magnitudes are non-negative and must include zero.
Section 1: Implications of Weber's Law for mimicry systems
HONEST VERSUS DECEPTIVE MIMICRY
Many coevolutionary systems involve mimics evolving to resemble models. Mimicry systems can be divided into two general types: honest and deceptive, depending on the type of information signaled to a receiver (reviewed by Jamie 2017).
Honest mimicry is defined as mimicry in which the mimic honestly signals information to the receiver. In Müllerian mimicry, a fitness cost is honestly signaled to the receiver. This includes systems in which multiple unpalatable species evolve to resemble each other, because they benefit from the increased efficiency of predator learning (Sherratt 2008). For example, apheloriine millipedes honestly signal their toxicity to predators through aposematic coloration (Fig. 2A, B), with several species evolving to resemble each other (Marek & Bond 2009). In rewarding mimicry, a fitness benefit is honestly signaled to a receiver. For example, multiple plants with nectar rewards may evolve to resemble each other because this facilitates attraction of pollinators (Fig. 2C, D; Benitez-Vieyra et al. 2007; Jamie 2017; Schaefer & Ruxton 2009; Coetzee et al. 2021). In honest mimicry, the model may in turn evolve a similar phenotype to the mimic (Balogh & Leimar 2005; Chouteau et al. 2011; Holmgren & Enquist 1999; Sherratt 2008; but see Benitez-Vieyra et al. 2007; Mallet 1999), and the distinctions between “model” and “mimic” may become blurred as both evolve to resemble the other.
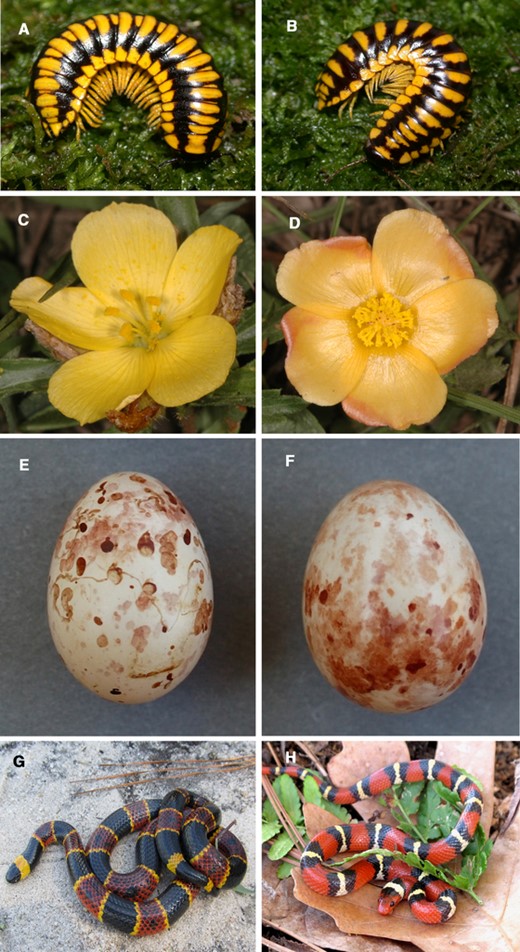
Four examples of mimicry systems, involving Müllerian, rewarding, aggressive, and Batesian mimicry, respectively. (A) Apheloria clade A and (B) Brachoria cedra are two unpalatable Müllerian mimetic millipedes. Apheloria clade A has a greater proportion of yellow aposematic coloration than B. cedra. (C) Turnera sidoides pinnatifida and (D) Modiolastrum malvifolium are flowers that demonstrate rewarding mimicry: both species benefit from increased visitation from pollinators due to their similarity. (E) Eggs of the tawny-flanked prinia Prinia subflava are the model for (F) eggs of its aggressive mimic, the brood-parasitic cuckoo finch Anomalospiza imberbis. Cuckoo finch eggs differ from prinia eggs in having larger markings on average (Spottiswoode & Stevens 2010). (G) The venomous Eastern coral snake Micrurus fulvius, a model for (H) its Batesian mimic, the nonvenomous scarlet kingsnake Lampropeltis elapsoides. The mimic differs from the model in that it exhibits a smaller area of black and a larger area of red. All photos reproduced with permission: panels A and B, Marek and Bond (2009); panels C and D, Andrea A. Cocucci; panels E and F, Claire N. Spottiswoode; panels G and H, David W. Pfennig.
Deceptive mimicry is defined as mimicry in which the mimic deceptively signals information to the receiver. In aggressive mimicry, a mimic advertises greater benefits than it provides to a receiver, by deceptively copying stimuli that convey these benefits (e.g., the presence of offspring or food). Aggressive mimicry systems include brood parasite-host systems, where parasites deceptively signal the presence of host offspring, often by mimicking egg phenotypes of their hosts (Fig. 2E, F). The model suffers costs from being mimicked, due to the erosion of signal reliability, and costs of being parasitized. Therefore, host phenotypes evolve toward increased discriminability from their parasites (e.g., Lahti 2005; Spottiswoode & Stevens 2012). In Batesian mimicry, a mimic advertises greater costs than it provides to a receiver by deceptively copying a model's stimuli that convey these greater costs, such as danger or unpalatability (Bates 1862). An example of Batesian mimicry is the aposematic color patterns of venomous Eastern coral snakes Micrurus fulvius (Fig. 2G) that are mimicked by nonvenomous scarlet kingsnakes Lampropeltis elapsoides (Fig. 2H), resulting in reduced predation on the latter due to predators avoiding the former (Pfennig et al. 2001). As with aggressive mimicry, models are predicted to benefit from evolving away from Batesian mimics, because mimicry erodes signal reliability, which could reduce the extent to which predators avoid the model (Fisher 1930). Empirical evidence for such “chase-away” dynamics has been found in aggressive mimicry systems (Lahti 2005; Spottiswoode & Stevens 2012) but is mixed in Batesian mimicry systems (Akcali et al. 2018; Kraemer et al. 2015; Rowland et al. 2010).
In both honest (Müllerian or rewarding) and deceptive (aggressive or Batesian) mimicry, models and mimics often differ from one another in the magnitude of certain stimuli, such that mimicry is imperfect (Kikuchi & Pfennig 2013; McLean et al. 2019; Sherratt 2002). This is true for the Müllerian, Batesian, and aggressive systems depicted in Figure 2. For example, in the case of coral snakes and kingsnakes, the areas of black and red markings differ between model (M. fulvius) and mimic (L. elapsoides) (Pfennig et al. 2007; Fig. 2G, H). These stimulus differences may be proportionally processed by receivers, leading to qualitatively different selection pressures on models that benefit from being resembled by their mimic (as may be the case in honest mimicry), and models which benefit from being discriminable from their mimic (as may be the case in deceptive mimicry), as we discuss next.
IMPLICATIONS OF WEBER'S LAW FOR HONEST MIMICRY
In honest mimicry, models benefit from reduced discriminability because the interests of model and mimic align: both benefit when the receiver cannot distinguish between them (indeed, the receiver benefits from this too). This means that models and mimics may converge toward very similar phenotypes. However, evolutionary constraints often mean that perfect mimicry is rare and so there may be further selection for reduced discriminability (Kikuchi & Pfennig 2013; Sherratt 2002). If Weber's Law applies to receiver perception, then both model and mimic should benefit from, and evolve toward, increased stimulus magnitudes (Fig. 3A, B). This is because Weber's Law states that a receiver should find discriminating between two stimuli of higher magnitude more difficult than discriminating between two stimuli of lower magnitude.
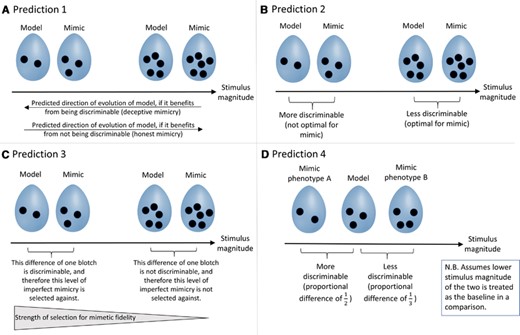
Weber's Law generates four predictions regarding the evolutionary trajectories and mimetic fidelity of mimicry systems, illustrated here using a hypothetical avian brood parasite-host system where receivers discriminate between eggs based on the spottiness of their shells. Weber's Law states that for higher stimulus magnitudes (i.e., more spots on an egg), a given absolute difference will be more difficult to discriminate. See main text for details and reasoning behind the predictions.
When stimulus magnitudes are high, reduced discriminability under Weber's Law could weaken selection for mimetic fidelity. This is because high-magnitude stimuli may be perceptually indistinguishable even when differing greatly in absolute magnitude (Fig. 3C). For example, the Müllerian co-mimetic millipedes Apheloria spp. and Brachoria spp. differ in the area of yellow aposematic color on their bodies (Marek & Bond 2009; Fig. 2A, B). If predators can discriminate between these species based on this difference, and if this stimulus is proportionally processed, then increasing the quantity of yellow should be beneficial to both species because it would make them less discriminable. As the quantity of yellow increases, even large absolute differences in this stimulus may be indistinguishable to predators, because they correspond to small relative differences. Therefore, large variation in mimetic fidelity may persist.
IMPLICATIONS OF WEBER'S LAW FOR DECEPTIVE MIMICRY
In contrast to models of honest mimics, models of deceptive mimics are under selection to be discriminable from their mimics (Fisher 1930; Holmgren & Enquist 1999; Lahti 2005; Nur 1970; Spottiswoode & Stevens 2012; but see Akcali et al. 2018). If receivers proportionally process the stimuli that differ between models and mimics, then Weber's Law predicts that models will evolve toward decreased stimulus magnitudes, such that the receiver can better discriminate them from their deceptive mimic, and such that increased mimetic fidelity would be required to trick the receiver. In the case of the snakes, M. fulvius and L. elapsoides (Fig. 2G, H), there is evidence that predators discriminate between model and Batesian mimic based on the area of colored markings (Harper & Pfennig 2007). If predators process, for instance, the area of red markings in a proportional manner, then Weber's Law predicts that the model should be selected to evolve decreased red coloration, because this would make it more discriminable from the mimic (Fig. 3A). Clearly, if predators proportionally processed the area of all three color markings (red, black, and yellow), models could not evolve decreased areas of all three colors simultaneously. In such bounded distributions, a decrease in the magnitude of one trait necessitates an increase in the magnitude of another. There may therefore be no benefit to evolving a lower magnitude of one stimulus, because this carries the cost of an increase in the magnitude of another stimulus, which under Weber's Law may reduce discriminability. If, however, receivers do not proportionally process all the traits making up the distribution (as may be the case if bright aposematic colors are processed preferentially to darker colors), models may benefit from evolving a reduced magnitude of the processed trait.
As with honest mimics, deceptive mimics benefit when stimulus magnitudes are elevated, such that their discriminability from models is lower (Fig. 3B). Therefore, unlike in honest mimicry systems, in deceptive mimicry systems the optimal stimulus magnitudes of model and mimic are in conflict with each other. Deceptive mimics are constrained to resemble models and must therefore “follow” the direction of model evolution (Holmgren & Enquist 1999; Nur 1970). This suggests that, in deceptive mimicry, the stimulus magnitudes of both model and mimic should evolve toward decreased magnitudes (Fig. 3A).
However, when mimicry is imperfect, under certain circumstances mimics may nevertheless evolve stimuli of higher magnitude than their model. This is because, when comparing between two stimulus magnitudes, receivers may make use of different baselines. For example, receivers may use a pre-existing mental template of the model's stimulus magnitude as a baseline. Alternatively and importantly, receivers could consistently treat either the higher, or the lower, stimulus magnitude of the two (whether model or mimic) as the baseline against which the other is compared (Akre & Johnsen 2014). Consider a model possessing a trait with a stimulus magnitude of 3, and imperfect mimics with stimulus magnitudes of either 2 or 4. In a comparison between model and imperfect mimic, receivers may consistently treat the lower stimulus magnitude as the baseline (i.e., comparing either 2 vs. 3, where the mimic has the lower stimulus magnitude, or comparing 3 vs. 4, where the model has the lower stimulus magnitude). If so, then the proportional difference is if the mimic's magnitude is 2 (because ), and if the mimic's magnitude is 4 (because ) (Fig. 3D). The latter proportional difference is smaller and therefore less discriminable than the former, according to Weber's Law. The situation is identical if receivers treat the higher stimulus magnitude as the baseline: the proportional difference is if the mimic's magnitude is 2 (because ), and if the mimic's magnitude is 4 (because ). Again, the latter proportional difference is smaller. A mimic is therefore less discriminable from the model when its stimulus magnitude is higher than that of the model, than when its stimulus magnitude is lower than that of the model (Fig. 3D).
Therefore, if specific conditions are met (i.e., mimicry is imperfect, stimuli are proportionally processed by receivers, and receivers treat either the higher or lower stimulus magnitude as the baseline), Weber's Law predicts that deceptive mimics should track their models toward evolving decreased stimulus magnitudes, while erring toward higher stimulus magnitudes than their model. There should be no such trend in honest mimicry, because both models and mimics benefit equally from reduced discriminability. Consistent with this prediction, L. elapsoides mimics have a greater area of aposematic red coloration than their M. fulvius models (Fig. 2G, H), although it remains to be determined whether all the necessary conditions are met for this observation to be explained by this prediction of Weber's Law.
WHAT IF RECEIVER PERCEPTION DOES NOT ADHERE TO WEBER'S LAW?
Weber's Law states that receiver discrimination should depend on the relative difference between stimuli. If Weber's Law does not apply, such as when processing is limited by the receiver's sensory physiology, or subject to perceptual processes such as categorical or linear processing, then relative stimulus differences should not predict discrimination. For example, the receiver's sensory acuity (the resolution with which a sensory system can parse information) could be the only limit on what is discriminable, meaning any difference that a receiver can resolve is discriminable. Under categorical perception (e.g., Caves et al. 2018), stimuli within categories are less discriminable than equally different stimuli between categories, regardless of the absolute magnitude of the difference. Under linear perception (e.g., Levi et al. 1988), discriminability should increase with absolute, but not relative, stimulus difference. Weber's Law does not apply in such cases and so the benefits and costs of high- and low-magnitude stimuli discussed above should not emerge, because relative differences will not predict discriminability. However, other factors such as the costs of producing high-magnitude signals may also influence the evolution of stimulus magnitudes of models and mimics, and these must be tested alongside the predictions of Weber's Law (Box 2).
SIGNAL VERSUS CUE MIMICRY
So far, we have discussed cases in which a mimic simulates (either honestly or deceptively) a model's signal. In such “signal mimicry,” by definition, the signals of both model and mimic share an intended receiver. However, instead of mimicking model signals, some mimics instead mimic model cues, which are defined as stimuli that have not evolved to elicit responses from a certain receiver. In such “cue mimicry,” cues are defined with respect to the mimic's receiver (Jamie 2017). A model's cue may have no signaling function to the model. For example, predators may mimic an inanimate model, such as a twig, to remain unnoticed by prey; the traits of the model (here, the twig) have not evolved to signal to the mimic's intended receiver (here, the prey). Alternatively, a model's cue may have a signaling function intended for a different receiver than the mimic. For example, Vidua finches attract mates by mimicking the songs of estrildid finches. This is an example of cue mimicry because estrildid songs have evolved as signals to other estrildids, and not to the mimic's intended receiver (here, conspecific Vidua finches) (Payne et al. 2000). In cases of cue mimicry, model and mimic do not share an intended receiver. Therefore, selection from the receiver on the mimic should not influence the evolution of the model (Jamie 2017). Thus, although Weber's Law can influence the evolution of mimics alone (in cases of cue mimicry), for receiver perception to affect the coevolution of models and mimics, mimics must copy a models’ signals rather than their cues (as occurs in signal mimicry).
SUMMARY OF PREDICTIONS FOR EVOLUTION OF MIMICRY SYSTEMS UNDER WEBER'S LAW
If receivers process stimuli proportionally, then the evolutionary trajectories and mimetic fidelity of mimicry systems should depend on the stimulus magnitudes of models and mimics. This hypothesis generates four testable predictions, illustrated in Figure 3, that are only applicable when receivers process stimuli proportionally. Predictions 1 and 2 relate to the evolutionary trajectories and optimality of absolute stimulus magnitudes of models and mimics, respectively, whereas predictions 3 and 4 relate to the magnitude and directionality of the difference between the model and the mimic. Prediction 1 relates to models specifically, and therefore applies only to signal mimicry systems in which models evolve in response to mimics (see Akcali et al. 2018; Benitez-Vieyra et al. 2007; Mallet 1999 for signal mimicry systems in which models do not evolve in response to mimics). Predictions 2–4 relate to mimics, and are applicable to both signal mimicry and cue mimicry.
- Prediction 1:
Models in honest mimicry systems should, all else being equal, evolve stimuli of greater absolute magnitude than models in deceptive mimicry systems (Fig. 3A).
- Prediction 2:
Mimics should exhibit greater fitness or success at higher stimulus magnitudes, regardless of whether mimicry is honest or deceptive (Fig. 3B).
- Prediction 3:
Regardless of whether mimicry is honest or deceptive, mimicry of high-magnitude stimuli should be more imperfect (i.e., larger and more variable stimulus differences between model and mimic should be able to persist) than mimicry of low-magnitude stimuli (Fig. 3C).
- Prediction 4:
Assuming that receivers consistently treat either the stimulus of higher or lower magnitude as a baseline (regardless of whether this is the model's or the mimic's stimulus), and that mimicry is imperfect, stimulus magnitudes should evolve to be higher in deceptive or cue mimics than in their models (Fig. 3D).
These four predictions are testable within and between systems (Box 2), and could contribute to explaining the variation between mimicry systems in mimetic fidelity and stimulus evolution. They also suggest that the evolution of traits toward increased or decreased magnitudes may not be arbitrary, as is often implicitly assumed (e.g., in signal detection theory; Oaten et al. 1975).
Here, we highlight several mimicry systems in which species differ in traits such as color patch area or marking size that might be proportionally processed. These lend themselves to tests of the evolutionary predictions of Weber's Law that we have described, although of course researchers will best understand the strengths and weaknesses of their own study systems. Potentially suitable honest mimicry systems include Müllerian mimicry rings of ladybirds, apheloriine millipedes, Yponomeuta and arctiine moths, Heliconius butterflies, and velvet ants (Brakefield 1985; Marek & Bond 2009; O'Reilly et al. 2019; Turner 1981; Wilson et al. 2015). Examples of rewarding mimicry are rare, although some plant-pollinator systems may be suitable (Benitez-Vieyra et al. 2007; Jamie 2017). Potentially suitable deceptive mimicry systems include Batesian mimicry in scarlet kingsnakes, Papilio butterflies, and hoverflies (Harper & Pfennig 2007; Kunte 2009; Penney et al. 2012), and aggressive mimicry in avian brood parasites (Section 2) and fangblenny fish (Plagiotremus rhinorhynchos; Cheney & Côté 2005). In general, systems with geographical variation in the incidence of models and mimics may be optimal, because comparisons of stimulus magnitudes can be made between areas where the species co-occur (where selection should act on discriminability) and areas where only one species occurs. Moreover, systems where evolutionary history of stimuli can be inferred, such as those in which historical museum collections exist, could be used to determine evolutionary trajectories of proportionally processed stimuli.
Testing all four predictions outlined in Section 1 requires a mixture of studies of specific systems, and comparisons across systems. In both honest and deceptive systems where the mimics and models are prey, an experiment on prey survival could determine whether models with higher magnitude stimuli are discriminated less readily than those with lower magnitude stimuli (Prediction 1). A similar experiment could determine whether imperfect mimics with higher stimulus magnitudes are predated less often than imperfect mimics with lower stimulus magnitudes (Predictions 2 and 4). Although such studies will ultimately benefit from being conducted in situ, controlled lab studies are probably a realistic first step to isolate effects of proportional processing. Controlled environments such as the “novel world” experimental arena (Alatalo & Mappes 1996), which has been used for studies of social learning (e.g., Hämäläinen et al. 2020), could provide a good setting for conducting such studies. Here, predators are exposed to a novel artificial environment containing manipulated “prey” items, to study predator responses to prey stimuli in a controlled manner.
Where historical data exist, the direction of stimulus magnitude evolution over time can be quantified (Predictions 1 and 4). Comparative studies carried out with systems in which mimics vary along a continuum from honest to deceptive (as has been suggested in Heliconius butterflies; Brower et al. 1963) could determine whether stimulus magnitudes differ adaptively between honest and deceptive mimics (Prediction 1). Similarly, studies could test whether lower magnitude stimuli show greater mimetic fidelity than higher magnitude stimuli in comparable mimicry systems (Prediction 3).
Other factors aside from Weber's Law influence the evolution of mimicry systems, which may affect the suitability of different study systems for testing the predictions of Weber's Law. For example, greater mimetic fidelity may evolve in systems where receivers have more time to discriminate mimics from models, than when receivers must make rapid decisions. Hosts of brood parasites have the opportunity to study the eggs in their nests carefully before deciding whether to reject a potential mimic that will hatch weeks later. By contrast, in many predator-prey systems, the receiver must make a rapid decision (Chittka & Osorio 2007), which may result in weaker selection for mimetic fidelity, irrespective of any effect of Weber's Law. Similarly, mimetic fidelity will depend on factors including the costs of receiver errors and genetic constraints (Kikuchi & Pfennig 2013; McLean et al. 2019). These considerations should be taken into account when testing whether mimetic fidelity depends on stimulus magnitudes across systems (Prediction 3).
High- or low-magnitude stimuli may also have costs and benefits independent of Weber's Law. For example, high-magnitude stimuli may be costly to produce or increase susceptibility to detection by predators, resulting in evolution toward lower stimulus magnitudes. This hypothesis makes the same prediction as Prediction 1 for models of deceptive mimics, and so must be tested alongside the hypothesis that Weber's Law affects the evolution of stimulus magnitudes. One way to distinguish between these hypotheses would be to compare the evolution of model stimulus magnitudes in areas where the model and mimic co-occur, with areas where only one species occurs. If the selective agent is a cost of producing high-magnitude signals, both populations should evolve toward low-magnitude signals. If selection is due to receiver discrimination between model and mimic (modulated by Weber's Law), only the population in which both species co-occur should exhibit evolution toward lower stimulus magnitudes.
In summary, other factors that might produce similar predictions should be tested alongside the hypothesis that Weber's Law affects the evolution of stimulus magnitudes. Researchers will be best placed to consider which specific alternative hypotheses to test in their study systems. However, perhaps the most important test for distinguishing between alternative hypotheses is to confirm that receivers proportionally process the stimuli of interest. We detail how to do this in Section 2.
Section 2: A Framework for Testing Weber's Law
Here, we provide a four-step framework for testing Weber's Law and illustrate it with real and hypothetical examples of aggressive mimicry, focusing on egg mimicry in avian brood parasites. Avian brood parasites lay eggs in the nests of “hosts,” which as a result often incur considerable costs through the death or reduction in fitness of their own offspring (Davies 2000). Hosts often evolve the ability to identify and reject parasitic eggs, in turn selecting for the evolution of egg mimicry by parasites. Hosts may evolve complex signatures in egg markings to make it harder for parasites to successfully mimic their eggs (Spottiswoode & Stevens 2010; Swynnerton 1918) and enhanced discrimination abilities to better identify parasitic eggs from their own (Spottiswoode & Stevens 2011). Avian brood parasite-host systems are often tractable for experimental research (Rothstein 1990): receivers are known and their responses easily elicited and scored, visual phenotypes are readily measurable, and in many cases the stimuli used by hosts in discrimination are already known. Therefore, they provide excellent case studies for investigating how to test Weber's Law correctly in coevolutionary mimicry systems.
STEP 1: IDENTIFY RECEIVER AND STIMULUS
It follows directly from the definition of Weber's Law that there must exist a receiver, and an appropriate stimulus. These terms should be specifically defined, particularly in a coevolutionary context.
Receiver species should be studied independently
In some coevolutionary systems, there may be several different receiver species, with different sensory and discrimination capabilities, and who pay attention to different stimuli (Dalziell & Welbergen 2016). For example, Heliconius butterflies are Müllerian mimics that signal toxicity to predators, but also signal their own species identity to conspecifics to avoid costly interspecific matings (Estrada & Jiggins 2008). Predators and conspecifics are thought to process different aspects of Heliconius wing pattern signals (Briscoe et al. 2010; Bybee et al. 2012; Dell'Aglio et al. 2018). In such systems, each receiver species must be studied independently to determine whether it exhibits proportional processing of the stimuli that it detects.
The stimulus must have an associated quantitative magnitude with a linear axis
It is also essential to identify an appropriate stimulus. For proportional processing to be feasible, a stimulus must have an associated quantitative magnitude, which includes zero, is never negative, and increases on a linear axis (Smeets & Brenner 2008). Weber's Law states that a greater stimulus difference is required to discriminate between stimuli of greater magnitude, and so it must be possible to say that stimulus X has a greater magnitude than stimulus Y. For example, stimuli associated with numerosity, such as the number of “chucks” in frog calls (Akre et al. 2011) and number of fish in a shoal (Gómez-Laplaza & Gerlai 2011), meet this requirement. However, when differences are large and easily distinguished irrespective of stimulus magnitude, proportional processing will likely not affect discrimination and therefore Weber's Law may not be biologically relevant.
“Stimulus magnitude” and “stimulus difference” must correspond to the same stimulus
In the equation for Weber's Law, ∆I is the stimulus difference (i.e., the difference in magnitude between two stimuli being compared), I is the stimulus magnitude, and k is a constant (eq. 1). Crucially, ∆I and I must refer to the same stimulus and be measured in the same units, because k is dimensionless.
If the stimulus is not explicitly defined, then it is easy to make an equivocation error (i.e., use a term inconsistently within an argument), as several of us (the authors) have done in the past. For instance, does Weber's Law predict that when clutches are large, rejection of foreign eggs becomes more difficult? It may seem intuitive that larger clutches have a larger stimulus magnitude than smaller clutches, and therefore discrimination of foreign eggs becomes more difficult as clutch size increases. Indeed, American robins Turdus migratorius with smaller clutches rejected a dark blue experimental egg added to their nest more readily than did robins with larger clutches of pale blue eggs (Abolins-Abols & Hauber 2020). The authors of this study cautiously suggest that their findings are consistent with Weber's Law, arguing that the greater stimulus magnitude (a larger surface area of pale blue, due to more robin eggs being present) of a larger clutch should reduce the robins’ ability to recognize a dark blue foreign egg compared to when the experimental egg is added to smaller clutches. However, if robins discriminate between foreign eggs based on color, then there is an equivocation error. This is because the stimulus referred to in the context of stimulus magnitude I (surface area of the clutch) is different to the stimulus referred to in the context of stimulus difference ∆I (color difference). Hence, Weber's Law cannot explain how these birds recognize the foreign eggs added in this experiment. Interestingly, Weber's Law could apply to how American robins in this study detect that parasitism has occurred, but not apply to recognition of which egg is parasitic (see Section 3). In summary, to avoid equivocation errors it is essential to define the term “stimulus” throughout, ensuring that both the stimulus magnitude and the stimulus difference refer to the same stimulus.
STEP 2: TEST WHETHER RECEIVERS USE THE STIMULUS IN DISCRIMINATION
An essential step when testing Weber's Law is to demonstrate that receivers process the stimulus being tested in discrimination (for examples where deviations from Weber's Law are observed because this criterion is not met, see Xu & Spelke 2000; Olsson et al. 2015; Smeets & Brenner 2008). For example, when testing how angelfish (Pterophyllum scalare) choose which size shoal to join, Gómez-Laplaza and Gerlai (2011) simultaneously demonstrate that angelfish do indeed choose based on shoal size, and that their choice occurs in line with Weber's Law. Clearly, if angelfish did not use shoal size in decision-making, then Weber's Law could not apply to the stimulus of shoal size.
STEP 3: TEST FOR WEBER'S LAW
If the stimulus difference relative to the stimulus magnitude (ΔI/I) predicts discrimination better than the absolute stimulus difference (ΔI), then Weber's Law has been demonstrated (eq. 1). One can also test for the near-miss and opposite-miss to Weber's Law by including absolute stimulus magnitude (I) in the model that also contains relative stimulus difference (ΔI/I). If, in such a model, absolute stimulus magnitude positively affects discrimination then the near-miss is supported, whereas if absolute stimulus magnitude negatively affects discrimination then the opposite-miss is supported (for details, see LaBarbera et al. 2020).
STEP 4: CONSIDER (CO-)EVOLUTIONARY IMPLICATIONS
In Section 1, we discussed evolutionary implications of Weber's Law; here, we provide a hypothetical example to suggest how testing for Weber's Law could contribute toward an understanding of potentially counterintuitive results in specific systems.
Consider pattern complexity, which can be defined as a measure of how difficult a pattern is to reproduce (Stoddard et al. 2014). Hosts of brood parasites may produce complex egg pattern signatures that are difficult to mimic. If hosts proportionally process differences in pattern complexity when discriminating between eggs, then a host should be able to discriminate between two eggs with simple patterns more easily than between two eggs with complex patterns. In this way, simpler host patterns may evolve to improve discrimination of foreign eggs (Prediction 1 [Section 1]), as opposed to the long-standing hypothesis that selection should select for more complex patterns as these may be more difficult to mimic (Stoddard et al., 2014). A study on common cuckoo and host egg phenotypes (Stoddard et al. 2014) suggested that intermediate complexity may be favored because of the trade-off between advantages of high complexity (making forgery more difficult) and advantages of lower complexity (making eggs more recognizable, defined as how easily an egg could be matched to its clutch). However, we suggest here that Weber's Law could also contribute to the benefits of simplicity for facilitating discrimination. This example demonstrates that testing Weber's Law has the potential to explain aspects of a system that are counterintuitive, or are not explained by other factors.
Section 3: Case Studies
Because Weber's Law has largely been overlooked in the study of coevolution, we provide two brief hypothetical case studies to illustrate the four-step framework outlined above (Section 2), and to highlight coevolutionary implications of Weber's Law (Section 1). Hosts of brood parasites often recognize visual mimics by discriminating between eggs. However, a first line of defense could be detection of the addition of an egg to a clutch (in the absence of recognition of which egg is foreign). Therefore, in the case of egg rejection, Weber's Law and its coevolutionary implications can be tested with respect to both detection and recognition of parasitic eggs; the two case studies below focus on detection of a change and recognition of a mimic, respectively.
CASE STUDY 1: DETECTION
Step 1: Identify receiver and stimulus
If parasites add their own egg to a host clutch without concurrently removing a host egg (e.g., greater honeyguides Indicator indicator [Spottiswoode and Koorevaar 2012]; lesser honeyguides Indicator minor [Skead 1951]; American coots Fulica americana [Lyon 2003]), then hosts (i.e., the receivers) might detect a parasitism event on the basis of a change in clutch size (i.e., the stimulus) (Section 2, STEP 1).
Step 2: Test whether receiver uses the stimulus in discrimination
If hosts upregulate anti-parasite defenses (e.g., abandoning their nest) when an egg is added to a clutch, this implies that the addition of an egg is involved in discrimination. This can be tested with experiments in which an egg is either added to a host clutch (i.e., increase in clutch size) or swapped for a host egg (i.e., no change in clutch size, despite experimental addition) and rates of anti-parasite defenses are recorded.
Step 3: Test for Weber's Law
Weber's Law can be tested using the same experimental setup as in Step 2, by determining whether anti-parasite defenses are elicited more readily when clutch size is small. Specifically, if the relative difference in clutch size caused by adding a parasitic egg to the host clutch predicts defense behavior, in the absence of other indicators of parasitism, then this is evidence for Weber's Law (Section 2, STEP 3). For example, the finding that American robins with smaller clutches are more likely to reject foreign eggs than those with larger clutches (Abolins-Abols & Hauber 2020; Section 2, STEP 1) is consistent with Weber's Law when applied to detection of parasitism.
Step 4: Consider (co-)evolutionary implications
Although this example does not involve discriminating mimics from models, coevolutionary predictions from Section 1 can be tested. For example, hosts should evolve smaller clutch sizes, because this facilitates detection of parasitism (Prediction 1 [Section 1]; Akre & Johnsen 2014). Consistent with Prediction 1, comparative evidence suggests that parasitized species lay smaller clutches than unparasitized species, and that the frequency of nest abandonment (an anti-parasite defense) is negatively correlated with clutch size (Hauber 2003), although this study did not interpret these results in terms of Weber's Law but rather other factors. To determine that lower clutch sizes evolve due to Weber's Law rather than other selective forces, it would be necessary to show that these reductions in clutch size occur in host species that detect additional eggs based on Weber's Law, but not in hosts that do not.
Parasites, however, should preferentially target larger clutches, because they benefit from the addition of their egg going undetected (Prediction 2 [Section 1]; Akre & Johnsen 2014). Prediction 2 can be experimentally tested by manipulating host clutch sizes, in systems where brood parasites examine host clutches before laying and can therefore target specific nests, rather than laying indiscriminately.
CASE STUDY 2: RECOGNITION
As described above, American robins could detect parasitism by the addition of an egg. However, to single out a parasitic egg and reject it, they must use other stimuli to recognize which is the foreign egg, and avoid rejecting their own eggs (Davies & Brooke 1988). In the context of recognition, Weber's Law is testable if recognition is based on stimuli for which quantitative magnitudes can be measured, as in the following hypothetical example.
Step 1: Identify receiver and stimulus
In principle, hosts (the receivers) might use a trait such as the number of markings on eggs (the stimulus) to recognize parasitic eggs.
Step 2: Test whether receivers use the stimulus in discrimination
We must first test whether hosts do indeed discriminate between eggs based on the number of markings on them. This can be done using egg rejection experiments, in which experimental eggs are placed inside host nests and rejection behavior quantified (e.g., Davies & Brooke 1989; Spottiswoode & Stevens 2010). If the difference in the number of markings between host and experimental eggs predicts rejection (as seen in fork-tailed drongos Dicrurus adsimilis; Lund & Spottiswoode, unpublished data), this implies that the number of markings is used in discrimination.
Step 3: Test for Weber's Law
In addition to demonstrating that hosts discriminate between eggs based on the number of markings, such egg rejection experiments can be used to directly test for Weber's Law itself. Specifically, if the relative difference in the number of markings between host and experimental eggs predicts egg rejection better than the absolute difference, then this is evidence for Weber's Law (c.f. Fig. 1).
Step 4: Consider (co-)evolutionary implications
Given that brood parasites exhibit deceptive mimicry, the number of markings on host eggs should decline over time (because eggs with lower stimulus magnitudes are more discriminable from those of their parasites; Prediction 1 [Section 1]). If historical collections allow examination of egg phenotypes from the past, then this prediction could be tested. Field studies or examination of egg collections of brood parasites and hosts in museums might also permit comparison of levels of mimetic fidelity across hosts: if many host species discriminate based on the number of markings, then Weber's Law predicts greater mimetic fidelity in parasites targeting hosts that exhibit lower number of markings, than those that exhibit higher number of markings (Prediction 3).
Conclusions
In this article, we highlight the advantages of considering Weber's Law in the study of coevolution, and particularly mimicry, and provide guidance on pitfalls to avoid when doing so. Many factors can affect the evolution of models and mimics, and thus other hypotheses unrelated to Weber's Law must be tested. If researchers do choose to study Weber's Law in coevolutionary systems, then we urge that it is essential to identify the receiver in question; to identify the stimuli being used in discrimination; and to ensure that when defining “stimulus magnitude” and “stimulus difference,” these measures apply to the same stimulus. In general, defining terms explicitly can help to prevent misapplications of Weber's Law.
Applying Weber's Law appropriately to mimicry systems will help both to generate and to test predictions about optimal phenotypes in models and their mimics, the potentially dissimilar evolutionary trajectories that mimics and models may follow, and the variation in mimetic fidelity between mimicry systems. In particular, the specific type of mimicry being exhibited may shape how proportionally processed stimuli evolve. Such effects of receiver perceptual processing on the evolution of mimicry systems emphasize that receiver perception should be integrated into theoretical models of coevolution between models and mimics, as it can drive evolution in otherwise unexpected or counterintuitive directions.
ACKNOWLEDGMENTS
We thank M. E. Hauber and M. Abolins-Abols for discussing their study with us. We also thank J. M. Riederer, J. Lund, M. N. Attwood, G. A. Jamie, M. K. Dixit, and J. M. Walker for comments on an earlier version of the manuscript. TD was funded by a Balfour studentship from the Department of Zoology, University of Cambridge. EMC was funded by the European Union's Horizon 2020 research and innovation program under the Marie Sklodowska-Curie grant agreement (No. 793454). CNS was funded by a BBSRC David Phillips Fellowship (BB/J014109/1) and the European Research Council (Consolidator Grant 725185).
AUTHOR CONTRIBUTIONS
TD led the conception and writing, with contributions from all coauthors.
CONFLICT OF INTEREST
The authors declare no conflict of interest.
LITERATURE CITED
Associate Editor: J. L. Sachs
Handling Editor: T. Chapman