-
PDF
- Split View
-
Views
-
Cite
Cite
Michinori Hirano, Hiroaki Yamamoto, Yuhi Hasebe, Koji Fukuda, Susumu Morosawa, Hirokazu Amamizu, Kazuma Ohyama, Hironori Uzuka, Kazuyoshi Takayama, Hiroaki Shimokawa, Development of a novel shock wave catheter ablation system—A validation study in pigs in vivo, EP Europace, Volume 20, Issue 11, November 2018, Pages 1856–1865, https://doi.org/10.1093/europace/eux244
- Share Icon Share
Abstract
Although the radiofrequency catheter ablation (RFCA) is widely used for the treatment of tachyarrhythmias, it has three fundamental weaknesses as a thermal ablation system, including a limited lesion depth, myoendocardial injury linking to thromboembolism, and prolonged inflammation followed by subsequent recurrences. In order to overcome these limitations, we have been developing a shock wave (SW) catheter ablation (SWCA) system as a novel non-thermal therapy. In the present study, we validated our new SWCA system with increased SW intensity.
In a total of 36 pigs, we applied our new SWCA to ventricular muscle in vivo for the following protocols. (i) Epicardial approach (n = 17): The lesion depth achieved by the SWCA from the epicardium was examined. High intensity SW achieved 5.2 ± 0.9 mm lesions (35 applications), where there was a strong correlation between SW intensity and lesion depth (R = 0.80, P < 0.001, 54 applications). (ii) Endocardial approach (n = 6): The extent of endocardial injury with the two energy sources was examined by electron microscopy (8 applications each). Shock wave catheter ablation markedly reduced myoendothelial injury compared with RFCA (4.3 ± 1.2 vs. 79.6 ± 4.8%, P < 0.01). The electrophysiological effects on the SW lesions were also confirmed using three-dimensional mapping system. (iii) Time-course study (n = 6 each): The healing process after ablation therapy was examined. We found transient inflammatory responses and accelerated reparative process with preserved blood flow in the SWCA group.
These results indicate that our SWCA system is characterized, as compared with RFCA, by deeper lesion depth, markedly less myoendocardial injury and accelerated tissue repair process.
What’s new?
We demonstrated that our shock wave catheter ablation (SWCA) could be a promising system for control of tachyarrhythmias with the following superiorities to conventional radiofrequency ablation;
The SWCA system could cause deeper lesion with markedly less myoendocardial injury.
The SWCA system also has functional electrophysiological feasibility.
Shock wave catheter ablation lesions showed more rapid healing process with preserved myocardial blood flow, presumably resulting in a good long-term control for tachyarrhythmias.
Introduction
Radiofrequency catheter ablation (RFCA) is an established therapy for drug resistant tachyarrhythmias, including atrial fibrillation (AF), ventricular tachycardia (VT), and ventricular fibrillation (VF). However, conventional RFCA, although quite effective and feasible, has the three fundamental weaknesses as a thermal ablation system, including a limited lesion depth, myoendocardial injury with thromboembolic complications, and sustained post-procedural inflammation with subsequent early phase recurrences.1–5 Indeed, arrhythemogenic substrates are frequently located in deep intramural lesions in structural heart diseases, for which conventional RFCA has limited ability to achieve deep intramural lesions.1–3 In addition, RFCA causes myoendothelial damage, occasionally resulting in thromboembolic complications and steam-pop.4,5 The frequency of symptomatic stroke caused by RFCA has been reported to be 0.6% in general and 1.8–2.0% in left-side heart procedures.5 Finally, RFCA causes prolonged inflammatory response with subsequent early phase recurrences.6
In order to overcome these weaknesses of the conventional RFCA therapy, novel ablation systems have been under development, such as photodynamic therapy and electroporation.7,8 However, no system has yet overcome these weaknesses of conventional RFCA in a satisfactory manner. Both systems have the limitation of lesion depth in addition to safety use; the photodynamic therapy was evaluated only for atrial application in the clinical settings,7 and the electroporation had limitations of inconsistent lesion depth and arrhythmogenicity.8 Shock wave (SW) is an acoustic pressure wave consisting of a compressive phase (over pressure) followed by a tensile phase (negative pressure) (Figure 1A). Focused SW, which is used for extracorporeal shock wave lithotripsy, can cause tissue damage at an arbitrary depth without heat generation. We have been developing a shock wave catheter ablation (SWCA) system as a novel non-thermal therapy (Figure 1B). The concept of our SWCA system is that it could cause deeper lesion without severe superficial injury by using focused SW. We have recently demonstrated that a prototype SWCA causes 2 mm depth lesions with less superficial injury and less thrombus formation compared with RFCA in pigs in vivo.9 However, the lesion depth was insufficient for ventricular arrhythmias, and the influences of SWCA on myocardium and blood vessels remain to be examined. We have recently improved the SW generator so that it produces 1.5 times greater intensity of SW than the previous prototype to achieve deeper lesion. In the present study, we thus examined the feasibility and safety of our new SWCA system in pigs in vivo.
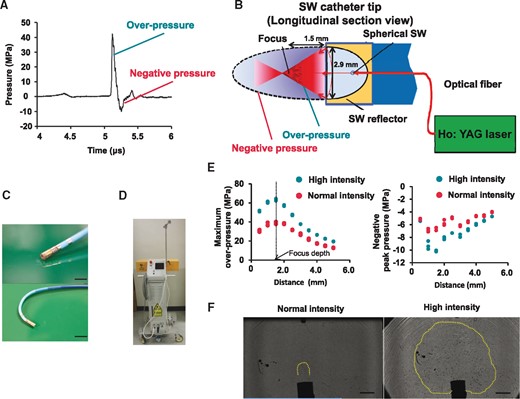
Characteristics of the new shock wave catheter ablation system. (A) Shock wave pulse form in the focal zone; SW is an acoustic pressure wave consisting of a compressive phase (overpressure) followed by a tensile phase (negative pressure). (B) Schematic diagram of the SWCA system: the spherical SW is generated in a water-filled semi-elliptical reflector attached to the catheter tip by irradiation of Q-switched Holmium (Ho): yttrium aluminium garnet (YAG) laser beam through quartz optical fibre. The SW is then reflected by the reflector and is converged onto the outer focus. Reflector diameter: 2.9 mm, reflector to the focus point (measured): 1.5 mm. (C) The new SW catheter (10Fr deflectable SW catheter) is equipped with bipolar electrodes at the tip for recording local electrograms and 2-ring electrodes for three-dimensional mapping system. Scale bar: 5 mm (upper panel), 10 mm (under panel). (D) The newly improved laser generator. (E) Distributions of both maximum overpressure (left panel) and negative peak pressure (right panel) were greater with high intensity compared with normal intensity. Measured focal length was 1.7 mm. (F) Shadowgraphs of two different SW intensities under water taken by high-speed camera, showing cavitation bubbles with normal intensity SW (left panel) and high-intensity SW (right panel). High-intensity SW caused extensive cavitation phenomenon in bubbled ECF buffer. Scale bar, 3 mm.
Methods
Newly improved shock wave catheter ablation system
The new SWCA system includes a 10-French deflectable ablation catheter equipped with improved SW reflector and two polar electrodes at the tip to confirm that the catheter is held vertical to the tissue surface with local electrograms (Figure 1C) and an improved laser generator to which the catheter is connected (Figure 1D).
Characteristics of high-intensity shock wave
The shadowgraph of SW was taken by a high-speed camera (HPV-X2, Shimadzu Corp., Kyoto, Japan) in room air bubbled with extra cellular fluid (ECF) buffer. The pressure distribution of SW was measured using a polyvinylidenefluoride needle hydrophone with a 0.5 mm sensitive diameter and a 35 ns rise time (Müller Instruments Inc., Oberursel, Germany). The signals were stored in the digital transient memory (DS-5534, Iwatsu Electric Co. Ltd., Tokyo, Japan) at a sampling rate of 2GS/s.
Experimental animal models
We performed four protocols with male domestic pigs in vivo (BW 49.1 ± 6.7 kg). All procedures were performed according to the protocols approved by the Institutional Committee for Use of Laboratory Animals of Tohoku University (Nos. 2016MdA-53, 54, and 64).
The animals were anesthetized with medetomidine (0.05 mg/kg, IM) and midazolam (0.4 mg/kg, IM). After 10 min, they were intubated after induction of anaesthesia with inhaled 5% sevoflurane, and were mechanically ventilated with room air and supplemental oxygen. General anaesthesia was maintained with 2.5–4.0% sevoflurane. They were continuously monitored with surface electrocardiograms, percutaneous oxygen saturation measurements, and direct measurements of arterial pressure.
Focused SW was applied at a 1 Hz pulse repetition frequency at each point for 180 shots. For comparison with our new SWCA system, we also performed the RFCA protocols. We used an open-irrigated 3.5 mm tip catheter (Thermocool, Biosense-Webster, Inc., Diamond Bar, CA, USA); the RF energy was delivered in a temperature-controlled manner at 50 °C and a maximum power output of 30 W for 30 s with an irrigation flow of 20 mL/min and limiting impedance decrease of 10 Ω.3,5
Prior to SW or RF application, the animals were pretreated with amiodarone (400 mg/day, PO) for 4 days, in order to prevent RFCA-induced ventricular arrhythmias (VAs). Additional bolus lidocaine (25 mg, IV) was injected when VAs were noted.10 We performed the following four protocols. (i) Epicardial approach (17 pigs, BW 50.2 ± 7.2 kg): We examined whether our new SWCA system could cause deeper lesion with epicardial approach. The animals underwent a standard medial sternotomy, and the pericardium was incised in each animal. We applied SW to the right or left ventricular myocardium with the epicardial approach under direct vision. To minimize the mechanical effects of contact with the catheter tip, the SW catheter was gently attached to and remained vertical to the ventricular myocardium where coronary vessels or thick epicardial fat tissue was absent. (ii) Endocardial approach (6 pigs, BW 50.8 ± 6.6 kg): We examined whether the SWCA system could reduce myoendothelial injury compared with RFCA. The SWCA and RFCA catheters were inserted through percutaneous femoral vein and were placed at the right ventricular target site. Then, SWCA and RFCA were performed as described above (eight sections each). Systemic heparinization by bolus administration (5000 U, IV) followed by continuous infusion (2000 U/h) and additional boluses were performed to maintain activated clotting time of > 300 s during the procedures. After euthanasia, we analysed the treated sites by scanning electron microscopy (SEM) and transmission electron microscopy (TEM). (iii) Ablation study (1 pig, BW 48 kg): We examined whether our SWCA system has functional electrophysiological feasibility with endocardial approach. We applied SW to the right ventricular myocardium so that it caused cluster-like area by dragging method. Electrocardiograms and pacing thresholds at target sites were recorded on endocardial voltage map before and after the SWCA procedure. Bipolar voltage map of the right ventricular endocardium was acquired using ablation catheter (Thermocool, Biosense-Webster, Inc., Diamond Bar, CA, USA) and Ensite NavX mapping system (St. Jude Medical, St. Paul, MN, USA). Conventional voltage thresholds were applied (<0.5 mV as dense scar, 0.5–1.5 mV as border zone, and ≥1.5 mV as normal tissue).1,2 (iv) Time-course study (9 pigs, BW 45.9 ± 5.7 kg): We compared the time-course of lesion healing between RFCA and SWCA in pigs in vivo (nine sections each). The surviving animals were subsequently euthanized at Days 1, 4, and 7 after the procedure (3 pigs each). We compared lesion condition, inflammation cell infiltration, and microvascular proliferation between the two groups. In the endocardial approach study and time-course study, we applied SWCA and RFCA in the same pigs.
In each protocol, the animals were euthanized 1 h after the procedure. After the in vivo experiment, the heart was extracted and subsequently fixed in 10% formalin after the gross examination. The fixed heart was then sectioned, and a vertical-section slide was made for each application site. In electron microscopy study, the samples were fixed with 2% paraformalin and 2.5% glutaraldehyde in 0.2 mol/L cacodylate buffer.
Histological analysis
The samples were fixed in 10% buffered formalin. After embedding in paraffin, 2 μm cuts were performed and stained with histochemical dyes. The tissue specimens were stained with haematoxylin–eosin for all studies, Masson’s trichrome stain for the time-course study (for clear visualization of fibrotic lesions). Immunoreactivities were examined, by using rabbit antihuman von Willebrand factor antibody (1:3) (N1505; Dako, Copenhagen, Denmark) for proliferation of microvessels. All histopathological slides were examined by light microscopy (Olympus BX51, Olympus America Inc., NY, USA). In the lesion depth analysis, we sectioned the centre of lesions to the vertical direction. The lesion depths in consecutive sections were manually measured macroscopically and were validated microscopically, by using the Image-J (U.S. National Institute of Health, Bethesda, MD, USA).
Electron microscopic study
We used SEM (S3200N, Hitachi-High Technologies Inc., Tokyo, Japan), and TEM (H-7600, Hitachi-High Technologies Inc.) for comparison of the two energy sources (RF vs. SW). The samples were fixed in 2.5% glutaraldehyde and post-fixed in 1% osmium tetroxide for 1 hour at 4 °C. In the SEM study, the samples were dehydrated in ascending series of ethanol amylacetate mixture, and critical point dried. The surface of the specimens was coated with osmium prior to observation. Samples used in TEM observation were dehydrated through a series of alcohols, infiltrated with propylene oxide, and embedded in epoxy resin in an inverted beam capsule. Ultra-thin sections were stained with uranyl acetate and lead citrate. An endocardial injury grading score was determined according to the area of endomyocardial injury as follows; intact (no changes, score 0), mild (denaturation of the endothelium, score 1), and severe (loss of the endothelium, score 2). Histomorphometric parameters in the areas with injured endocardium were manually delineated and measured by using the Image-J. We examined three fields per each section randomly. Then, we used the average value of the three fields.
Measurement of regional myocardial blood flow
Coloured microspheres (Dye-Trak, Triton Technology Inc., San Diego, CA, USA) were used for measurement of regional myocardial blood flow.11 After the SWCA or RFCA procedure, yellow microspheres (12 μm in diameter) were injected into the left atrium (6 million microspheres per injection). The microspheres were isolated from tissue by digestion with potassium hydroxide, they were centrifugated, the dyes were extracted from the coloured microspheres, and the separation of colours and measurement of their concentration was performed by spectrometry (Ultrospec 3300 pro, Amersham Bioscience Corp., Amarsham, UK). The basic principle of all deposition techniques for regional flow measurement is that the deposition is proportional to the flow (per unit volume or mass of tissue).11
Statistical analysis
All continuous results are expressed as mean ± standard deviation. The Pearson correlation was computed to assess the associations between lesion depth and SW intensity. The Mann–Whitney’s U test was used to compare the extent of myoendocardial injury between the SW and RF groups. Student’s t-test was used to compare the histological change in the time-course protocol. A P-value < 0.05 was considered to be statistically significant.
Results
Characteristics of the new shock wave catheter ablation system
The new SWCA system was able to generate high intensity SW compared with the previous one.12 The focal length with maximum overpressure was 1.7 mm (Figure 1E). Measured overpressure and negative pressure were greater with high-intensity SW compared with normal intensity SW (Figure 1E). The shadowgraph of SW taken to describe cavitation bubbles generation showed that high-intensity SW caused more extensive cavitation phenomenon compared with normal intensity SW (Figure 1F).
Lesions created by high-intensity shock wave catheter ablation
We first examined whether the new SWCA system could cause deep lesion with epicardial approach. A total of 35 lesions were created in 7 pigs with high-intensity SW (35 applications, maximum overpressure, 55.3 ± 4.7 MPa, Day 0). The lesion depth was 5.2 ± 0.9 mm with high-intensity SW (35 sections), which was deeper than that in designed focus depth (Figure 2A). Histologocal examination (35 sections) showed that coarse injured myocardial tissue was noted at the designed focus region (e.g. disruptions of myocardial fibres, interstitial haemorrhage), whereas at the deeper region, degenerative myocardial cell death was noted (e.g. eosinophilic necrosis, waving, contraction band necrosis) (Figure 2A). Scanning electron microscopy examination (eight applications, eight fields each; focus regions vs. deeper regions) showed that a number of micro-craters developed in myocardial cells not only in the designated focus region but also in the deeper region (Figure 2B).
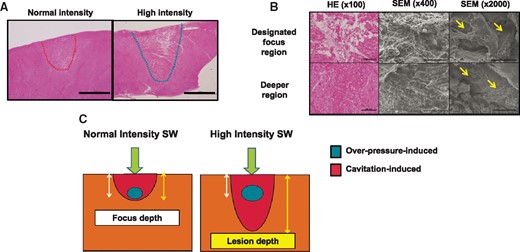
Histopathological findings of ablated lesions by epicardial SWCA. (A) Histopathological findings of epicardial lesions (low magnification); high-intensity SW (right panel) caused deeper lesion than normal intensity (left panel). Scale bar: 2 mm. (B) The extent of injury of myocardial tissue was severe at the designed focus region (upper panel), while only myocardial tissue degeneration was noted at the deeper region (lower panel). A number of micro-craters, which were rarely observed in control regions, were noted on myocardial cells throughout the lesions (yellow arrows). Scale bar; 200, 100, and 20 μm in the left, centre, and right panel, respectively. HE, haematoxylin–eosin; SEM, scanning electron microscope. (C) Mechanism of SWCA-induced deeper lesion formation; SWCA may cause tissue injury through the combination of two different mechanical stresses, shear force by overpressure and cavitation effects. Normal intensity SW causes near focal depth lesion (left panel), whereas high-intensity SW can cause deeper lesion formation through enhanced cavitation effects (right panel).
Correlation between shock wave intensity and lesion depth
The correlation between SW intensity and lesion depth was also examined in 10 pigs with epicardial approach (54 applications, maximum overpressure range, 43.9–90.8 MPa, Day 0). The lesion depth became significantly deeper in response to increasing SW intensity with a strong correlation between them (R = 0.80, P < 0.001) (Figure 3A). Especially when a super-high intensity SW was applied (maximum overpressure > 75 MPa), the lesion depth achieved was 7.8 ± 0.9 mm (8/54 applications), creating transmural lesion in the left ventricular wall (Figure 3B).
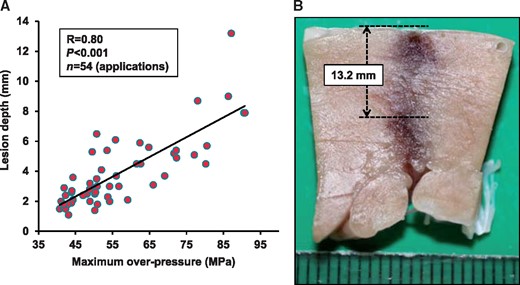
Relationship between SW intensity and lesion depth. (A) Shock wave intensity expressed as maximum overpressure significantly correlated with lesion depth (10 pigs, 54 applications, R = 0.80, P < 0.001). (B) Representative transmural lesion of the left ventricle with super-high intensity SW (75–90 MPa).
Influence of shock wave on the endomyocardium
We compared the extent and characteristics of endomyocardial injury between RFCA and SWCA (six pigs, SEM: eight applications each and two sham, TEM: three applications, Day 0) by using electromicroscopy. The RF lesions showed severe injury (e.g. extensive endocardial disruption, subendocardial necrosis, and severe denaturation), whereas the SW lesions showed only mild denaturation (e.g. small endocardial craters, subendocardial oedema) and little disruption of endocardium (Figure 4A). The SW intensity, as expressed in maximum overpressure, was 58.0 ± 4.0 MPa (eight applications). Importantly, the extents of endomyocardial injured area (Figure 4B) and the injured grading score (Figure 4C) were markedly less with SWCA compared with RFCA (eight sections each, both P < 0.001).
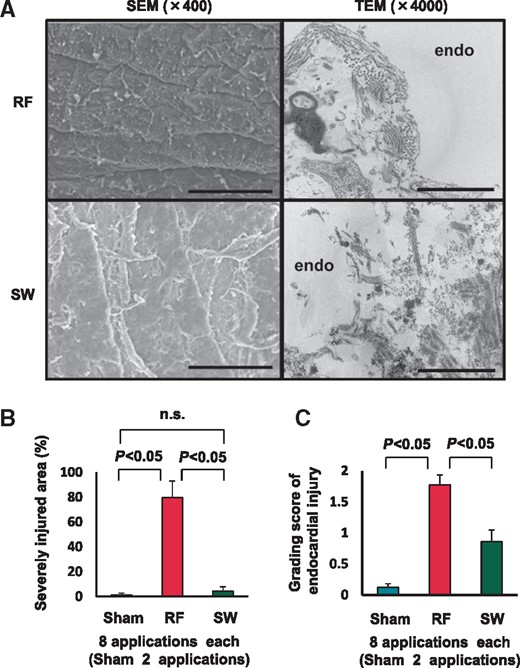
Endomyocardial injury by catheter ablation. (A) Radiofrequency lesion showed severe endomyocardial injury and destroyed endocardial basement membrane structure (upper panels), whereas SW lesion showed only mild denaturation with preserved membrane structure (lower panels). Scale bar; 100 μm (left panel, SEM) and 2 μm (right panel, TEM). SEM, scanning electron microscope; TEM, transmission electron microscope. (B and C) Shock wave catheter ablation caused markedly reduced myoendothelial injury compared with RFCA, in terms of severely injured area (left panel) and grading score of endocardial injury (right panel).
Ablation study
We examined whether electrophysiological effects were achieved by SW lesions with endocardial approach for clinical application. Three-dimensional electro-anatomical map was obtained from 929 accepted endocardial electrograms. Shock wave of 1440 shots was applied to the RV anterior wall so that it caused cluster-like area by dragging method (Figure 5A and B). Pacing threshold measurement was feasible both before and after ablation on five points in the SW application area. Bipolar voltage could be reliably measured before and after applications on the five points in the target area. A decrease in bipolar voltage was consistently noted after ablation (4.11 ± 0.41 mV before ablation vs. 0.24 ± 0.22 mV after ablation, P < 0.001) (Figure 5C and D). Thresholds in the area significantly increased from 0.82 ± 0.10 V to 4.04 ± 1.14 V by ablation application (P < 0.05). No tamponade, VF, or other fatal adverse effects were noted. There was no macroscopic injury to any adjacent organs including the lungs.
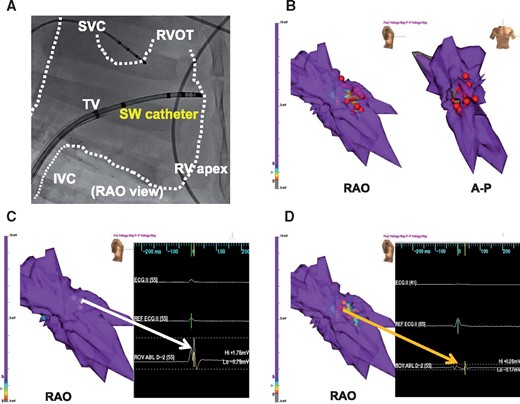
Functional electrophysiological effectiveness of SWCA. (A) Fluoroscopic image of SW catheter position at SW applications inside the right ventricle. IVC, inferior vena cava; RAO, right anterior oblique; RVA, right ventricular apex; RVOT, right ventricular outflow; SVC, superior vena cava; TA, tricuspid annulus. (B) Shock wave of 1440 shots was applied in the RV anterior wall so that it caused cluster-like area by dragging method. Red tags indicate application points. Grey area shows <0.5 mV as injured area, red–blue area 0.5–1.5 mV as border zone, and purple area ≥ 1.5 mV as normal tissue. (C) Representative endocardial voltage map at baseline showed normal tissue (≥1.5 mV as purple) and a normal amplitude electrogram (1.76 mV) in a target region (white arrow). (D) Representative post-SWCA voltage map showed low-voltage area (<1.5 mV as area from red to dark blue) and a low-amplitude electrogram (0.26 mV) in a target region (orange arrow).
Time-course of lesion development and healing
We compared the time-course of lesion development and healing between RFCA and SWCA (nine pigs, Days 1, 4, and 7; three applications each energy source and each day). The time-course of fibrotic change was different between the RFCA and SWCA groups. The changes in the RFCA lesions tended to be slower compared with the SW lesions. In the RFCA group, at Day 7, heterogeneous fibrotic lesion was noted at the border zone, but necrotic lesion still remained at the central zone (Figure 6A). In contrast, in the SWCA group, homogenous fibrotic lesion was noted in the entire lesions at Day 7 (Figure 6A). Maximum overpressure of the SW intensity was 54.2 ± 6.5 MPa (nine applications). The time-course of inflammatory cell infiltration was also examined. In the RFCA group, inflammatory cells tended to slowly increase in the border zone, whereas in the SWCA group, they tended to infiltrate in the zone from the early phase (Figure 6B). In the central zone, this was also the case in the SWCA group, whereas in the RFCA group, such infiltration of inflammatory cells was less evident (Figure 6C).
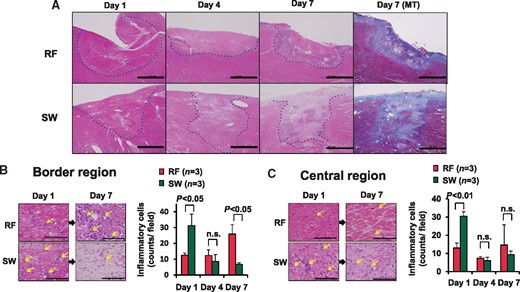
Time-course of formation and healing of ablated lesions. (A) In the RFCA lesion, necrotic lesions and heterogenous fibrotic lesions were noted at Day 7, respectively, whereas in the SWCA lesion, healing process was accelerated with homogenous fibrotic lesions at Day 7. Dashed line denotes the ablated lesion. Scale bars, 2 mm. (B) In the border region, RF lesions had only a few inflammatory cells at Day 1, which was later increased at Day 7, whereas the SW lesions had enhanced infiltration of inflammatory cells at Day 1 followed by rapid decrease at Day 7. (C) In the central regions, the SW lesions had a higher number of inflammatory cells at Day 1 compared with RFCA lesions (left panel, HE). Scale bars, 200 μm. HE, haematoxylin–eosin staining.
Effects of shock wave catheter ablation on myocardial angiogenesis and blood flow
In the RFCA group, more than 50% of small vessels showed thrombotic occlusions, whereas in the SWCA group, such occlusion was markedly suppressed (three applications each, Day 1 P = 0.03) (Figure 7A). Similarly, regional myocardial blood flow in the central zone tended to be decreased in the RFCA group, whereas in the SWCA group, it was significantly increased compared with normal zone and the RFCA group (three pigs, three applications each energy, Day 0, both P < 0.01) (Figure 7B). Time-course of the number of microvessels was also examined. In the border zone, SWCA significantly increased the number of microvessels at Day 7 compared with RFCA (Figure 7C). In the centre zone, SWCA significantly increased the number of microvessels at Days 4 and 7 compared with RFCA (Figure 7D).
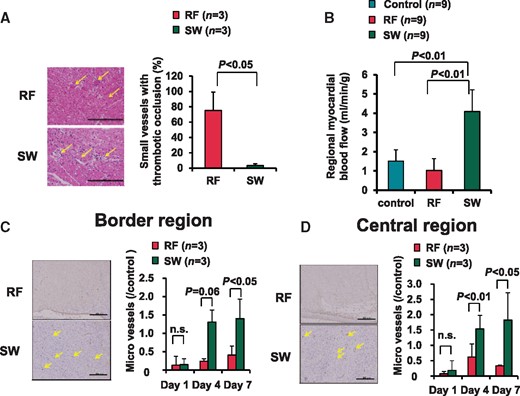
Influence of SWCA on small coronary vessels. (A) In the RF lesions, more than 50% of the vessels showed thrombotic occlusion, whereas almost all small vessels were patent in the SW lesions (left lower panel, arrow). Scale bars: 200 μm. Quantitative data are shown in the right panel. (B) Regional myocardial blood flow was higher in the SW lesions compared with the RFCA lesions. (C and D) The extent of myocardial angiogensis in the border region (C) and the central region (D), as evaluated by the number of microvessels, was significantly increased in the SWCA lesions than in the RFCA lesions at the subacute phase (Days 4 and 7). Scale bar: 200 μm.
Discussion
The major findings of the present study were as follows; (i) our new SWCA system was able to generate high overpressure and extensive cavitation phenomenon, (ii) the SWCA system could cause deeper lesion in response to increasing SW intensity compared with RFCA, (iii) the SWCA system had functional electrophysiological effectiveness, (iv) the SWCA system caused markedly reduced myoendocardial injury compared with RFCA, and (v) the SWCA lesions showed transient inflammatory responses followed by accelerated healing process with preserved myocardial blood flow. These results demonstrate the advantages of the new SWCA system to overcome the limitations of the current RFCA system.
Mechanism of deeper lesion development by shock wave catheter ablation
Shock wave is supposed to cause tissue injury through the combination of two different mechanical stresses; shear force and the cavitation effect.9 When a cavitation bubble collapses near cell membrane surface, the collapse is asymmetrical with a resultant formation of a liquid jet towards the surface. A number of micro-jets puncture cell membrane, thereby creating a number of micro-craters, so-called as cavitation phenomenon.13 The increased cellular permeability results in the disruption of cellular haemostasis and in cell death.13
We consider that the development of deeper lesions by high-intensity SW is caused by a greater cavitation phenomenon (Figure 2C). This notion is supported by the present findings that high-intensity SW caused extensive cavitation phenomenon in vitro (Figure 1B) and that degeneration with a number of micro-craters was noted in the deeper region presumably caused by micro-jets in vivo (Figure 2B).
Clinical superiority of shock wave catheter ablation
The previous studies showed that 3–6 mm depth lesions achieved with conventional RFCA may not be sufficient to treat any type of VAs with endocardial approach.3 Furthermore, certain arrhythmogenic substrates may exist in a deep intramural tissue beyond the reach of the combination of endocardial and epicardial ablation.2 Epicardial application of SWCA could cause less superficial injury as in endocardocardial approach, and may be able to overcome the present obstacle of RFCA epicardial approach such as epicardial fat tissue. Furthermore, our SWCA has a potential to achieve transmural lesion from the endocardium. In the present study, the new SWCA system achieved 5.2 ± 0.9 mm depth lesions with high intensity SW (50–60 MPa), and 7.8 ± 0.9 mm depth lesions with super-high intensity SW (75–90 MPa). Furthermore, our SWCA system was able to create transmural lesion in the left ventricular muscle (13.2 mm) with super-high intensity SW (Figure 3B). Since there was a strong correlation between SW intensity and lesion depth, our SWCA system would be useful from atrial muscle to ventricular muscle by controlling SW intensity.
The conventional RFCA has several clinical complications as it utilizes the joule heat as an energy source, including myoendocardial injury, thromboembolic complications, collateral damage to extra-cardiac structures, and steam-pop.4,5,12 Especially, thromboembolism is a serious complication of the current RFCA, which is directly caused by the joule heat and indirectly caused by endocardial injury with resultant activation of coagulation.14 In contrast, the SWCA system is unlikely to cause thromboembolism as it utilizes non-thermal energy souse with less damage to the endocardium. In addition, the non-thermal SWCA system is able to avoid steam pops principally, which point was confirmed in the present study.
Unstable lesions such as a border zone of myocardial infarction could become arrhythmogenic substrates.15 The control of tachyarrhythmias sometimes becomes difficult after catheter ablation, presumably because of incomplete or unstable ablation lesions.16 Accelerated wound healing and early stabilization of ablated lesions may be important for control of tachyarrhythmias. In the present study, the healing process of ablated lesions was obviously different between SWCA and RFCA. The SWCA system prompted the inflammation process and homogenous fibrotic lesions as compared with RFCA (Figure 6). The mechanisms of rapid inflammation and rapid healing process may be, at least in part, due to preservation of blood flow in ablated area.17 Indeed, in the present study, the SWCA preserved intramyocardial microvessels while RFCA occluded them with thrombi. Furthermore, the extent of myocardial angiogenesis was enhanced in the SWCA group in the subacute phase compared with the RFCA group (Figure 7). Accelerated healing processes are similarly observed with cryoablation, which is also associated with greater freedom from arrhythmias after ablation as compared with RFCA.18,19,20 These findings suggest that the SWCA is useful for long-term control of arrhythmias.
Cryoablation is known as one of the most successful novel ablation devices.18 As compared with RFCA, cryoablation has some advantages, including reduced risk of thromboembolism due to decreased injury of endomyocardium, rapid healing process due to lack of vascular or endothelial disruption, and avoidance of steam pop,18 as our SWCA system. However, lesion depth of cryoablation (5.8 ± 0.6 mm) is insufficient for ventricular ablation.21 Our new SWCA system achieved 7.8 ± 0.9 mm depth lesions with super-high intensity SW. Thus, our system may be a promising ablation device for ventricular tachyarrhythmias.
Study limitations
Several limitations should be mentioned for the present study. First, the mechanisms of deeper lesion effects with high-intensity SW remain to be fully elucidated. The cavitation effects could be one of the main mechanisms, which is supported the present finding that high-intensity SW caused extensive cavitation in ECF buffer and a number of micro-craters, presumably caused by micro-jets in SW lesion. Second, the long-term effects of the SWCA therapy remain to be elucidated. However, we consider that SW lesions are unlikely to be destabilized long after the therapy since the healing process occurs at early stage, leading to early stabilization of the lesions (Figure 7). Third, the SW application in the present study was not synchronized with heart beats. In the present study, we observed no ventricular arrhythmias by the R-on-T stimulation except extra-systoles. The synchronized equipment is under development in order to achieve safer stimulation and anticipated lesion depth. Forth, SW effects on the surrounding tissues (e.g. epicardial coronary arteries, oesophagus) remain to be fully elucidated. There was no macroscopic injury on the surrounding tissues in this study, although a high-intensity SW overpressure or excessive cavitation effects could cause injury. Fifth, validation of appropriate catheter direction to the tissue surface in the endocardial applications is difficult to confirm. Thus, our SW catheter has two polar electrodes at the tip for validating vertical catheter direction. In addition, we performed electrophysiological preliminary study with only one pig, as it required high-catheter operability and long-procedure time. However, with this experiment, we learned the future direction of our SW system for clinical use. Thus, we have been developing the catheter with higher operability in order to keep vertical direction to any endocardial tissue in cardiac chambers, and SW generator, which can be used stably several hours. We will continue to improve our SW catheter system to overcome this limitation. Sixth, due to electrophysiological vulnerability of pigs,22 we did not examine the difference in lesion depth between SW and RFCA with epicardial approach, although the current RF lesion depth was previously examined.3 Seventh, in the RF study, we were unable to use force controlled RF catheter, which is nowadays state of the art in RFCA.3 Further studies with force controlled irrigation RF catheter are needed. Finally, the present results were obtained only with normal domestic pigs. Thus, it remains to be examined whether the SWCA system is also useful and safe for the treatment of tachyarrhythmias in diseased heart models.
Conclusions
Our SWCA system may be superior to the RFCA in terms of lesion depth, myoendocardial injury, and tissue repair process. The SWCA system may be a promising option to compensate for the weaknesses of the current RFCA therapy.
Supplementary material
Supplementary material is available at Europace online.
Acknowledgement
We thank Yumi Watanabe, Ai Nishihara, Shokichi Hayasaka, Syun Sawada, Chika Tazawa, Yukihiro Inomata, Takashi Yonetsu, and Seiya Abe for their excellent technical assistance.
Funding
This work was supported by the grants-in-aid for the Translational Research Network Program and for Adaptable [Seeds B-17]; and Seamless Technology Transfer Program (A-STEP) through target-driven R&D [AS2313010F] from the Japan Agency for Medical Research and Development (AMED), Tokyo, Japan.
Conflict of interest: M.H., H.Y., and H.S. have stocks of the Shock Wave Medical, Inc. The other authors report no conflicts of interest regarding the present study.