-
PDF
- Split View
-
Views
-
Cite
Cite
Célia Rousseau, Marie Barbiero, Thierry Pozzo, Charalambos Papaxanthis, Olivier White, Actual and Imagined Movements Reveal a Dual Role of the Insular Cortex for Motor Control, Cerebral Cortex, Volume 31, Issue 5, May 2021, Pages 2586–2594, https://doi.org/10.1093/cercor/bhaa376
- Share Icon Share
Abstract
Movements rely on a mixture of feedforward and feedback mechanisms. With experience, the brain builds internal representations of actions in different contexts. Many factors are taken into account in this process among which is the immutable presence of gravity. Any displacement of a massive body in the gravitational field generates forces and torques that must be predicted and compensated by appropriate motor commands. The insular cortex is a key brain area for graviception. However, no attempt has been made to address whether the same internal representation of gravity is shared between feedforward and feedback mechanisms. Here, participants either mentally simulated (only feedforward) or performed (feedforward and feedback) vertical movements of the hand. We found that the posterior part of the insular cortex was engaged when feedback was processed. The anterior insula, however, was activated only in mental simulation of the action. A psychophysical experiment demonstrates participants’ ability to integrate the effects of gravity. Our results point toward a dual internal representation of gravity within the insula. We discuss the conceptual link between these two dualities.
Introduction
Mental simulation is a precious tool for the human mind to recall previous or to anticipate future events. Through mental actions, we can simulate the consequences of our movements on the environment without physically interacting with it. Because motor imagery does not involve movement, the actual motor command is inhibited during mental simulation of movements. This mental process is particularly beneficial when physical movements are not possible, for example, for a patient in a bed rest. It is captivating that mental and actual actions engage similar neural networks, such as the parietal and prefrontal cortices, the supplementary motor area, the premotor and primary motor cortices, the basal ganglia, the cerebellum, and even the spinal cord (Jeannerod 2001; Guillot and Collet 2005; Hétu et al. 2013; Grosprêtre et al. 2016; Hardwick et al. 2018). At the computational level, evidence support the hypothesis that mental simulation of movements is generated by internal forward models, which are neural networks that mimic the causal flow of the physical process by predicting the future sensorimotor state (e.g., position, velocity) given the efferent copy of the motor command and the current state (Miall and Wolpert 1996; Wolpert and Flanagan 2001; Kilteni et al. 2018). This computational perspective assumes that actual and mental movements trigger similar (comparable) motor representations (simulation theory, Jeannerod 2001) and predicted sensory consequences (emulation theory, Grush 2004). Interestingly, motor imagery circumvents any influence of sensory feedback: while mental movement is only a prior of motor planning, actual movement embeds motor planning corrected by sensory feedback. In both cases, sensorimotor information about the initial state is available. Contrasting the execution of movement with motor imagery offers an approach to probe the influence of actual feedback on an action.
Up to now, most motor imagery studies have investigated mental actions by analyzing kinematic variables. One interesting question, therefore, is whether mental simulation also echoes alterations of dynamic parameters that subserve more fundamental properties of a motor action? It is well established that the brain stores internal representations of physical laws to efficiently control movements in altered environment (Wolpert and Ghahramani 2000; Mcintyre et al. 2001; Angelaki et al. 2004; Gaveau et al. 2016; Barbiero et al. 2017). One of the most omnipresent and constant environmental features is the gravitational force. The neural representation of gravity helps optimizing movement execution (Berret et al. 2008; White et al. 2008; Crevecoeur et al. 2009; Gaveau et al. 2016), interacting with falling objects (Zago and Lacquaniti 2005; Zago et al. 2008; Lacquaniti et al. 2013), accurately perceive the body (Angelaki et al. 1999, 2004; Merfeld et al. 1999; Laurens et al. 2013) by solving the ambiguity between gravitational and inertial accelerations (Einstein’s equivalence principle). Previous studies showed that the interaction with visual objects relies on an internal model of gravity stored in the vestibular cortex, assumed to be located in the posterior insula (Indovina et al. 2005; Lacquaniti et al. 2013). This internal representation of gravity was found to be activated by visual motion that appears to be coherent with natural gravity (Indovina et al. 2005; Lacquaniti et al. 2014). Interestingly, the same authors found that mental imagery of objects’ visual motion does not have access to the internal model of Earth gravity but resorts to a simulation of visual motion compatible with a 0-g environment (Gravano et al. 2017). Therefore, it seems that the gravitational acceleration on falling objects influences the behavior of the forward model (that incorporates a representation of gravity) when one performs real actions that involve movements in the environment. A remaining question, however, is whether an internal model of gravity is used when we mentally simulate our own body movements that rely on non-trivial interactions between voluntary limb acceleration and gravitational acceleration.
At the neural level, recent studies have clearly shown that, apart motor-related cortical and subcortical networks, the insula is a crucial brain area involved in the production of arm movements in the gravitational field (Rousseau et al. 2016). This is, however, a nuanced story. Indeed, the posterior insula is activated when the task requires visual gravity cues to plan interceptive actions (Indovina et al. 2005). In contrast, when a task is imagined (that is, only simulated without execution and feedback) or is governed by more abstract rules, the neural activity seems more prominent in the anterior insula (Mutschler et al. 2009). It seems, therefore, important to elucidate the roles of the anterior and posterior insulae when we physically interact with gravity, e.g., during vertical movements, or when we mentally simulate this interaction. Here, participants physically performed or imagined vertical right-hand movements during fMRI sessions. We found that the posterior insula was activated when a movement occurs, but less when forming a mental image of the same task. Furthermore, we also verify in a psychophysical control experiment that both gravity and inertia that characterize the task influence motor imagery processes. Altogether, our studies suggest a dual internal representation of gravity within the insula, but with distinct roles.
Methods
Brain Imaging Experiment
Participants
Twenty-six healthy adults (11 females and 15 males, mean age: 28.3 ± 7.4 years) volunteered for the experiment. All were right-handed, as assessed by the Edinburgh Handedness Inventory (Oldfield 1971), and none of them had history of neurological disorders or any indication against an fMRI examination. No participant had previous sensorimotor experience in hypo- or hyper-gravity. The Movement Imagery Questionnaire-Revised (MIQ-RS, Loison et al. 2013) was used to evaluate participants’ motor imagery ability prior to fMRI. The average score (40 ± 7.8; maximum score = 56) indicated good imagery ability. The entire experiment complied with the Declaration of Helsinki, and informed consents were obtained from all participants. The protocol was approved by the clinical Ethics Committee of the University Hospital of Dijon (registered number 2009-A00646-51).
Data Acquisition
To avoid any circadian bias, we conducted all fMRI experimental sessions during a 2-pm–6-pm interval (Gueugneau et al. 2009; Bonzano et al. 2016). Data were acquired using a 3-T Magnetom Trio system (Siemens AG, Munich, Germany), equipped with a standard head coil configuration. We used standard single shot echo planar (EPI) T2*-weighted sequence in order to measure blood oxygenation level-dependent (BOLD) contrast. The whole brain was covered in 40 adjacent interlaced axial slices (3 mm thickness, TR = 3050 ms, TE = 45 ms, flip angle = 90°), each of which was acquired within a 64 × 64 matrix (FOV was 20 × 20 cm), resulting in a voxel size of 3.125 × 3.125 mm.
Experimental Procedure
We adopted a block design paradigm that alternated periods of rest (10 volumes) and periods of either motor execution (10 volumes) or kinesthetic motor imagery (10 volumes). Each block lasted for 20 s and allowed participants to naturally complete about 10 movement cycles. In the MRI scanner, the upper right limb was maintained slightly elevated by small cushions. The hand was in supine position (palm up) with fingers released (Fig. 1A). In the rest condition, participants were instructed to remain quiet, motionless, and to keep their eyes open without thinking of anything in particular. In the executed condition, participants performed hand flexion-extension in the sagittal plane at free pace. In the imagined condition, they internally simulated the same vertical hand movements (Fig. 1B) without actually performing them and by adopting the same hand configuration as during the rest condition. Specifically, participants had to feel themselves performing hand flexions and extensions in a first-person perspective (as in Demougeot and Papaxanthis 2011). After the imagined session, participants reported the quality of imagined movements on a 7-point scale (1 corresponding to “Very difficult to feel/reproduce the motor task” and 7 “Very easy to feel/reproduce the motor task”). The average score (5 ± 1.1) indicated that all participants were actively engaged in the motor imagery process without experiencing any difficulty. Participants executed or imagined the motor task between a “GO” and a “STOP” signal delivered by the experimenter through headphones. We repeated the rest and executed conditions or the rest and imagined conditions four times during one recording session. Therefore, 80 volumes in each experimental condition were recorded per participant (4 × 10 volumes in the rest condition and 4 × 10 volumes of either the executed or the imagined condition). Half of the participants started with the executed condition and half with the imagined condition.
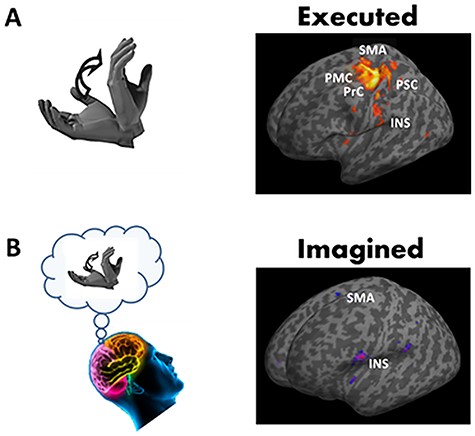
Brain areas activated during motor execution (A) and during motor imagery of hand movements (B) compared to rest. Brain responses are projected onto the inflated T1 template of MNI. Most significant brain responses are highlighted in the primary motor cortex (PMC) and the primary somatosensory cortices (PSC), in the premotor cortex (PrC) and the supplementary motor area (SMA), and the insular cortex (INS).
Data Preprocessing and Analysis
Data analysis was performed using SPM 12 (www.fil.ion.ucl.ac.uk/spm). For functional data pre-processing, each volume of both sessions was spatially realigned with the first volume of the first session using a 6-parameter fixed body transformation. Then, the T1-weighted anatomical volume was co-registered to mean images created by the realignment procedure and was normalized to the Montreal Neurological Institute (MNI) space resampled to 2-mm isotropic voxel size. The anatomical normalization parameters were subsequently used for the normalization of functional volumes. Finally, the normalized functional images were spatially smoothed with 8 × 8 × 8 mm3 full-width at half-maximum isotropic Gaussian kernel. Time series at each voxel for each participant were high-pass filtered at 128 s to remove low-frequency drifts in signal and pre-whitened by means of an autoregressive model AR(1). Data were subsequently analyzed by applying a general linear model (GLM) separately for each participant. Blocks of executed, imagined, and rest conditions were modeled using a box-car function convolved with the hemodynamic response function. Movement parameters derived from realignment corrections were also used as regressors of no interest.
At the individual level, we first assessed the whole network of brain areas involved in the processing of executed and imagined hand movements by contrasting the active phases with the rest blocks. Then, we performed a group analysis and applied one sample t-tests for the basic contrasts executed>rest and imagined>rest. A particular emphasis was given to insular cortex activation. We specifically tested insular cortex activation by contrasting executed and imagined conditions (executed-rest > imagined-rest and imagined-rest > executed-rest). We used an anatomical mask of the bilateral insula when performing group analysis and applied one sample t-tests for each of the two contrasts. For the whole brain network analysis, as well as for the insular cortex analysis, clusters of activated voxels were identified based on the intensity of the individual response for all contrasts (P < 0.05, corrected for multiple comparisons with Bonferroni correction, t > 6.3). An extended threshold of 10 voxels was determined empirically and then used for all contrasts. Results of brain activations were characterized in terms of their peak height and spatial extent and were presented in normalized stereotactic space (MNI). Brain responses were identified by means of the anatomic automatic labeling (Tzourio-Mazoyer et al. 2002).
Psychophysical Experiment
The aim of this experiment was to examine whether dynamic constraints, such as the effects of gravity on movements, are integrated into the processes that subserve motor imagery. A very simple and natural movement that is influenced by gravity and by the biomechanics of the system is a pendular arm movement. Previous studies showed that participants tend to adopt a pace that is faster (respectively slower) when gravity increases (respectively decreases) (Mechtcheriakov et al. 2002; White et al. 2008) or when inertia increases (Hatsopoulos and Warren 1996). This strong effect holds even if participants are instructed to maintain a constant pace.
Participants
Fifteen right-handed healthy adults (6 females and 9 males, mean age: 25.3 ± 7.8 years), who did not participate in the fMRI experiment, volunteered for the psychophysical experiment. No participant had history of neurological disorders or neuromuscular or chronic disease. The Movement Imagery Questionnaire-Revised (MIQ-RS, Loison et al. 2013) was used to evaluate participants’ motor imagery ability prior to taking part to the psychophysical experiment. The average score (36.7 ± 8.8; maximum score = 56) indicated good kinesthesis imagery ability. The protocol was approved by the clinical Ethics Committee of the University Hospital of Dijon (registered number 2009-A00646-51) and complied with the Declaration of Helsinki. Written informed consent was obtained from all participants. All of them were naïve as to the purpose of the experiment and were debriefed after the experimental session.
Experimental Procedure
Participants were comfortably seated in an armless chair and positioned the dominant arm vertically outstretched. In the light loading condition, participants held an empty plastic bottle (0.039 kg). In the heavy loading condition, participants held the same bottle, but full of water (1.089 kg), therefore increasing the inertia of the arm-bottle system. We positioned markers on the bottle, as well as on the shoulder, elbow, forearm, and wrist joints and recorded their 3D position (200 Hz, dual-pass autoregressive filter at 20 Hz) with a Vicon motion tracking system. Participants were asked to perform rhythmic movements at a free comfortable and natural pace for 30 s with the outstretched arm, holding either the empty bottle or the full bottle. Each bottle was used five times, and trials were randomly interleaved. We then extracted the period of oscillations with a fast Fourier transform.
We repeated the same experiment in motor imagery. Participants adopted the same starting posture, but were now asked to mentally simulate 15 continuous cycles of movement in each loading condition (four with the empty bottle and four with the full bottle, randomized). The participants were instructed to keep their arm fixed along the body. As previously, they did not have an imposed pace to follow. However, they had to imagine performing constant swing amplitudes throughout the experimental session. The same experimenter triggered a stopwatch when the participant said “GO” and stopped it at his/her “STOP” injunction.
Finally, we pushed the exercise one step further and asked the participants to mentally simulate the same pendular movements, but in two unnatural environments. In the first, they had to imagine as if they were in weightlessness (0 g condition). In the second, they had to project themselves in hyper-gravity (2 g condition), as if they weighted twice as much. Participants watched short movie clips of unrelated movements (jumps) performed in weightlessness and hyper-gravity contexts (recorded during parabolic flights). As before, there were four trials of 15 cycles in each gravitational condition randomly presented. The posture adopted was the same as in the imagined light condition.
Data Analysis
Normal distribution of the variables was verified before using parametric statistical tests (Shapiro–Wilk W test; P > 0.05). We used a 2-way RM ANOVA to analyze the effects of modality (2 levels; executed vs. imagined) and loading (2 levels; light vs. heavy) on period. We also used paired two-tailed t-tests to compare periods between 0 g and 2 g in the imagined modality. We calculated linear correlation between the periods predicted by the model and the measured periods, separately in each modality, by taking into account the different anthropometric characteristics of participants. Data processing and statistical analyses were done using Matlab (The Mathworks, Chicago, IL).
Results
Our main objective is to refine the roles of the anterior and posterior insulae with respect to the processing of gravity in the control of actions. We compared neuronal activations between executed and imagined hand movements in an MRI scanner. While both modalities require a cognitive step for movement preparation, only executed movements process feedback signals. We also developed a simple biomechanical model to demonstrate that even during motor imagery, biomechanical factors are integrated into mental states and thus influence the spontaneous timing of the mental action.
Actual and Mental Movements Activate Different Parts of the Insula
Participants performed actual and mental movements of hand flexion-extension in the sagittal plane at free pace. Table 1 summarizes the brain network involved in the execution (executed > rest) and the mental simulation (imagined > rest) of hand movements. In actual movement production, the largest clusters were identified in the left primary motor and somatosensory cortices, in the bilateral supplementary motor area (SMA), as well as in the right cerebellum (lobes IV, V, VI). Activations were also highlighted in the left thalamus, in the left premotor cortex, in the left cerebellum (VI), and in the left middle temporal gyrus. For the imagined hand movements, the largest clusters were recorded in the right cerebellum (lobes VI) and the SMA bilaterally. The right inferior frontal gyrus, the left putamen, the right inferior frontal operculum, and the left inferior parietal lobule were also activated. Figure 1 depicts the main brain areas activated during actual movement production and its mental simulation.
Significant activations to the contrasts [executed>rest] and [imagined>rest] (P-corrected for multiple comparisons<0.05). Brain lobe, regions from AAL atlas and coordinates (x, y, z) in the MNI space are reported. The first region has the highest number of voxels in the cluster, and the other regions [between brackets] belong to the cluster with lower number of voxels. The two last columns correspond respectively to the maximum T-value and the number of voxels in the cluster
Lobes . | X . | Y . | Z . | T-value . | Voxels in . |
---|---|---|---|---|---|
AAL regions executed>rest | |||||
Parietal, frontal, sublobar and limbic lobe, left postcentral gyrus, [left precentral gyrus, left superior motor area, left midcingulate area, left supramarginal, left Rolandic operculum, left inferior parietal lobule, right superior motor area] | −30 | −26 | 56 | 17.57 | 3260 |
Anterior lobe of cerebellum, right cerebellum (lobules IV, V, VI), [Vermis IV, V, VI] | 22 | −50 | −22 | 15.80 | 1738 |
Parietal lobe, left thalamus | −14 | −22 | 6 | 9.99 | 75 |
Sub lobar & frontal lobe, left insula, [left Rolandic operculum] | −44 | 0 | 2 | 9.32 | 208 |
Parietal lobe, right supramarginal | 56 | −32 | 24 | 9.22 | 233 |
Frontal lobe, left precentral gyrus | −56 | 4 | 32 | 7.96 | 30 |
Anterior lobe of cerebellum, left cerebellum (lobules VI) | −30 | −54 | −26 | 7.88 | 57 |
Sub lobar, frontal and temporal lobe, right Rolandic operculum [right inferior frontal operculum], [right insula] | 46 | 2 | 6 | 7.82 | 103 |
Temporal lobe, left middle temporal gyrus | −52 | −70 | 6 | 7.78 | 20 |
Anterior lobe of cerebellum, Vermis IV, V | 2 | −44 | −2 | 7.59 | 17 |
Lobes | X | Y | Z | T-value | Voxels in |
AAL regions imagined>rest | |||||
Anterior lobe of cerebellum, right cerebellum (lobules VI) | 34 | −54 | −28 | 10.79 | 138 |
Sub lobar, frontal and temporal lobe, left Rolandic operculum [left inferior frontal operculum], [left insula] | −54 | 6 | 10 | 10.15 | 356 |
Frontal lobe, left superior motor area, [right superior motor area] | −6 | −8 | 66 | 9.04 | 125 |
Parietal lobe, left supramarginal | −48 | −36 | 22 | 8.47 | 88 |
Frontal lobe, right inferior frontal gyrus | 46 | 42 | −6 | 8.28 | 49 |
Sublobar, left putamen | −26 | −4 | 12 | 8.24 | 27 |
Frontal lobe, right inferior frontal operculum | 56 | 8 | 20 | 7.77 | 21 |
Parietal lobe, right supramarginal | 60 | −32 | 26 | 7.69 | 44 |
Parietal lobe, left inferior parietal lobule | −50 | 46 | 40 | 7.59 | 12 |
Sub lobar, frontal and temporal lobe, right Rolandic operculum | 50 | 6 | 6 | 7.23 | 27 |
Frontal lobe, right inferior frontal operculum | 54 | 16 | 14 | 6.80 | 10 |
Lobes . | X . | Y . | Z . | T-value . | Voxels in . |
---|---|---|---|---|---|
AAL regions executed>rest | |||||
Parietal, frontal, sublobar and limbic lobe, left postcentral gyrus, [left precentral gyrus, left superior motor area, left midcingulate area, left supramarginal, left Rolandic operculum, left inferior parietal lobule, right superior motor area] | −30 | −26 | 56 | 17.57 | 3260 |
Anterior lobe of cerebellum, right cerebellum (lobules IV, V, VI), [Vermis IV, V, VI] | 22 | −50 | −22 | 15.80 | 1738 |
Parietal lobe, left thalamus | −14 | −22 | 6 | 9.99 | 75 |
Sub lobar & frontal lobe, left insula, [left Rolandic operculum] | −44 | 0 | 2 | 9.32 | 208 |
Parietal lobe, right supramarginal | 56 | −32 | 24 | 9.22 | 233 |
Frontal lobe, left precentral gyrus | −56 | 4 | 32 | 7.96 | 30 |
Anterior lobe of cerebellum, left cerebellum (lobules VI) | −30 | −54 | −26 | 7.88 | 57 |
Sub lobar, frontal and temporal lobe, right Rolandic operculum [right inferior frontal operculum], [right insula] | 46 | 2 | 6 | 7.82 | 103 |
Temporal lobe, left middle temporal gyrus | −52 | −70 | 6 | 7.78 | 20 |
Anterior lobe of cerebellum, Vermis IV, V | 2 | −44 | −2 | 7.59 | 17 |
Lobes | X | Y | Z | T-value | Voxels in |
AAL regions imagined>rest | |||||
Anterior lobe of cerebellum, right cerebellum (lobules VI) | 34 | −54 | −28 | 10.79 | 138 |
Sub lobar, frontal and temporal lobe, left Rolandic operculum [left inferior frontal operculum], [left insula] | −54 | 6 | 10 | 10.15 | 356 |
Frontal lobe, left superior motor area, [right superior motor area] | −6 | −8 | 66 | 9.04 | 125 |
Parietal lobe, left supramarginal | −48 | −36 | 22 | 8.47 | 88 |
Frontal lobe, right inferior frontal gyrus | 46 | 42 | −6 | 8.28 | 49 |
Sublobar, left putamen | −26 | −4 | 12 | 8.24 | 27 |
Frontal lobe, right inferior frontal operculum | 56 | 8 | 20 | 7.77 | 21 |
Parietal lobe, right supramarginal | 60 | −32 | 26 | 7.69 | 44 |
Parietal lobe, left inferior parietal lobule | −50 | 46 | 40 | 7.59 | 12 |
Sub lobar, frontal and temporal lobe, right Rolandic operculum | 50 | 6 | 6 | 7.23 | 27 |
Frontal lobe, right inferior frontal operculum | 54 | 16 | 14 | 6.80 | 10 |
Significant activations to the contrasts [executed>rest] and [imagined>rest] (P-corrected for multiple comparisons<0.05). Brain lobe, regions from AAL atlas and coordinates (x, y, z) in the MNI space are reported. The first region has the highest number of voxels in the cluster, and the other regions [between brackets] belong to the cluster with lower number of voxels. The two last columns correspond respectively to the maximum T-value and the number of voxels in the cluster
Lobes . | X . | Y . | Z . | T-value . | Voxels in . |
---|---|---|---|---|---|
AAL regions executed>rest | |||||
Parietal, frontal, sublobar and limbic lobe, left postcentral gyrus, [left precentral gyrus, left superior motor area, left midcingulate area, left supramarginal, left Rolandic operculum, left inferior parietal lobule, right superior motor area] | −30 | −26 | 56 | 17.57 | 3260 |
Anterior lobe of cerebellum, right cerebellum (lobules IV, V, VI), [Vermis IV, V, VI] | 22 | −50 | −22 | 15.80 | 1738 |
Parietal lobe, left thalamus | −14 | −22 | 6 | 9.99 | 75 |
Sub lobar & frontal lobe, left insula, [left Rolandic operculum] | −44 | 0 | 2 | 9.32 | 208 |
Parietal lobe, right supramarginal | 56 | −32 | 24 | 9.22 | 233 |
Frontal lobe, left precentral gyrus | −56 | 4 | 32 | 7.96 | 30 |
Anterior lobe of cerebellum, left cerebellum (lobules VI) | −30 | −54 | −26 | 7.88 | 57 |
Sub lobar, frontal and temporal lobe, right Rolandic operculum [right inferior frontal operculum], [right insula] | 46 | 2 | 6 | 7.82 | 103 |
Temporal lobe, left middle temporal gyrus | −52 | −70 | 6 | 7.78 | 20 |
Anterior lobe of cerebellum, Vermis IV, V | 2 | −44 | −2 | 7.59 | 17 |
Lobes | X | Y | Z | T-value | Voxels in |
AAL regions imagined>rest | |||||
Anterior lobe of cerebellum, right cerebellum (lobules VI) | 34 | −54 | −28 | 10.79 | 138 |
Sub lobar, frontal and temporal lobe, left Rolandic operculum [left inferior frontal operculum], [left insula] | −54 | 6 | 10 | 10.15 | 356 |
Frontal lobe, left superior motor area, [right superior motor area] | −6 | −8 | 66 | 9.04 | 125 |
Parietal lobe, left supramarginal | −48 | −36 | 22 | 8.47 | 88 |
Frontal lobe, right inferior frontal gyrus | 46 | 42 | −6 | 8.28 | 49 |
Sublobar, left putamen | −26 | −4 | 12 | 8.24 | 27 |
Frontal lobe, right inferior frontal operculum | 56 | 8 | 20 | 7.77 | 21 |
Parietal lobe, right supramarginal | 60 | −32 | 26 | 7.69 | 44 |
Parietal lobe, left inferior parietal lobule | −50 | 46 | 40 | 7.59 | 12 |
Sub lobar, frontal and temporal lobe, right Rolandic operculum | 50 | 6 | 6 | 7.23 | 27 |
Frontal lobe, right inferior frontal operculum | 54 | 16 | 14 | 6.80 | 10 |
Lobes . | X . | Y . | Z . | T-value . | Voxels in . |
---|---|---|---|---|---|
AAL regions executed>rest | |||||
Parietal, frontal, sublobar and limbic lobe, left postcentral gyrus, [left precentral gyrus, left superior motor area, left midcingulate area, left supramarginal, left Rolandic operculum, left inferior parietal lobule, right superior motor area] | −30 | −26 | 56 | 17.57 | 3260 |
Anterior lobe of cerebellum, right cerebellum (lobules IV, V, VI), [Vermis IV, V, VI] | 22 | −50 | −22 | 15.80 | 1738 |
Parietal lobe, left thalamus | −14 | −22 | 6 | 9.99 | 75 |
Sub lobar & frontal lobe, left insula, [left Rolandic operculum] | −44 | 0 | 2 | 9.32 | 208 |
Parietal lobe, right supramarginal | 56 | −32 | 24 | 9.22 | 233 |
Frontal lobe, left precentral gyrus | −56 | 4 | 32 | 7.96 | 30 |
Anterior lobe of cerebellum, left cerebellum (lobules VI) | −30 | −54 | −26 | 7.88 | 57 |
Sub lobar, frontal and temporal lobe, right Rolandic operculum [right inferior frontal operculum], [right insula] | 46 | 2 | 6 | 7.82 | 103 |
Temporal lobe, left middle temporal gyrus | −52 | −70 | 6 | 7.78 | 20 |
Anterior lobe of cerebellum, Vermis IV, V | 2 | −44 | −2 | 7.59 | 17 |
Lobes | X | Y | Z | T-value | Voxels in |
AAL regions imagined>rest | |||||
Anterior lobe of cerebellum, right cerebellum (lobules VI) | 34 | −54 | −28 | 10.79 | 138 |
Sub lobar, frontal and temporal lobe, left Rolandic operculum [left inferior frontal operculum], [left insula] | −54 | 6 | 10 | 10.15 | 356 |
Frontal lobe, left superior motor area, [right superior motor area] | −6 | −8 | 66 | 9.04 | 125 |
Parietal lobe, left supramarginal | −48 | −36 | 22 | 8.47 | 88 |
Frontal lobe, right inferior frontal gyrus | 46 | 42 | −6 | 8.28 | 49 |
Sublobar, left putamen | −26 | −4 | 12 | 8.24 | 27 |
Frontal lobe, right inferior frontal operculum | 56 | 8 | 20 | 7.77 | 21 |
Parietal lobe, right supramarginal | 60 | −32 | 26 | 7.69 | 44 |
Parietal lobe, left inferior parietal lobule | −50 | 46 | 40 | 7.59 | 12 |
Sub lobar, frontal and temporal lobe, right Rolandic operculum | 50 | 6 | 6 | 7.23 | 27 |
Frontal lobe, right inferior frontal operculum | 54 | 16 | 14 | 6.80 | 10 |
The main objective of this report was to investigate whether the insula, known to be involved in the processing of gravity-relevant signals during executed movements, was also activated during imagined hand movements. We therefore focused our analysis on that brain region. The contrasts executed>rest and imagined>rest revealed the activation of both the right and left insulae. The analysis did not highlight clear differences in insular responses between the two contrasts, likely due to the size of the clusters covering the insula. Noteworthily, the peak activity was localized in a more anterior part of the insular cortex in imagined movements (compare Fig. 1A,B). To further compare the activation of the insular cortex between executed and imagined movements, we examined the contrasts executed-rest>imagined-rest and imagined-rest>executed-rest by using an anatomical mask of the bilateral insula (P < 0.05, corrected for multiple comparisons). Interestingly, we identified one cluster in the posterior part of the left insular cortex (x = −38, y = −26, z = 24) when contrasting executed with imagined movements (Fig. 2), but not when we contrasted imagined with executed hand movements.
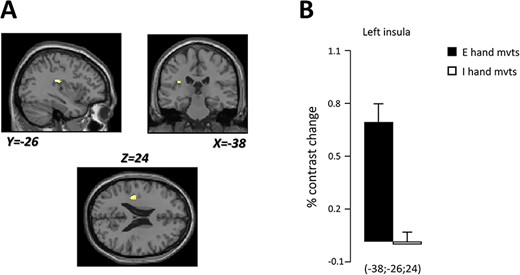
(A) Localization of the cluster found in the left insular cortex (white spot) specifically engaged during executed compared to imagined hand movements. Brain responses are projected onto the sagittal (Y = −26), coronal (X = −38), and axial (Z = 24) views of the T1-single subject template of MNI. (B) Percent contrast change in the left insular cortex during executed (black) and imagined (white) vertical hand movements.
Imagined Movements Integrate Gravito-Inertial Constraints
The aim of the psychophysical experiment was to verify that gravito-inertial constraints are taken into account during motor imagery. Participants imagined and performed rhythmic pendular movements in two inertial conditions in the lab environment and imagined the same movements as if they were in microgravity (0 g) or hyper-gravity (2 g).
Figure 3 (left panel) depicts the average periods spontaneously adopted by participants (in the normal gravity environment of the lab) in the light (empty bottle) and heavy (filled bottle) loading conditions and separately for the real (gray bars) and imagined movement conditions (hatched bars).

Mean periods of movements. (Left panel) Spontaneous periods of movements in the light loading condition (open bottle) and in the heavy loading condition (closed bottle). Gray bars and hatched bars denote real and imagined movement conditions, respectively. (Right panel) Periods measured during imagined movements performed in weightlessness (0 g) or in hypergravity (2 g). Error bars are SD.
The ANOVA revealed a main effect of loading condition (F1,14 = 14.62, P = 0.002, η2 = 0.51), but no effect of movement condition (F1,14 = 0.02, P = 0.90) or interaction effect (F1,14 = 0.01, P = 0.74). Consistently, participants moved at a slower pace (i.e., spontaneously adopted longer periods) in the executed and imagined heavy movement conditions compared to the light loading condition. A correlation analysis within each loading condition and between both movement modalities confirmed these results. The correlation coefficient was 0.66 (P < 0.001) between the executed and imagined light condition and 0.75 (P < 0001) between the executed and imagined heavy condition. The model also predicts small changes with body segment lengths. To quantify this, we calculated the correlation between model predictions of the spontaneous period (by specifying the exact lengths of the upper arm, lower arm, and wrist) and the measured period. We found significant correlations in the executed modality (r = 0.54, P < 0.001) and imagined modality (r = 0.42, P = 0.038).
The average value of |$\alpha$| is 0.61 (SEM = 0.12), which is below 1 but clearly not zero. This is not surprising since the model is ill-defined for g = 0 (it predicts infinite periods). It was shown previously that other neural mechanisms take over when g approaches zero (White et al. 2008).
Together, this psychophysical experiment shows that participants do not only integrate biomechanical factors to simulate a movement including their own morphology, but also fundamental environmental factors such as gravity. This underlines a good capacity of kinesthetic mental imagery and puts us on safe grounds to assume that gravity is indeed encoded in mental simulation.
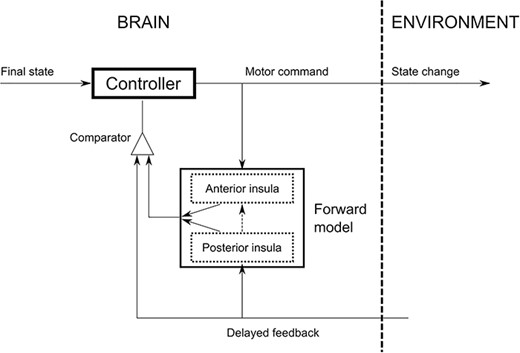
Conceptual sketch illustrating the different roles of the posterior and anterior insula in internal models, with respect to the specific processing of gravity.
Discussion
Our aim was to disentangle the roles of the anterior and posterior insulae in the way they process gravity by contrasting actual and mental movements. We found that mental movements activated brain areas similar to actual movements. This confirms that motor execution and motor imagery engage common neural representations (for a review, see Hétu et al. 2013; Hardwick et al. 2018). Our findings also revealed brain responses in the insular cortex in both actual and imagined movements. More specifically, we found posterior activation of the insula in movement execution and anterior activation of the insula in mental movement simulation. The gravitational force significantly alters motion dynamics during the execution of movements. The insula, known to process the effects of gravity via a stored internal representation of gravitational acceleration, is activated for actual hand movements (Indovina et al. 2005; Lacquaniti et al. 2014; Rousseau et al. 2016). Here, we made a step forward by showing that the insula is also activated when the task mainly relies on mental feedforward states.
Visual tasks have been shown to activate the insula. In an elegant study, Lacquaniti and colleagues demonstrated that an internal representation of gravity is activated by visual motion that appears to be coherent with natural gravity (Indovina et al. 2005; Lacquaniti et al. 2014). The insular activation associated to neural feedforward mechanisms was also observed during experiment of visual motion when comparing prediction versus perception of visual motion (Cheong et al. 2012). Here, we go one step beyond these previous investigations by showing that the insular cortex is activated during mental movement simulation in absence of gravity-relevant visual feedback. In line with our results, global body movements, such as active balance simulation task (Karim et al. 2014) and mental imagery of balance (Malouin et al. 2003; Jahn et al. 2004) elicited fMRI responses in the insula. Further, Sacco and colleagues showed that the insula plays an important role in mental imagery of tactile and proprioceptive sensations and therefore in the imagery of actions and sensations in general (Sacco et al. 2006; Dijkerman and de Haan 2007). Our results demonstrate that the insular cortex integrates dynamical constraints—including gravity—to implement feedforward mechanisms, even in absence of physical interaction between the body and the environment.
We, however, found different activations within the insula across experimental conditions. The posterior insula was more activated during actual hand movements compared to imagined hand movements, which, to the best of our knowledge, is an original finding. The largest number of afferences with the sensorimotor system in the posterior insula may explain why its activity during real movements was higher compared to the anterior insula. At this stage of investigation, we can speculate that the anterior and posterior insulae entail different roles. On the one hand, the anterior insula may implement feedforward mechanisms (internal simulations) to integrate the expected dynamical constraints caused by gravity when no gravity-relevant feedback is available. On the other hand, the posterior insula could be fundamental to process the effects of gravity by including the information conveyed by sensory feedback. More precisely, our data suggest there may be two complementary representations of gravity in the insular cortex, conceptually illustrated in Fig. 4. The first, situated in the posterior insula (dotted bottom box), is used when sensory feedback is available. In that case, feedback signals directly update the internal representation of gravity (vertical up arrow). The output of the forward model (slanted up arrow) is compared with the feedback to evaluate the presence of errors. The second, offline, representation of gravity is held in the anterior part of the insula (dotted top box) and can be considered as a proxy of the first. Since no task-relevant feedback is available, we posit this dual internal model of gravity cannot be adapted during motor imagery, but is accessible for simulations. In this case, the posterior insula is not included in the online control loop. During motor imagery, the output of the anterior insula (slanted down arrow) is compared with feedback. These two regions are unidirectionally connected in such a way to allow the posterior insula to update the abstract representation of gravity in the anterior insula (vertical black dashed line). The question remains open as to why two different versions exist.
We also examined the temporal features of actual and mental movements performed under different gravito-inertial constraints. Our results showed, regardless of task dynamics, a high temporal correspondence between them. This isochronism extends previous findings that reported comparable durations of actual and mental movements (Gentili et al. 2004; Guillot and Collet 2005; Papaxanthis et al. 2012) and reinforces the well-documented idea of a similar neurocognitive network between actual and mental states (Ruffino et al. 2017; Grosprêtre et al. 2019). Temporal similarities between actual and mental movements reinforce the idea that the brain preserves an accurate internal model of arm and environmental dynamics. A controller and a forward internal model, integrating task dynamics, could provide similar durations for actual and mental movements. The controller would generate the appropriate neural commands necessary to move the segment into the vertical plane. The forward model would relate the sensory signals of the actual state of the segment (e.g., position, velocity) to the motor commands and would predict its future states. In the case of imagined movements, the motor commands do not reach muscles, i.e., no movement occurs. However, a copy of these motor commands is still available to the forward model, which predicts the future states of the segment and therefore provides temporal information very similar to that of actual movements. In general, our results indicate that the CNS maintains an accurate representation of gravito-inertial dynamics and uses this representation to appropriately simulate arm movements in the vertical plane.
Strikingly, albeit participants were in a normal gravity environment, they could mentally simulate arm movements in radically new contexts (i.e., microgravity or hyper-gravity) by appropriately changing their temporal features (see modeling results). Specifically, movement imagined in microgravity and hyper-gravity were, correspondingly, slower and faster than movements imagined in normal gravity. This further suggests that the brain can imagine movements in a purely cognitive process (none of the participants had a personal sensorimotor experience of these altered contexts) in other environments than the habitual Earth’s environment. Should the brain had imagined movements irrespective of gravitational constraints, similar durations would have been observed between the different conditions. Note that the integration of gravity into motor prediction per se is not a de facto condition. Indeed, it has been proposed that mental imagery of objects’ visual motion does not have access to the internal model of Earth gravity but resorts to a simulation of visual motion compatible with a 0-g environment (Gravano et al. 2017). It is possible that when trying to extrapolate object trajectories in the environment, the internal model of gravity for these tasks is triggered by visual information of the object motion. In contrast, during internally generated feedforward movements (mental imagery), the brain could rely on a more physical (in the Newtonian sense) internal model of gravity (Mcintyre et al. 2001). The question of whether the body is actively involved in the task may therefore be decisive as to which gravitational representation to load. It remains to be tested, however, which part of the insula and therefore which modality of the gravitational model is activated when purely imagining an object moving in the gravitational field as opposed to motor imagery.
Notes
The authors thank J. Gaveau for interesting discussions to refine the psychophysics experiment. The authors also warmly thank the University Hospital and its kind personnel to assist the first author (CR) during the fMRI sessions. Conflict of Interest: The authors declare no conflict of interest.
Funding
Conseil Général de Bourgogne-Franche Comté (France); Fonds Européen de Développement Régional (FEDER); Centre National d’Etudes Spatiales (CNES); French “INVESTISSEMENTS D’AVENIR” program, project ISITE-BFC (contract ANR-15-IDEX-0003); ANR (Motion, ANR-14-CE30-0007).
References
Author notes
Célia Rousseau and Marie Barbiero Equal contribution
Charalambos Papaxanthis1 and Olivier White Equal contribution