-
PDF
- Split View
-
Views
-
Cite
Cite
Tomi Turner, Rebecca Wheeler, Ian Oliver, Assessing the Impacts of Land Spreading Water‐Treatment Residuals on the Anecic Earthworm Lumbricus terrestris, Soil Microbial Activity, and Porewater Chemistry, Environmental Toxicology and Chemistry, Volume 40, Issue 7, 1 July 2021, Pages 1962–1970, https://doi.org/10.1002/etc.5052
- Share Icon Share
Abstract
Water‐treatment residuals (WTRs), by‐products of drinking water clarification, are increasingly recycled to land to promote circular economy and reduce disposal costs, yet there is a lack of published literature on their effects on soil ecology. In the present study, the effects of WTRs on earthworm growth, soil respiration, and soil porewater chemistry were investigated throughout a 7‐wk outdoor mesocosm trial. We derived WTRs from both aluminum and iron coagulants and applied them to a loam soil at 0 to 20% (w/w). In addition, soil from a field that had received long‐term WTR applications and that of an adjacent nontreated reference field were included in the study. Earthworm mass increase was significantly higher in all but one laboratory‐treated soil when compared to the control. Furthermore, a linear regression model was used to predict increases in weekly soil respiration based on the application rates of both Al and Fe WTRs. In addition, a significant increase in soil respiration was observed from the treated farm soils during the first 4 wk of the trial. Measured sodium, magnesium, potassium, and iron porewater concentrations were higher in the treated farm soils than the reference site soil in a majority of samples, although these differences may be related to land management. Laboratory‐treated soils had elevated porewater arsenic concentrations (e.g., ~17 µg L–1 in controls vs ~62 µg L–1 in the 20% w/w Al WTR treatment in week 1), whereas porewater nickel concentrations were, respectively, elevated and lowered in Al WTR– and Fe WTR–amended samples. Overall, observed disturbances to soil ecology were determined to be minimal. Environ Toxicol Chem 2021;40:1962–1970. © 2021 The Authors. Environmental Toxicology and Chemistry published by Wiley Periodicals LLC on behalf of SETAC.
Abstract
INTRODUCTION
Water treatment residuals (WTRs) are a by‐product generated during treatment of drinking waters. Their main component is determined by the flocculants used in the treatment process, the most common of which are alum (aluminum sulfate), iron chloride, and iron sulfate, leading to WTRs being referred to as Al WTRs or Fe WTRs, respectively (Turner et al. 2019). Typically, drinking water purification produces approximately 10 to 30 mL of WTRs for every liter of water clarified (Dassanayake et al. 2015), and although current global production figures of WTRs are difficult to obtain, older estimates suggest that 10 000 t d–1 are produced globally (Waite and Dharmappa 1993). Water treatment residuals are regularly applied to land, which is considered to have environmental benefits, including a liming effect, adding organic matter, and the immobilization of a variety of contaminants and excess nutrients such as Cu, Ni, As, Cd, Pb, Zn, and P (Garau et al. 2014; Elkhatib and Moharem 2015; Nagar et al. 2015). However, although a number of studies have explored aspects of potential chemical impact of WTRs on the environment, very few have specifically explored the ecological impacts.
Earthworms are one of the most abundant terrestrial invertebrates in the temperate regions and important “ecosystem engineers.” They are well suited to use in monitoring potential contamination or other soil impacts because of the constant contact between their permeable skin and the surrounding soil, which makes them sensitive to changes in the chemical and physical soil properties (Paoletti et al. 1998; Roubalová et al. 2015). Indeed, Spurgeon and Hopkin (1999) demonstrated that earthworm abundance and biomass decreased with proximity to a Pb/Zn/Cd smelting works in the United Kingdom. However, little research has been done to date on the effects on earthworms following soil amendment with WTRs, although an initial short‐term (14‐d) study by Howells et al. (2018) found that earthworms exposed to 0 to 20% w/w WTR‐amended soils did not demonstrate effects on biomass, survival, or reproduction. However, the same study found that earthworms avoided soils amended to ≥10% w/w of Fe WTRs and to 20% w/w Al WTRs, which together with a lack of published data relating to potential ecological impacts warrants further investigation.
The influence of land application of WTRs on soil microbial activity is also yet to be fully understood. It is known that WTRs themselves are a source for microorganisms such as Proteobacteria, Cyanobacteria, Bacteroidetes, Firmicutes, Verrucomicrobia, and Planctomycetes (Würzer et al. 1995; Oliver et al. 2011; Xu et al. 2018); but the overall effect of WTR addition is still not certain. Pecku et al. (2006) found that an application of 300 t ha–1 of WTRs had no detrimental effect on soil respiration or microbial diversity. However, mixed results were obtained by Garau et al. (2014) when applying Fe WTR amendments (3% w/w addition rate), with an increase in the amount of culturable heterotrophic bacteria and actinomycetes and a decrease in the amount of heterotrophic fungi. They concluded that the overall microbial biomass of samples remained approximately constant, although the suite of species present changed. A commonly employed method of estimating overall microbial activity is the measurement of soil CO2 efflux. In soils, CO2 is primarily released through microbial decomposition of soil organic matter, whereas a few percent are caused by root interactions (e.g., root respiration and rhizo‐microbial respiration) and chemical oxidation of organic matter (Raich and Schlesinger 1992; Kuzyakov 2006; Smith et al. 2008). Therefore, monitoring CO2 efflux can reveal changes in microbial activity following amendments and other treatments.
The potential for WTRs to leach constituent elements, particularly Al, into the surrounding environment is often considered to be the greatest concern in relation to land application of WTRs. In some countries this is accounted for in legislation; for example, in England and Wales the application of Al WTRs and Fe WTRs is limited to soils above a pH of 6.0 and 5.0, respectively, because of the increased mobility of Al and Fe in soils at low pH (Environment Agency 2013). The importance of these restrictions was highlighted by Howells et al. (2018), who found that the amount of leachable (0.001 M CaCl2) Al from the Al WTRs increased from 4.5 to 382 mg kg–1 when decreasing the pH from 5.5 to 4.4.
The present study aimed to confirm that addition of WTRs to soil does not alter the porewater chemistry (in terms of metal and metalloid concentrations) to any substantial degree that would inhibit plant or soil biota health and that no negative effects on earthworm growth occur. Furthermore, we aimed to test for the first time how WTR addition affected overall microbial activity measured by microbial respiration.
MATERIALS AND METHODS
Soils and WTRs
Soil treatments were prepared in the laboratory by adding Al WTRs or Fe WTRs to a commercially supplied natural soil, Kettering loam (supplied by Boughton). This soil was steam‐sterilized before purchase (~4 yr prior to use) and then stored under cover outdoors. Kettering loam was selected because it has been previously used in earthworm studies and is known to be suitable for a range of soil‐dwelling species (Butt 2002; Rajapaksha et al. 2014; Brami et al. 2017). In addition, sandy clay soils were collected from 2 adjacent agricultural fields in south Wales to compare the impacts of long‐term WTR application in a parallel study. One field had received heavy applications of WTR solids for many years (most recently 135 t ha–1 in 2015 and 92 t ha–1 in 2016), whereas the other acted as a control and had received no (or only incidentally) applied WTRs (henceforth referred to as “farm‐treated” and “farm‐reference” soils, respectively). It is worth noting that the treated area had more commonly been used as arable land, whereas the reference area had more commonly been used for pasture. These soils were all dried at 105 °C and sieved to 4 mm to ensure sample homogeneity. The WTRs used in the present study were sourced from treatment plants in the southwest of the United Kingdom. The Fe WTRs came from the same water‐treatment plant as the WTRs applied to the treated farm soil. Per the soils, the WTRs were dried at 105 °C and crushed using a jaw crusher before being sieved (to 2 mm).
The water content of the WTRs, as received, was 25.85 and 65.27% (w/w) for the Al and Fe WTRs, respectively. Soils and WTRs were characterized for pH by 1:5 solid to deionized water suspensions, organic matter by loss on ignition at 550 °C, and water holding capacity (WHC) by saturation and drainage (Table 1).
Properties of the soils and water‐treatment residuals used in the present study
Sample | pH | Organic content (%) | Water holding capacity (mg g–1) |
Farm treated soil | 7.05 ± 0.00 | 12.09 ± 0.07 | 0.76 |
Reference farm soil | 5.79 ± 0.01 | 12.05 ± 0.26 | 0.60 |
Kettering loam | 7.65 ± 0.02 | 16.05 ± 0.11 | 0.64 |
Fe WTR | 7.48 ± 0.01 | 24.81 ± 4.56 | — |
Al WTR | 6.2 ± 0.04 | 66.93 ± 0.72 | — |
Sample | pH | Organic content (%) | Water holding capacity (mg g–1) |
Farm treated soil | 7.05 ± 0.00 | 12.09 ± 0.07 | 0.76 |
Reference farm soil | 5.79 ± 0.01 | 12.05 ± 0.26 | 0.60 |
Kettering loam | 7.65 ± 0.02 | 16.05 ± 0.11 | 0.64 |
Fe WTR | 7.48 ± 0.01 | 24.81 ± 4.56 | — |
Al WTR | 6.2 ± 0.04 | 66.93 ± 0.72 | — |
WTR = water‐treatment residual.
Properties of the soils and water‐treatment residuals used in the present study
Sample | pH | Organic content (%) | Water holding capacity (mg g–1) |
Farm treated soil | 7.05 ± 0.00 | 12.09 ± 0.07 | 0.76 |
Reference farm soil | 5.79 ± 0.01 | 12.05 ± 0.26 | 0.60 |
Kettering loam | 7.65 ± 0.02 | 16.05 ± 0.11 | 0.64 |
Fe WTR | 7.48 ± 0.01 | 24.81 ± 4.56 | — |
Al WTR | 6.2 ± 0.04 | 66.93 ± 0.72 | — |
Sample | pH | Organic content (%) | Water holding capacity (mg g–1) |
Farm treated soil | 7.05 ± 0.00 | 12.09 ± 0.07 | 0.76 |
Reference farm soil | 5.79 ± 0.01 | 12.05 ± 0.26 | 0.60 |
Kettering loam | 7.65 ± 0.02 | 16.05 ± 0.11 | 0.64 |
Fe WTR | 7.48 ± 0.01 | 24.81 ± 4.56 | — |
Al WTR | 6.2 ± 0.04 | 66.93 ± 0.72 | — |
WTR = water‐treatment residual.
Mesocosm setup
Experiments were conducted in semifield conditions using purpose‐built outdoor mesocosms. These consisted of cylindrical pots with a depth of 12.5 cm and a diameter of 14.5 cm. Drainage holes were drilled in the base of the pots. Velcro was attached to the inner rims of the pots to discourage earthworm escape. The Kettering loam soil was hand‐mixed with the Al or Fe WTRs at rates of 0, 5, 10, and 20% by dry weight. The 5% WTR application rate was selected as an upper level of what is likely to be used in land spreading practices on agricultural soil (i.e., 5% equates to ~120 t ha–1, assuming a soil density of 1.2 g cm–3 and depth of 20 cm), whereas the 10 and 20% application rates were selected as extremes to determine the extent of application required to bring about ecological effects. For reference, within England and Wales, WTR application is limited to 250 t ha–1 per annum, although this is often further reduced for WTRs with high solid content. Each mesocosm was filled with 1.5 kg of the corresponding substrate. Farm and laboratory soils were wetted to 60 and 50% WHC, respectively. Once installed outdoors the water content was allowed to fluctuate naturally and checked weekly to ensure that they did not dry out.
Ten sexually mature (visible clitellum) Lumbricus terrestris earthworms were rinsed with deionized water, patted dry, weighed, and then placed in each pot (earthworms were originally sourced from Yorkshire Worms). On average, there was 24.0 g of earthworms per kg of substrate in each mesocosm. This density of earthworms is lower than the 50 to 60 g of soil per earthworm specified in International Organization for Standardization and Organisation for Economic Co‐operation and Development protocols for earthworm studies but is in keeping with rates recommended by others for long‐term tests (e.g., Bart et al. 2018). Lumbricus terrestris is a species of earthworm that falls within the anecic ecological subgroup. Anecic earthworms characteristically create and live within permanent vertical burrows. This species was chosen because it is commonly found in mineral soils, unlike other species often employed in ecotoxicology assays such as Eisenia fetida and Eisenia andrei that generally live in high–organic matter substrates such as composts and litters. Rhizon samplers (Rhizosphere Research Products) were installed at 5 cm depth in all mesocosms. Once prepared, mesocosms were sown with 3 g of ryegrass (Lolium perenne) seeds to create an environment that reflected a pasture soil scenario and would act as a food source for the earthworms. The mesocosm treatments thus included 2 farm soils, 6 laboratory‐amended soils, and one control soil (nonamended Kettering loam; Figure 1). Four replicates were prepared for each, resulting in 36 mesocosms being assembled in total. For the duration of the study the mesocosms were situated in an enclosed (fenced‐off) outdoor site. Mesocosms were elevated off the ground on wooden frames with plastic mesh around them to prevent access to birds and other wildlife but otherwise keep conditions consistent with field conditions (i.e., natural field temperatures and rain conditions for central United Kingdom during October–November 2018; Figure 2).
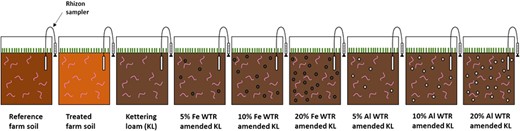
A summary of the different mesocosm substrates prepared for the present study. KL = Kettering loam; WTR = water‐treatment residual.
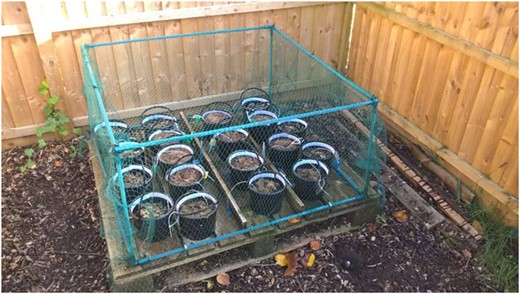
A photo of the outdoor setup used for holding mesocosms during the present study.
Earthworm, porewater, and CO2 flux measurements
Earthworms were recovered from mesocosms at the end of the experiment (after 49 d) via hand sorting. They were washed and weighed in the field to measure their average weight for comparison to weights before the experiment. The change in average weight of earthworms was chosen as an indicator of earthworm health.
Porewater samples were collected weekly over a 5‐wk period via the installed rhizon samplers. These samplers were comprised of a porous ceramic‐like filter attached to a polyvinyl chloride tube through which water can be extracted using a syringe under vacuum conditions. Collected samples were acidified with analytical‐grade HNO3 and analyzed via inductively coupled plasma mass spectrometry (ICP‐MS; Agilent 7500ce) along with certified solution standards. Soil porewater sampling has many benefits compared to other measures of element bioavailability (e.g., extraction with neutral salt solutions) because it directly samples the solution that plant roots and soil invertebrates experience and does not rely on an artificial reagent to displace solutes. Moreover, the rhizon sampler method allowed repeated samples to be taken in a nondestructive manner.
Flux of CO2 was measured weekly over a 6‐wk period using a PP Systems EGM‐5 Portable CO2 analyzer. This method works by placing the device's chamber (surface area of 78.5 cm2) on the soil surface to produce an airtight seal; then, air is pumped through the chamber, and the difference between CO2 concentrations in the inflowing and outflowing air streams determines the CO2 flux from the soil. The CO2 flux measurements were conducted over a 60‐s period for each sample after a 15‐s purge time and a 12‐s equilibration time.
Additional data sources and statistical methods
Elemental analysis of WTRs was conducted by a certified, commercial laboratory via US Environmental Protection Agency Method 3050B (US Environmental Protection Agency 1996), following standard quality assurance/quality control protocols (see Supplemental Data, Table 1). In summary, 0.5 g of dried material was digested in 12 mL of aqua‐regia (9 mL HCl + 3 mL HNO3) in a hot‐block digestion set at 125 °C. The digestate was then diluted to 50 mL with deionized water, and elemental concentrations were determined by either ICP‐optical emission spectroscopy or ICP‐MS depending on the concentration present. Meteorological data were collected from a weather station situated approximately 500 m from the site of the experiment, allowing highly accurate hourly weather data to be obtained. All data were processed, analyzed, and statistically assessed using Microsoft Excel and Minitab, employing linear regressions, t tests, and analyses of variances (ANOVAs) following appropriate checks for adherence to normality and associated underlying assumptions.
RESULTS
Earthworm weight change
When assessed with a standard ANOVA approach, there were no significant differences found between the weight changes of earthworms in treatments and controls for any of the farm‐treated or laboratory‐amended soils (Al WTR treatments vs control ANOVA, p = 0.064; Fe WTR treatments vs control ANOVA, p = 0.095; farm‐treated vs reference soil t test, p = 0.264). However, prompted by apparent visual trends (Figure 3), assessment via one‐sample t tests revealed significantly higher earthworm mass increases in all of the Al WTR–treated soils and the 5 and 20% Fe WTR–treated soils compared with the control (p < 0.05); but for the treated farm soils there were still no detectable significant differences from the reference soil (p = 0.20). A statistically significant positive relationship was also identified by linear regression analysis between Fe WTR addition (percentage w/w) in laboratory‐amended soils and earthworm mass increase, although only a low proportion of the variance could be accounted for by this model (R 2 = 0.30, p = 0.027).
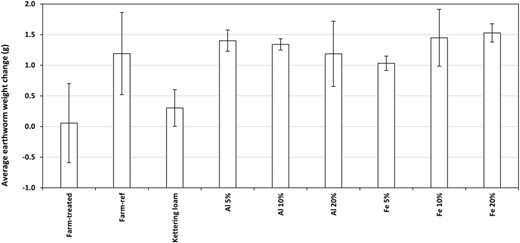
The average change in earthworm weight after 49 d; error bars display 1 standard error.
CO2 flux (soil respiration)
Field‐treated farm soils
There was a significant difference between CO2 fluxes of the treated and reference farm soil mesocosms in weeks 1 to 4 but not in weeks 5 and 6, with the treated farm soils having a higher CO2 efflux in every case (t test, p < 0.05; Figure 4).
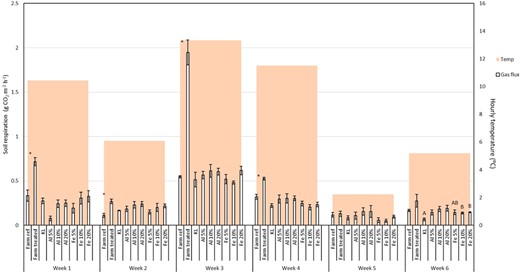
A summary of CO2 flux measurements and air temperature during the 6 wk of sampling. Error bars display 1 standard error; *significantly different samples; A and B indicate significant difference groupings. KL = Kettering loam.
Laboratory‐amended soils (Kettering loam)
When examined on a weekly basis, the only significant difference in CO2 flux observed following laboratory additions of Al or Fe WTRs to Kettering loam was recorded during week 6 under Fe WTR application (ANOVA, p > 0.05 in every other case; Figure 4). Multiple linear regression analysis of the overall data set indicated that the main predictor of gas flux was air temperature (p < 0.001, R 2 = 0.60). However, when considering the whole data set on an independent weekly basis (allowing a degree of normalization for air temperature), regression results indicated that the application rate of Al and Fe WTRs could be used to predict the CO2 flux of soils (p = 0.004 and 0.018, respectively) and accounted for a large amount of the variance (R 2 = 0.7405 and 0.7782, respectively). Regression equations for Al WTR– and Fe WTR–amended CO2 flux can be seen in Equations 1 and 2, respectively.
In the equations, c is a constant that varies from week to week, and A is the application rate expressed as a dry weight percentage.
Porewaters
Farm soils (field‐treated and reference)
Porewater element concentrations were assessed week by week and evaluated for differences between the field‐treated and reference farm soils via t test comparisons. Interestingly, the Al concentrations of porewaters were not significantly different. Concentrations of Cu, Zn, As, and Pb only differed significantly (treated vs reference soil) during 1 wk over the entire sampling period; however, these were all lower in the treated soils, aside from Pb which was marginally enriched in treated soils (0.3 vs 0.07 µg L–1 in treated vs reference farm soils during week 3; Supplemental Data, Table 2). More notably, Na, Mg, K, Mn, and Fe concentrations differed significantly during at least 3 wk of the study (Figure 5). In every case, these 5 elements were elevated in treated soils; for example, Fe concentrations were 339 and 8062 µg L–1 during week 4 in reference and treated farm soils, respectively.
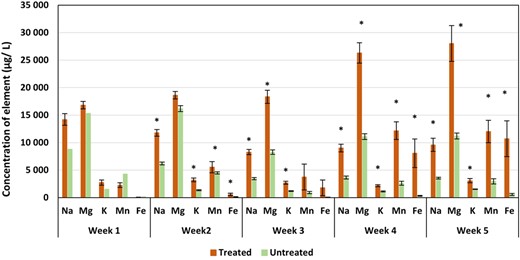
The concentrations of elements of interest in porewaters from farm soils, with significant differences noted by asterisks; error bars represent 1 standard error.
Laboratory‐amended soils (Kettering loam)
The Al concentrations in porewaters of Al or Fe WTR laboratory‐amended soil did not differ significantly from controls (Figure 6), nor did the concentrations of Fe except for under Al WTR application in week 5 (Figure 6). However, additions of Al WTRs significantly elevated the As concentration compared with the untreated control in every week except week 3 (Figure 7), with the increase typically being at least 3‐fold at the highest application rate (e.g., from ~17 µg L–1 in the control to ~62 µg L–1 in the 20% Al WTR treatment in week 1). The addition of Fe WTRs also increased porewater As concentration in 3 of the weeks during which porewaters were monitored, but the increases were more modest than in the case of Al WTRs (Figure 7). In addition, in the case of Fe WTR–treated soils, Ni concentrations in porewaters were elevated during the same weeks that As enrichment was observed, by a factor of 1.3 to 2.0 (Figure 7). Contrastingly, Al WTR–treated mesocosms had reduced Ni concentrations in every week of sampling. Other elements, particularly Cr, were also either decreased in concentration or unaffected by Al and Fe WTR addition. The large increases in Fe concentrations observed in the amended farm soils were not replicated in Kettering loam amended with Fe WTRs in the laboratory.
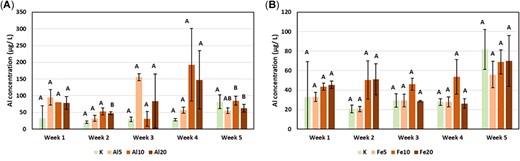
Mean concentration (micrograms per liter) of Al in Al water‐treatment residual (WTR)– (A) and Fe WTR– (B) amended Kettering loam soil. Error bars display 1 standard error.
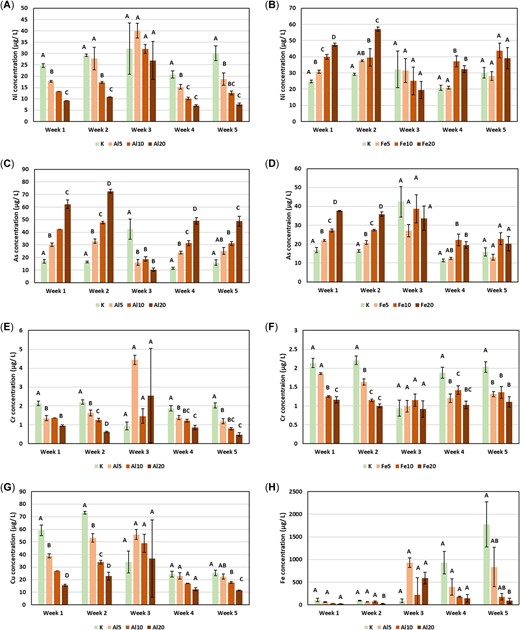
Mean concentration (micrograms per liter) of nickel (A, B), arsenic (C, D), chromium (E, F), copper (G), and iron (H) in porewaters of Al water‐treatment residual (WTR) and Fe WTR treated Kettering loam soil. Letters indicate analysis of variance groupings. Error bars display 1 standard error.
DISCUSSION
Earthworm mass increase was found to be higher in the laboratory‐amended soils, which contrasts with results from laboratory experiments reported by Howells et al. (2018), who applied WTRs at similar application rates (0, 5, 10, and 20% by weight) and found that there was no significant difference in the growth rate of a different earthworm species (Eisenia fetida). Between the present study and that of Howells et al. (2018), 2 of the 3 principal earthworm subgroups (anecic and epigeic) are covered; and therefore, there can be a degree of confidence that WTR application is unlikely to have any negative impacts on earthworm growth when they are applied at typical rates. The positive relationships between WTR application rate and earthworm weight increase found in the present study may be due to the organic matter additions from WTRs. The fact that the farm‐treated soils showed no difference in earthworm growth might indicate that any enhancement generated by WTR addition has a time‐limited effect.
The subtler differences between laboratory‐amended and nonamended Kettering loam gas fluxes when compared with those between the treated and reference farm soils could indicate that previous soil conditions and management practices of the farm soils may also have played a role in the differences in flux observed or that the pretreatment of the Kettering loam including steam sterilization approximately 4 yr prior to use may have influenced the microbial response observed during the experiment. The surface WTR amendments may have also led to indirect effects on CO2 flux because of changes in WHC, bulk density, and albedo. However, regression analysis in the present study did indicate that Fe and Al WTRs could influence soil respiration at higher application rates. These results differ from those reported by Mukherjee et al. (2014a, 2014b), who found no effect on CO2 emissions in soils amended with low rates of WTRs (0.5% w/w), but are consistent with those from Pecku et al. (2006), who observed a general increase in CO2 flux after higher applications rates of WTRs (up to 25% w/w) when measured in 24‐h jar incubation experiments. It is possible that the increase in organic matter and/or alteration or stimulation in the microbial community introduced by the addition of WTRs may contribute to differences in gas flux (and therefore microbial activity) over longer periods or at higher application rates, and therefore, understanding the underlying mechanisms (including changes to microbial species suites) is an avenue for further research.
Difference in the porewater Al and Fe concentrations of laboratory‐amended and nonamended Kettering loam soils were rarely statistically significant (the only exception is Fe in week 5; Figure 7H), suggesting that both Al and Fe from the WTRs are nonleachable under these conditions. This is likely, in part, due to the pH of the soils, which was >5.5 in all cases (i.e., above the point where Al and Fe become more readily mobile). The As concentration, on the other hand, was notably higher in the WTR‐amended Kettering loam soils (in the region of ~13–70 µg L–1 at all application rates). The As concentrations in the present study were below typical toxicity thresholds reported for porewater As; however, the highest level observed was comparable to the 50% effect concentration for cucumber plants recorded in one sensitive soil from Australia (60 µg L–1 [Kader et al. 2017]). Certain regulations in some jurisdictions require WTRs to be periodically analyzed for elemental content if they are to be applied to land, and such analysis can be used to set limits on how much can be applied. This is the case in the United Kingdom, where the WTRs used in the present study were obtained; and previous unpublished analysis of Al WTRs from the same water‐treatment plant identified that As is an element that can limit the amounts of the material that can be applied to land under those regulations. The porewater results of the present study indicate that such a limit is a prudent precaution because they demonstrate that As in these WTRs is potentially mobile to some degree. However, regional differences in WTR composition and properties must be considered because past studies have produced mixed results. For example, a study by Chiang et al. (2012) found that during sorption/desorption tests of goethite and WTR mixtures the leaching of As was proportional to the WTR content of the blend (i.e., WTRs contributed leachable As). However, Al WTRs from elsewhere have been shown to significantly reduce As mobility in treated soils (see Garau et al. 2014; Silvetti et al. 2014). Neither the modest release of Ni by Fe WTRs nor its sorption by Al WTRs, as observed in the present study for the laboratory‐amended soils, have been documented in the literature. In contrast to the Kettering loam laboratory‐amended soils, the field‐treated farm soils showed no changes in porewater Ni concentrations. Indeed, there were few examples of similar trends in porewater chemistry between the laboratory‐treated Fe WTR‐Kettering loam samples and the treated farm samples. It is possible that differences in the rates of WTR application and previous soil management at the farm, in addition to any aging effects and mineralogical composition, could have given rise to the dissimilar porewater results. Aging effects have been reported by Agyin‐Birikorang and O'Connor (2009), who found that Al mobility decreased in WTRs over time; and a similar process could potentially also occur for other elements. However, to our knowledge, no further investigation has been done on the subject. Reductions in other porewater elemental concentrations, particularly Cu in Al WTR–amended samples and Cr in all of the amended samples, can be accounted for by the high sorption capacity of WTRs. For example, Zhou and Haynes (2011) established that, even at a low pH of 5, Al WTRs added to test solutions (10 g L–1) could sorb up to 114 g kg–1 Cr and up to 52 g kg–1 Pb at the highest metal doses imposed. Similarly, Ngatenah et al. (2010) found that 100% of the Cu in a 65 mg L–1 Cu solution was removed using 2 g L–1 of ground WTRs. Meanwhile, Soleimanifar et al. (2016) found that 81% of a 100 μg L–1 dose of Cu was sorbed by WTR‐coated mulch (≥1:3 WTR to mulch w/w) over a period of 120 min. It is possible that the presence of earthworms and their degradation of organic matter may have influenced the mobility of some elements (Sizmur et al. 2011).
CONCLUSIONS
Mean earthworm mass increase was significantly enhanced in the majority of the laboratory‐amended soils, although no significant differences were discernible between soils treated with WTRs previously on the farm and the nontreated farm reference soils. When examined across the whole data set, a small positive association between fresh WTR additions was identified; investigation over a longer period may help provide a better picture of these effects.
Soil porewater Al was not appreciably affected by WTR addition in either the freshly applied or field‐applied and aged samples, indicating that Al leaching is not likely to be a concern with these WTRs under normal field conditions. Porewater As content was largely unaffected in the farm‐treated soils but was increased in the fresh laboratory‐amended soils when additions far above regular agricultural practices were made (up to ~70 µg L–1 at the highest rate of Al WTR application), and this warrants further research. Freshly applied Fe WTRs also appeared to be a minor source of soluble Ni, but this was not observed in the farm‐treated soils, suggesting that this effect may reduce over time. The results indicate that the elemental mobility in freshly treated soils and in aged, treated soils may vary. Generally, the leachable amounts determined in the porewaters represent a tiny fraction of the total element contents (see Supplemental Data, Table 1), indicating low mobility of elements within the WTRs. Nevertheless, longer‐term and/or intensive leaching studies are warranted to confirm that this remains the case over time.
At the rates that WTRs are commonly applied and considering the bounds of normal field conditions, the application of WTRs is unlikely to have a negative impact on earthworms or soil respiration, although there is still scope for longer‐term experiments to be conducted.
Supplemental Data
The Supplemental Data are available on the Wiley Online Library at https://doi.org/10.1002/etc.5052.
Acknowledgment
The present study was funded by the UK National Productivity Investment Fund (Natural Environment Research Council grant NE/R007314/1). We gratefully acknowledge the assistance of the landowners where samples were collected.
Author Contributions Statement
T. Turner conducted the experiment and wrote the manuscript; R. Wheeler provided expertise in the waste disposal industry; I. Oliver conceived the experiment and assisted in editing the manuscript.
Data Availability Statement
Data, associated metadata, and calculation tools are available from the corresponding author ([email protected]).
REFERENCES