-
PDF
- Split View
-
Views
-
Cite
Cite
Takahiro Kondo, Advancements in Freestanding Hydrogen Boride Sheets: Unraveling the Novel Properties of Borophane Polymorphs, Chemistry Letters, Volume 52, Issue 7, July 2023, Pages 611–621, https://doi.org/10.1246/cl.230210
- Share Icon Share
Abstract
Hydrogen boride (HB) sheets are two-dimensional materials and polymorphs of hydrogenated borophene (borophane) composed of boron and hydrogen in a 1:1 stoichiometric ratio. Freestanding HB sheets can be synthesized via ion exchange reactions between the protons and magnesium cations in magnesium diboride. Several unexpected properties of the HB sheets have been reported. Here, the recent progress in HB sheets is reviewed.
Introduction
Two-dimensional (2D) materials such as graphene and transition metal dichalcogenides possess unique electronic, optical, and thermal properties.1–3 The combination of 2D materials and van der Waals heterostructures imparts new properties4,5 and provides new designs for electronic devices.6,7 In addition, 2D materials exhibit various interesting properties, such as superconductivity, when stacked with other 2D materials with specific twist angles.8 Consequently, 2D materials have the potential to be used in a wide range of applications, including catalysts and electronic devices.9–12 Among the numerous reported 2D materials, boron-based 2D materials have polymorphic nature.13,14 Both single-layer boron (borophene) and single-layer hydrogenated boron (borophane) have been experimentally shown to exhibit polymorphism on Ag(111) surfaces.15–17 In addition to the individual functional properties of boron-based 2D materials,18,19 their polymorphic nature is highly attractive, because it offers numerous opportunities for combining with materials to create new functionalities.
In this review, we focus on one borophane polymorph, hydrogen boride (HB). HB sheets have been synthesized as freestanding metal-free sheets composed of boron and hydrogen in a 1:1 stoichiometric ratio in powder form without a substrate.20 Several intriguing properties, including theoretically unexpected properties, have been reported. This review summarizes the recent progress in HB sheets.
Formation of HB Sheets
Figure 1 shows a schematic of HB sheet formation. It can be obtained by mixing MgB2 and an ion-exchange resin in acetonitrile (or methanol) at room temperature (∼300 K) in a nitrogen atmosphere at atmospheric pressure (∼1 atm), removing the precipitate, and drying it.20 It is known that MgB2 undergoes hydrolysis in water, which results in the formation of Mg(OH)2 and “Mg-deficient hydroxyl-functionalized boron sheets” together with boric acid.21,22 Therefore, to obtain HB sheets in good yield, suppressing the hydrolysis reaction of MgB2 is essential, which can be realized by performing the ion exchange without exposure to water or air. The resulting average production yield of HB sheets was reported to be 42.3%, concerning the expected production amount of the chemical equation shown in Figure 1.20 One of the origins of the smaller production yield was impurities in the MgB2 starting material such as unreacted boron, boron oxide, and magnesium oxides. Notably, such impurities can be separated in the formation process of HB sheets without conducting pre-treatment for MgB2. Thus, the purity of HB sheets is high even using impurity containing MgB2 (Purity of HB sheets: 100% Bδ− species and no Mg signal in X-ray photoelectron spectroscopy (XPS), as reported previously).20,23 It has been found that the ion-exchange rate can be improved by mixing with formic acid, where acid is considered a proton mediator enhancing the ion-exchange reaction.24
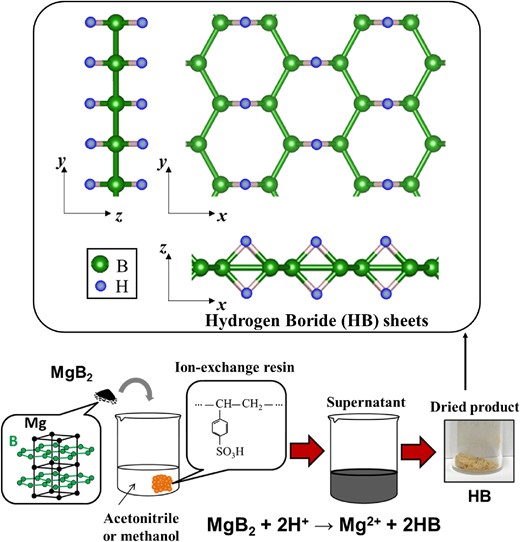
Schematic of hydrogen boride (HB) sheet formation. Adapted with permission from Ref. 20. Copyright 2017 American Chemical Society.
Characterization of HB Sheets
HB sheets have been characterized by microscopy and spectroscopy (Figures 2, 3, and 4), as well as structural analysis of the X-ray pair distribution function (XPDF) together with first-principles calculations based on density functional theory (DFT).20,23 Here, fundamental results are introduced.
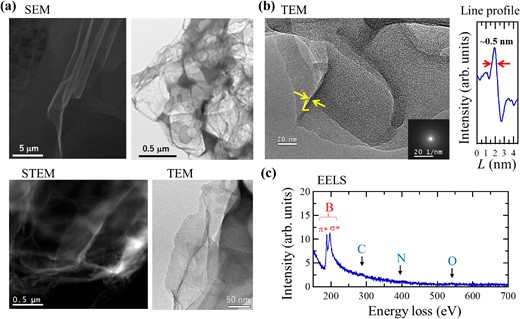
(a) Scanning electron microscopy (SEM), transmission electron microscopy (TEM), and scanning transmission electron microscopy (STEM) images; (b) TEM image and line profile (bottom right: electron beam diffraction image); and (c) Electron energy loss spectrum (EELS) of an HB sheet. Reprinted with permission from Ref. 20. Copyright 2017 American Chemical Society.

Atomic force microscopy (AFM) images and line profiles after spin coating and drying HB sheets dispersed in acetonitrile onto mica substrate. Adapted with permission from Ref. 23. Copyright 2020 Elsevier.
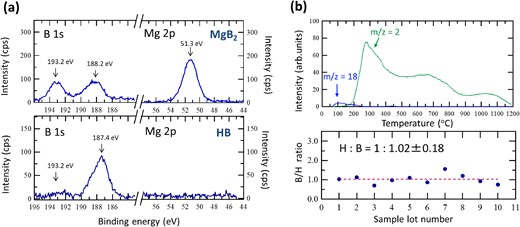
(a) X-ray photoelectron spectroscopy results for MgB2 and HB sheets. (b) B/H ratios of different sample lots (top) calculated from thermal desorption spectroscopy (TDS) results for HB sheet (bottom). Adapted with permission from Ref. 20. Copyright 2017 American Chemical Society.
When the HB sheet powder was observed using scanning electron microscopy (SEM), transmission electron microscopy (TEM), or scanning transmission electron microscopy (STEM), a wrinkled sheet structure was observed, as shown in Figure 2a. Under further magnification, the sheet structure remains, and according to the line profile of the cross-section, it has a thickness of 0.5 nm, which corresponds to the thickness of a single monatomic layer (Figure 2b). In the electron energy loss spectrum (EELS) measured simultaneously with the TEM image, only peaks originating from boron were observed, with no peaks for carbon, oxygen, or nitrogen (Figure 2c). Furthermore, the boron peak exhibits a structure consisting of π* and σ* peaks, which correspond to the sp2 bonding structure and are characteristic of a planar structure. This agrees well with the SEM and TEM images in terms of the two-dimensional characteristics of the HB sheets.
Figure 3 shows an atomic force microscopy (AFM) image of the HB sheets prepared on a mica substrate by spin coating followed by drying the HB sheets dispersed in acetonitrile.23 A sheet with a height of approximately 1 nm and width of approximately 100 nm, as in the line profile a-a′, was observed near the center of rotation of the spin coating. The height and width increased in the order b-b′, c-c′, and d-d′ as the distance from the center of rotation of the spin-coating increased, e.g. a sheet with a height of approximately 4 nm and a width of approximately 1300 nm was observed at c-c′. In all cases, the width was approximately one to two orders of magnitude larger than the thickness, indicating that a two-dimensional sheet morphology was obtained, corresponding to the TEM and SEM images shown in Figure 2.
It is well known that X-ray photoelectron spectroscopy (XPS) core-level binding energies reflect the charge states of surface elements in terms of chemical shift. From the XPS B 1s spectra, the charge states of surface boron atoms before and after the ion exchange process were determined. Figure 4a shows the XPS results for the starting materials of MgB2 and HB sheets obtained after ion exchange. It is clearly shown that the photoelectron peak originating from Mg (Mg 2p) disappears after ion exchange. The photoelectron peak of boron (B 1s) at approximately 188 eV indicated that B was negatively charged, which was dominant even after ion exchange (the B 1s peak positions reported in the literature20,25–48 are summarized in Figure 5). These results suggest that the positively charged Mg ions undergo ion exchange with protons. Notably, the photoelectron peak at 193.2 eV in the starting material originates from the oxide on the MgB2 surface. The surface oxide of MgO on MgB2 overlaps with the positively charged Mg peak at 51.3 eV. These oxide impurities can be separated during the sample preparation as sediments, as described above.20 Figure 4b shows the results of thermal desorption spectroscopy (TDS) in a vacuum to investigate the amount of hydrogen in the sample after ion exchange.20 A large amount of hydrogen molecules was released over a wide temperature range of 423 to 1473 K. Factors in the wide range of multiple TDS peaks are currently being analyzed. However, from the results of XPS and infrared spectroscopy (IR) performed along with heating, it is thought that structural changes to a stable structure occur with hydrogen release via multiple stages (two or more) corresponding to the number of peaks. From TDS spectra, the composition of the samples was examined: from the total amount of hydrogen molecules released and the weight of the sample before heating for each of the separately prepared 10 different lot samples, the elemental ratio of the HB sheets was determined to be H:B = 1:1.02 ± 0.18 (bottom of Figure 4b). These results show that HB sheets with an H:B ratio of 1 were formed by the following stoichiometric ion-exchange reaction at room temperature as shown by chemical equation in Figure 1.
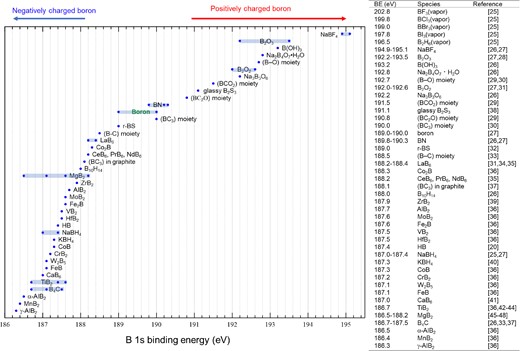
B 1s binding energies for several compounds reported in the literature.32 The vertical axis has no special meaning.
Note that this chemical equation is also consistent with produced HB charge states examined: (1) boron species in the material is negatively charged (Figures 4a and 5), (2) Hammett acidity function (H0, acid strength) of the HB sheet is below 1.5 and above 0.43,20 (3) Acidity constant (pKa, Ka is acid dissociation constant) estimated from pH change in water with the following assumption: Ka = [H+][B−]/[HB] ([H+], [B−], and [HB] are molar density of H+, B−, and HB, respectively) is 3.5 ± 0.2,49 (4) the HB sheets act as a solid acid catalyst,50,51 and (5) Löwdin charges of H and B in HB estimated from DFT calculation are 0.79 (below 1) and 3.14 (above 3), respectively.49 Thus, the HB sheets were named “hydrogen boride (HB)” in 2017 rather than the traditional borane name of “boron hydride (BH)”. By comparing the electronegativities of boron and hydrogen, the electronegativity of hydrogen (2.20) is higher than that of boron (2.04). Therefore, charge transfer from B to H atoms seems plausible. Indeed, charge transfer from silicon (1.90) to hydrogen (2.20) atoms has been observed in hydrogenated silicene.52 However, although the electronegativity of carbon (2.55) is higher than that of hydrogen (2.20), charge transfer from H atoms to C atoms has been observed in graphane.53 Thus, the charge state cannot be determined solely based on the electronegativity difference. In the case of HB sheets, the boron energy position is deeper than that of a single boron atom because of the formation of a large boron network with sp2-like bonding configurations (boron–boron hybridizations)54–56 and the boron atoms bond with hydrogen atoms via three-center two-electron bonds, which are composed of occupied hydrogen orbitals and the occupied and unoccupied orbitals of boron. Consequently, the boron network is negatively charged.49
The HB sheets did not exhibit any peaks in powder X-ray diffraction and exhibited an amorphous state in electron beam diffraction using TEM, as shown in Figure 2b. However, XPDF analysis and first-principles calculations of B 1s indicate that among the multiple stable structures consisting of H and B from previous theoretical predictions,54,55,57 the local structure of an HB sheet is one in which three-center two-electron bonds form such that the hydrogen is cross-linked in the backbone of boron six-membered rings,54,55 as shown in Figure 1. The reason for the lack of long-range order is ascribed to the presence of three different anisotropic domains originating from the 2-fold symmetry of the hydrogen positions against the 6-fold symmetry of the boron networks.20 High-precision analyses of the pure HB sample, including XPDF analysis and infrared spectroscopy, have shown that the hydrogen in actual HB sheets not only forms B–H–B bonds but also terminal B–H bonds and has a local structure with B–H–B bonds on one side and B–H bonds on the other side, instead of only B–H–B bonds on both sides.23
HB sheets were found to be chemically stable in water.49 Experiments revealed that mixing HB sheets and water produces negligible amounts of hydrogen, suggesting that hydrolysis does not occur and that HB sheets are chemically stable in water, which is in contrast to most boron hydride materials, such as B2H6 gas. First-principles calculations revealed that the HB sheets interact weakly with water, even in the presence of defects, and that negatively charged boron prevents the onset of hydrolysis. The charge state of boron and the covalent B–B bond network have been reported to be responsible for its chemical and structural stability.49 Note that chemical stability of HB sheets against water is a great advantage for applications such as hydrogen storage material in terms of safety, which is in sharp contrast to the case of general metal hydride materials (water-reactive substances).
A quite recent report shows that pure HB sheets prepared by the pre-treatment with water can preserve 100% Bδ− states even over nine months stored on a desk facing a window,58 indicating that HB sheets have a certain stability. HB sheets contain as much as about 8.5 wt % hydrogen and experimentally roughly estimated weight density of HB sheets is as much as 1.26 ± 0.10 g cm−3.58 Thus, HB sheets are expected to be safe, light, and compact solid hydrogen carrier materials.
Intriguing Properties of HB Sheets
Before the experimental report on the formation of HB sheets in 2017,20 the material was theoretically predicted in 2011 by Abtew et al.54,55 They reported that the phonon dispersion of a single-layer HB sheet did not exhibit any soft phonon modes (i.e., imaginary phonon energies), thus confirming the dynamic stability of the proposed structure. Abtew et al. predicted that charging the structure significantly softens the hydrogen-related phonon modes.55 In contrast, the boron modes were either hardened or not significantly affected by electron doping. Furthermore, the self-doping of the structure (bonding with additional boron atoms) considerably reduced the energy barrier against hydrogen release. These results suggest electrochemical charging or self-doping may facilitate hydrogen release while maintaining the underlying boron network for subsequent rehydrogenation. In 2019, Kawamura et al. reported that ultraviolet (UV) light irradiation induces H2 release from HB sheets,59 which is consistent with this theoretical prediction in terms of electron occupation to the antibonding orbital. However, theoretical calculation did not expect UV light induced H2 release. Moreover, other reported experimental findings including solid-acid catalytic activity,50,51 reductant properties,60–64 and the ability to convert CO2 to C2H6 by C–C coupling,65 have not been predicted theoretically. Here, such unexpected experimental findings are introduced together with other recent theoretical predictions of the excellent properties of HB sheets for several applications.
Light-induced H2 Release from HB Sheets
Figure 6 shows the optical absorption spectrum of the HB sheets.59 The absorption edge exhibits semiconductor characteristics, absorbing light at a wavelength shorter than blue light at 2.8 eV (443 nm). Although the electronic states found by first-principles calculations based on the basic structure shown in Figure 1 are gapless semimetal,56 optically, they have a semiconductor character owing to a selection rule.20,59 In terms of allowed electron transitions, two major transitions were suggested: transition-(1) the transition from the band of σ-bond, consisting of B 2py and H 1s atomic orbitals, to the band of B 2px atomic orbitals (2.4 eV). The optical absorption edge observed in Figure 6a is thought to arise from this transition. Transition-(2): Transition from the band of σ-bond, comprising B 2py and H 1s atomic orbitals, to the band of its antibonding states (3.8 eV). Because transition-(2) is a transition to the antibonding state, H2 release by light irradiation was expected.

(a) Optical absorption spectrum of HB sheets and (b) hydrogen evolution rate under irradiation from different light sources.59
The H2 gas evolution properties of the HB sheets upon light irradiation were then evaluated experimentally using a flow reaction vessel under two different light sources (Figure 6b). Irradiation with visible light causes transition-(1) described above, and irradiation with UV light causes transition-(2) above. The hydrogen molecules evolved only under UV light irradiation. Comparison with first-principles calculations suggests that the electrons transition to the antibonding orbital composed of hydrogen and boron, transition-(2), by irradiation with UV light, leading to cleavage of the hydrogen and boron bonds and evolution of hydrogen molecules.59 By considering the penetration depth of the UV light, the total hydrogen produced was estimated to be 8 wt %, corresponding to 94% of the hydrogen atoms in HB sheets (8.5 wt %). Considering the fact that H atoms have to make a bond prior to becoming H2 molecules in gas phase, as much as 94% hydrogen release from HB sheets by UV irradiation indicates that H atoms (and/or proton) may be able to diffuse on the boron framework of hydrogen deficient HB sheets. Change in the H2 generation rate under UV irradiation in Figure 6b is probably related to such kinetics change (e.g. change in the diffusion rate of H on hydrogen deficient HB sheets as a function of remaining H amount). For the application of the HB sheets as a hydrogen carrier, it is of great interest to explore the photo-induced hydrogen release mechanism as well as hydrogen restorage capability after release of hydrogen as future works.
Solid Acid Catalyst Property of HB Sheets
The solid-acid catalytic property of HB sheets has been experimentally revealed50 together with its catalytic reaction mechanism.51 Figure 7a shows the ethanol conversion rate (conversion rate from ethanol to another molecule) in the presence of HB sheets as measured at various temperatures, where ethanol was introduced as vapor flow. It is clear that the HB sheets have clear ethanol conversion activity. In contrast, MgB2, which is the starting material for HB production, and boron oxide B2O3 do not have any catalytic function. The conversion rate observed with the HB sheets increased with increasing W/F (g min/mmol, the weight of the sample used as catalyst W divided by the ethanol vapor flow rate F). The conversion rate was also found to be constant for a long period of time.50 Note that because of pre-heating at 573 K, hydrogen vacancies inevitably form in the HB sheets, and the HB sheets are in a state with reduced hydrogen content (around H:B = 1:2.5 ± 0.5).50 Gas chromatography detection of the reaction products (excluding water) from catalytic conversion using HB sheets revealed that ethylene is the main product under all measured temperatures (Figure 7b) and W/F conditions.50 These results show that the main catalytic reaction of ethanol reformation by HB sheets is the dehydration reaction, as schematically shown in Figure 7d (C2H5OH → C2H4 + H2O). In general, solid acid catalysts promote ethanol dehydration, whereas base catalysts promote the evolution of acetaldehyde (CH3CHO) via dehydrogenation. Therefore, HB sheets are solid acid catalysts. The activation energy estimated from the Arrhenius plot (Figure 7c) is 102.8 ± 5.5 kJ/mol, which is around the same as the value of well-known solid acid catalysts.50 The analysis of the mechanism using isotope ethanol with Fourier transformed IR showed that the ethanol first adsorbs to the HB (panel I of Figure 7e), and then the hydrogen of the HB and the hydroxyl group of the ethanol react to form water (panel II of Figure 7e), which is released into the gas phase. This is followed by a dehydrogenation reaction, in which the H atom of the methyl group coordinates with the remaining ethyl group and is pulled into the H vacancy created in the HB, and the ethylene that forms are emitted into the gas phase (panel III of Figure 7e). In other words, the lattice H of HB participated in the dehydration reaction of ethanol.51
![HB sheets act as solid acid catalyst. (a) Temperature dependence of C2H5OH conversion rate (W/F = 27.2 g·min/mmol).50 (b) Selectivity of ethanol reforming by HB as a function of temperature at W/F = 6.7 g·min/mmol.50 (c) Arrhenius plot of reaction rate k = (C2H5OH conversion [%])/100 × (C2H5OH flux [mol/s])/HB amount [mol]).50 (d) Schematic of ethanol dehydration on HB sheets.50 (e) Schematic of the reaction mechanism for ethanol dehydration on HB sheets.51](https://oup.silverchair-cdn.com/oup/backfile/Content_public/Journal/chemlett/52/7/10.1246_cl.230210/3/m_cl-230210fig07cmyk.jpeg?Expires=1750361961&Signature=vgS26WTIZIXiHzmP-vqMsx4LDFMDN-XxDQckIk919WKaP1RP5q3Nbxwvn7Zshgz1x29a5~siIbUEER~SSLxq6w-Gm4MH4TLceFJlwsjMGAhBefpH-PzAnmTr7i8p53Pxr4CHgcKoo0RioMR2ULFrjX88na4yGQS9P3uP3d8wBhW9S1PsQrcMKG69kvFTCStiY4hFN-0ZVQMcau33~RnNffw~UgydMvaMO1WqA~g0a9-O4xPgYGrpOjrXlANBQxGQOXL862iYAjzjwOYAuiGtIJTB8BH~YdP8W~uQcVMTHWJxz4hQw0JoJBGiGfnRHoLF1v-Pb313gjEN9jgH3JcO3g__&Key-Pair-Id=APKAIE5G5CRDK6RD3PGA)
HB sheets act as solid acid catalyst. (a) Temperature dependence of C2H5OH conversion rate (W/F = 27.2 g·min/mmol).50 (b) Selectivity of ethanol reforming by HB as a function of temperature at W/F = 6.7 g·min/mmol.50 (c) Arrhenius plot of reaction rate k = (C2H5OH conversion [%])/100 × (C2H5OH flux [mol/s])/HB amount [mol]).50 (d) Schematic of ethanol dehydration on HB sheets.50 (e) Schematic of the reaction mechanism for ethanol dehydration on HB sheets.51
Reductant Property of HB Sheets
When a metal complex and HB sheets were added to acetonitrile, the metal ions were reduced on the HB sheets to form metal nanoparticles. Experimental results using various metal ions and ultraviolet-visible light spectroscopy revealed that HB sheets have a redox potential of −0.277 to −0.257 V vs. SHE.60 Metal ions with a more positive redox potential can be reduced. In contrast, those with a more negative potential cannot be reduced. Furthermore, nanoparticles formed on the HB sheets after reduction, and a nanocomposite of metal nanoparticles supported on the HB sheets could be created by a one-pot reaction, as schematically shown in Figure 8a.60

Nanocomposite of metal nanoparticles and HB sheets. (a) Schematic of the facile nanocomposite formation of HB sheets with metal nanoparticles.60 (b–e). Transmission electron microscopy (TEM) images of Cu–HB nanocomposites.60 (f) Fast Fourier transformed image at the squared region in panel e.60 (g) Nanoparticle size distribution.60 (h) Energy dispersive X-ray spectroscopy was performed only at the sample site in scanning transmission electron microscopy mode.60 (i) TEM image of Ni-HB nanocomposite.64 (j) Histogram of Ni nanoparticles on HB.64
For example, the Cu–HB nanocomposite is shown in the TEM images in Figures 8b–8e.60 The small dark spots observed in the large sheets in Figures 8b and 8c correspond to the formed Cu nanoparticles. Each nanoparticle exhibited crystalline features in the magnified TEM images (Figures 8d and 8e). The fast Fourier transform of the image in the squared region in Figure 8e is shown in Figure 8f, indicating a face-centered cubic structure for the observed nanoclusters, as indicated by the superimposed circles. The particle size was estimated to be 1–6 nm, and the average particle size was 3.8 nm (Figure 8g). When energy dispersive X-ray spectroscopy (EDS) analysis was performed only at the sample site in STEM mode, the nanoparticles were found to be composed solely of Cu.
As another example, the Ni–HB nanocomposite is shown in the TEM image in Figure 8i,64 where Ni nanoparticles with an average diameter of 2 nm (Figure 8j) were spontaneously formed with high-density on HB sheets by simply mixing Ni acetylacetonate and HB sheets in acetonitrile, followed by drying. In the UV-Visible spectra, the absorbance peak position of this composite shifted to higher wavelengths as the Ni acetylacetonate concentration in the mixture increased during formation. This change was then ascribed to the change in the plasmon resonance due to the decrease in the interparticle distance rather than due to the particle size change, based on the TEM observations.64 This indicates that HB sheets are a good support material for dispersing small metal clusters with high density, which is important for various applications, including catalysts, absorbents, and batteries because a large surface area can be realized.
Using this reductant property of HB sheets, Gao et al. reported that noble metal ions, such as PtCl42−, PdCl42−, and AuCl4−, can be reduced without using a surfactant, and then noble metal nanoparticles can be formed on carbon (M/B–C, M = Pt, Pd, and Au) with an ultrafine size (2–3 nm) and high dispersion.61 The amount of noble metal loading reached as high as 52.9 wt %. Owing to their ultrafine size and clean surface, M/B–C catalysts exhibit an activity that surpasses that of their commercial counterparts for the oxygen reduction reaction. Based on this research, Gao et al. further reported that platinum–palladium (PtPd) alloy catalysts with high durability could be viable substituents for commercial Pt/C for proton exchange membrane fuel cells (PEMFCs), where a facile approach for the gram-scale preparation of PtxPd100−x alloy nanoparticles on carbon black was developed using HB sheets.63 The optimized Pt54Pd46/B–C catalyst showed mass activity (MA) and specific activity 3.4 and 1.9 times higher than those of commercial Pt/C. In H2/O2 and H2/air PEMFCs, the membrane electrode assembly (MEA) with Pt54Pd46/B–C achieves peak power densities of 2.33 and 1.04 W cm−2, respectively, and shows negligible performance degradation after 100 h of running in H2/O2 conditions. Moreover, the MA of MEA with Pt54Pd46/B–C in H2/O2 PEMFC reaches 0.978 A mgPt+Pd−1 beyond the 2020 target of the Department of Energy (DOE) of 0.44 A mgPt−1.63
Saad et al. reported that Ag nanoparticles decorated on boron sheets using HB sheets exhibited a greatly enhanced oxygen evolution reaction (OER) performance for Co3O4 catalysts.62 The hydrogen atoms on the surface of HB were interpreted to reduce Ag+ to form Ag nanoparticles with a size of ∼7 nm. The as-formed metallic Ag nanoparticles enhanced the charge-transfer ability of the catalysts. In any case, the as-obtained catalyst (Co3O4-Ag@B) with a crumpled structure displayed an overpotential of 270 mV and a relatively small Tafel slope of 62 mV dec−1, outperforming the Co3O4-Ag@rGO nanosheets and benchmark IrO2 catalysts.62
CO2 Adsorption and Conversion to CH4 and C2H6 on HB Sheets
It is found that while CO2 does not adsorb on pristine HB sheets at 273 K, CO2 reproducibly physisorbed on hydrogen-deficient HB sheets prepared by preheating at 473 K (HxB1, 0.81 < x < 0.98).65 The adsorption of CO2 was found to follow the Langmuir model with a saturation coverage of 2.4 × 10−4 mol g−1 and heat of adsorption of approximately 20 kJ mol−1, which was supported by DFT calculations.65
Notably, at 423 K in a moist atmosphere, CH4 and C2H6 were detected as products of the reaction between CO2 and the HB sheets,65 indicating that hydrogen-deficient HB promotes both C–C coupling and CO2 conversion reactions, as schematically shown in Figure 9. Although CO2 conversion66,67 and C–C coupling68,69 have previously been demonstrated using boron-based molecular systems, this result shows that a solid material composed solely of boron and hydrogen can accomplish both C–C coupling and CO2 conversion.
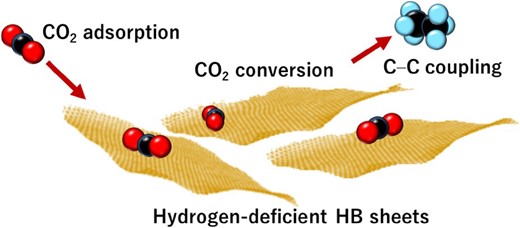
Schematic of CO2 adsorption and conversion to C2H6 via C–C coupling on hydrogen deficient HB sheets.65
Other Theoretically Predicted Intriguing Properties of HB Sheets
Parallel to the experimental findings, theoretical modeling, and computer simulations predicted70 that HB sheets have an intriguing giant thermal conductance,71 and are promising candidates for certain applications such as hydrogen storage materials,54,55,72–74 battery anode materials,75–78 highly selective and sensitive sensors,79,80 current limiters,81 photodetectors,79 and band engineering for electronic devices by applying mechanical loading.82,83
Specifically, HB sheets exhibit a comparable lattice thermal conductance (4.07 nWK−1 nm−2) to graphene (4.1 nWK−1 nm−2) and an electron thermal conductance (3.6 nWK−1 nm−2), which is almost ten times that of graphene. Consequently, the total thermal conductance of 2D hydrogen boride is approximately twice that of graphene and is the highest among all known 2D materials. Moreover, the tensile strain along the armchair direction increased the carrier density, significantly increasing the electron thermal conductance. The increase in the electron thermal conductance offsets the reduction in the phonon thermal conductance, contributing to an abnormal increase in thermal conductance.71
The HB sheet showed an unexpectedly high affinity for Li compared to other alkali and alkaline earth metals (Na, K, Ca, Mg, and Al), with a binding energy of −2.38 eV, which is much larger than its bulk cohesive energy (−1.63 eV). The energy barrier of Li diffusion on an HB sheet was approximately 1.12 eV, showing high dynamic and thermodynamic stability without cluster formation. Moreover, an Li-decorated HB sheet is expected to achieve a high theoretical gravimetric density of 11.57 wt % with an average H2 adsorption energy of −0.17 eV, demonstrating it holds great potential in massive hydrogen storage under ambient conditions.72
As an anode battery, HB sheet was also theoretically reported to lead to a high specific capacity of 861.78 mA h g−1 for Li ions. In contrast, the H vacancies that emerged in the HB sheet electrodes were reported to improve both potassium ion intercalation and potassium ion hopping, yielding a high theoretical capacity (1138 mAh g−1) among the highest reported value in the literature for K ion anode materials.77 Moreover, the HB sheet was reported to present an ultralow out-of-plane bending stiffness, rivaling that of graphene, which gives it more flexibility to accommodate repeated bending, rolling, and folding when applied to wearable devices. It also showed a high in-plane stiffness (157 N/m along the armchair and 109 N/m along the zigzag directions). Thus, the HB sheets can be used as a novel flexible, lightweight potassium ion battery anode material with high capacity, low voltage, and desirable rate performance.75 The mechanical response of the HB sheet was reported to be anisotropic, with an elastic modulus of 131 N m−1 and a high tensile strength of 19.9 N m−1 along the armchair direction. By applying mechanical loading, the metallic electronic character of HB sheets can be altered to direct band gap semiconductor, which is very appealing for applications in nanoelectronics.83
Summary and Perspective
This review summarized the formation and characterization of HB sheets, and experimentally reported several unexpected properties. Specifically, properties such as light-induced H2 release, solid acid catalysis, reduction, and CO2 adsorption and conversion to CH4 and C2H6 were reported in addition to other intriguing theoretically predicted properties. These properties highlight the immense potential of HB sheets for various applications including hydrogen storage and batteries.
Although not introduced here, the experimental formation of HB sheets20 has also paved the way for the conceptual development of new HB materials, including 5-7-ring type HB sheets, HB film, HB nanoribbons, HB nanotubes, and BH/BHB ratio-controlled HB sheets.82,84–94 Recently, hydrogenated borophane with a structure different from that of HB sheets has also been reported as sheets grown on Ag(111)17 and as a free-standing powder.95 These new materials have been further accelerating experimental studies on borophane.18,96–100
Acknowledgment
T. K. thanks Prof. M. Miyauchi, Prof. T. Fujita, Dr. S. Tominaka, Prof. I. Hamada, Prof. S. Okada, Prof. E. Nishibori, Prof. A. Yamamoto, Prof. J. Nakamura, Prof. I. Matsuda, Prof. S. Orimo, Prof. H. Hosono, Dr. N. T. Cuong, Dr. A. Yamaguchi, Dr. S. Iimura, Dr. S. Ito, Dr. T. Fujitani, Prof. J. N. Kondo, Prof. A. Hirata, Dr. N. Umezawa, Mr. H. Nishino, Mr. A. Fujino, Mr. R. Ishibiki, Ms. M. Hikichi, Mr. T. Goto, Mr. T. Fujimori, Mr. R. Kawamura, Ms. C. Shimada, Mr. T. Hirabayashi, Ms. N. Noguchi, Mr. Y. Cho, Dr. K. I. Rojas, Prof. Y. Morikawa, Prof. R. Osuga, Dr. J. M. Oliva-Enrich, Dr. T. Masuda, Dr. I. Tateishi, other members of the Kondo lab, and other co-workers. T. K. appreciates the funds from JSPS KAKENHI (Grant No. JP16H03823, JP16H00898, JP18H03874, JP18K18989, JP19H02551, JP21H05012, JP22K18964, JP23H01843, and Hydrogenomics [JP19H05046:A01 and JP21H00015:B01]), JST PREST (Grant No. JPMJPR1296), JST CREST (Grant No. JPMJCR21O4), MEXT Element Strategy Initiative to Form Core Research Center (JPMXP0112101001), Sanoh Industrial Co., Ltd., and MHI Innovation Accelerator LLC.
References
J. Moulder, W. Stickle, W. Sobol, K. D. Bomben, Handbook of X-Ray Photoelectron Spectroscopy., 1992.
T. Kondo, I. Matsuda, Chemically Modified Borophene. In: Matsuda I., Wu K. (eds) 2D Boron: Boraphene, Borophene, Boronene (https://doi.org/10.1007/978-3-030-49999-0_5), ed. by Iwao Matsuda, Kehui Wu, Springer, Cham., 2021.
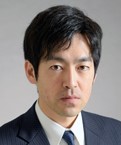
Takahiro Kondo is Professor at Institute of Pure and Applied Sciences and Director of R&D Center for Zero CO2 Emission with Functional Materials, University of Tsukuba from 2022. He also holds Specially-appointed Professor position at the Advanced Institute for Materials Research (AIMR) at Tohoku University. He received Doctor of Philosophy in Engineering from University of Tsukuba at 2003. He spent 4 years at Institute of Physical and Chemical Research (RIKEN) as Postdoctoral researcher. He then moved to University of Tsukuba from 2007. His research focuses on creating new materials, technologies, sciences, and concepts to solve energy, environment and resource problems.