-
PDF
- Split View
-
Views
-
Cite
Cite
Vanessa Siffredi, Maria G Preti, Valeria Kebets, Silvia Obertino, Richard J Leventer, Alissandra McIlroy, Amanda G Wood, Vicki Anderson, Megan M Spencer-Smith, Dimitri Van De Ville, Structural Neuroplastic Responses Preserve Functional Connectivity and Neurobehavioural Outcomes in Children Born Without Corpus Callosum, Cerebral Cortex, Volume 31, Issue 2, February 2021, Pages 1227–1239, https://doi.org/10.1093/cercor/bhaa289
- Share Icon Share
Abstract
The corpus callosum is the largest white matter pathway in the brain connecting the two hemispheres. In the context of developmental absence (agenesis) of the corpus callosum (AgCC), a proposed candidate for neuroplastic response is strengthening of intrahemispheric pathways. To test this hypothesis, we assessed structural and functional connectivity in a uniquely large cohort of children with AgCC (n = 20) compared with typically developing controls (TDC, n = 29), and then examined associations with neurobehavioral outcomes using a multivariate data-driven approach (partial least squares correlation, PLSC). For structural connectivity, children with AgCC showed a significant increase in intrahemispheric connectivity in addition to a significant decrease in interhemispheric connectivity compared with TDC, in line with the aforementioned hypothesis. In contrast, for functional connectivity, children with AgCC and TDC showed a similar pattern of intrahemispheric and interhemispheric connectivity. In conclusion, we observed structural strengthening of intrahemispheric pathways in children born without corpus callosum, which seems to allow for functional connectivity comparable to a typically developing brain, and were relevant to explain neurobehavioral outcomes in this population. This neuroplasticity might be relevant to other disorders of axonal guidance, and developmental disorders in which corpus callosum alteration is observed.
Introduction
Neuroplasticity is the intrinsic property of the central nervous system to respond dynamically to the environment via modification of neural circuitry. In the context of a brain lesion, plasticity provides a mechanism for the brain to adjust through remyelination, reorganization of circuits, and/or neural and behavioral compensation (Anderson et al. 2011a; Dennis et al. 2013). In particular, clinicopathological observations suggest that the immature human brain is capable of major structural and functional reorganization (Kennard 1938). As an example of early disruption of programmed developmental brain processes, children and adults born without a corpus callosum show remarkable brain plasticity. Indeed, there is very little evidence of the interhemispheric disconnection compared to adults who have undergone surgical disconnection of the corpus callosum (i.e., split brain patients), which affects motor control, spatial orientation, vision, hearing, and language (Lassonde and Jeeves 1994; Mancuso et al. 2019).
With more than 190 million topographically organized axons, the corpus callosum is the major commissural fiber bundle in the human brain connecting homologous structures in both hemispheres (Edwards et al. 2014). This structure develops prenatally with the first fibers crossing the midline through the massa commissuralis as early as 11–12 weeks of gestation (Richards et al. 2004; Schell-Apacik et al. 2008) and reaches its final shape by 20 gestational weeks. In typical brain development, these callosal connections participate in a myriad of lower and higher level cognitive functions, and in the integration of complex information transfer between the cerebral hemispheres (Schulte and Müller-Oehring 2010). Given the structural and functional importance of the corpus callosum and its very early maturation, its developmental absence, called agenesis of the corpus callosum (AgCC), provides a unique window to better understand neuroplastic responses.
In the context of AgCC, different neuroplastic responses, for example, enlarged (hyperplasia) of anterior and posterior commissures, may contribute to neurobehavioral functioning (Brown et al. 1999; Siffredi et al. 2019). A recent study found associations between structural and microstructural properties of these commissures and attentional capacity (Siffredi et al. 2019). Moreover, atypical pathways connecting the posterior parietal cortices homotopically via the anterior and the posterior commissures, as well as the primary visual areas via the anterior commissure, have been reported in case studies (Tovar-Moll et al. 2014; van Meer et al. 2016). Another proposed neuroplastic response is a functional compensation via strengthening of intrahemispheric pathways in individuals with AgCC (Dennis 1976; Risse et al. 1978; Chiarello 1980). In fetuses with AgCC (n = 20), Jakab et al. (2015) showed weaker interhemispheric structural connectivity starting in the second trimester of gestation followed by stronger intrahemispheric structural connectivity starting specifically in the third trimester, compared to control fetuses with normal brain development. To date, strengthening of intrahemispheric pathways has not been explored in individuals born with AgCC, and the potential implications of this neuroplastic response for neurobehavioral outcomes are unknown.
AgCC is a heterogeneous condition. It can be complete or partial and may be isolated or associated with additional brain abnormalities such as cortical malformations (Paul et al. 2007; Edwards et al. 2014). Individuals with AgCC present with impairments of varying severity across a range of neurobehavioral domains (Paul et al. 2007; Siffredi et al. 2013). General cognitive ability in individuals with AgCC who come to clinical attention is typically in the low average range (Siffredi et al. 2013; Siffredi et al. 2018). A core syndrome of agenesis of the corpus callosum (AgCC) has recently been proposed around three main axes: (1) reduced interhemispheric transfer of sensory-motor information, (2) reduced cognitive processing speed, and (3) deficits in complex reasoning and novel problem solving (Brown and Paul 2019). These core deficits are then expressed as difficulties across different domains including executive functions, such as deficits in cognitive control (e.g., selective attention and inhibition) and cognitive flexibility (Marco et al. 2012; Siffredi et al. 2018; Brown and Paul 2019), learning and memory (Erickson et al. 2014; Paul et al. 2016), cognitive processing speed (Marco et al. 2012; Erickson et al. 2014), and social and emotional cognition (Anderson et al. 2017). Nevertheless, consistent with the heterogeneity of their neuroimaging profile, neurobehavioral outcomes vary (Siffredi et al. 2018), and the role of neuroplastic responses in explaining this variability remains unclear.
This study aimed to assess brain structural and functional connectivity at the whole-brain and regional level, and their associations with neurobehavioral outcomes across general cognitive, executive, memory, and social domains, in a uniquely large cohort of children with AgCC compared with typically developing controls (TDC). We hypothesized a neuroplastic response resulting in strengthening of intrahemispheric pathways in children with AgCC accounting for the documented variability in neurobehavioral outcomes in this population. More specifically, we expected strengthening of intrahemispheric pathways to be associated with better neurobehavioral outcomes in children with AgCC.
Materials and Methods
Sample
This study used data from the “Pediatric Agenesis of the Corpus Callosum Project” conducted at the Murdoch Children’s Research Institute (Siffredi et al. 2018), which examined neurobehavioral, neurological, and neuroimaging outcomes in children with AgCC (n = 28) compared with typically developing children (n = 30). This project was approved by the Royal Children’s Hospital (RCH) Human Ethics in Research Committee. Written informed consent was obtained from the parents or legal guardians prior to participation. A cohort of 28 children with AgCC was recruited from clinics and radiology records at The RCH in Melbourne, Australia. Inclusion criteria were as follows: 1) aged 8 years 0 months to 16 years and 11 months, 2) documented evidence of AgCC on magnetic resonance imaging (MRI) conducted as part of a routine clinical work-up, 3) English speaking, and 4) functional ability to engage in the assessment procedure. MRI findings were qualitatively reviewed by a pediatric neurologist with expertise in brain malformations (RJL), who confirmed diagnosis of AgCC, including complete and partial AgCC, and identified associated brain malformations. A TDC group of 30 children comparable in age and sex to the AgCC group was recruited through advertisement in local schools and through staff at the RCH.
Children were included in the current study if they had completed the required MRI sequences (T1, DWI and resting-state fMRI), resulting in the exclusion of five participants from the AgCC group and one participant from the TDC group. After quality checking the functional and structural MR images, two AgCC participants were excluded from the structural connectivity analysis, while six AgCC and one TDC participants were excluded from the functional connectivity analysis. One children with AgCC was also excluded due to atypical commissural elements. Seven children were assessed on two separate occasions, for whom the most complete of the two neurobehavioral assessments was used, as well as the MRI scan complete at the time of the most complete neurobehavioral assessment.
The final sample size for the structural connectivity analysis was 20 children with AgCC and 29 TDC, and the final sample size for the functional connectivity analysis was 16 children with AgCC and 28 TDC.
Procedure
Consenting families were seen at a research clinic at the Murdoch Children’s Research Institute. Child neurobehavioral assessment was conducted by a training psychologist with expertise in assessments in children, using standardized tests that were developmentally appropriate for children and commonly used in clinical settings.
Materials
Neuroimaging
Image Acquisition
Images were acquired on a 3 T MAGNETOM Trio scanner (Siemens, Erlangen, Germany) at the RCH. A 32-channel head coil was used for transmission and reception of radio frequency and signals. An anatomical image was acquired using a T1-weighted MP-RAGE sequence (TR = 1900 ms, TE =2.71 ms, TI = 900 ms, FA = 9°, FoV = 256 mm, voxel size = 0.7 × 0.7 × 0.7 mm). Echo planar diffusion-weighted imaging (DWI) data were acquired at two different b-values, including two scans without diffusion weighting (b-factor = 0): a) b-value = 3000 s/mm2, 50 gradient directions where 54 slices with isotropic voxels of 2.3 mm3 were obtained (TR = 8200 ms; TE = 112 ms; FoV = 240 mm) in an anterior to posterior direction and b) b-value = 1000s/mm2, 30 gradient directions where 64 slices with isotropic voxels of 2 mm3 were obtained (TR = 8600 ms; TE = 90 ms; FoV = 256 mm) in an anterior to posterior direction. Resting-state gradient-echo EPI sequences was also acquired (196 frames, TR = 2000 ms, TE = 30 ms, voxel size: 2.6 × 2.6 × 4 mm, FA: 90 deg, FoV = 250 × 250 mm). During the resting-state functional MRI (fMRI) sequence, participants were instructed to keep their eyes closed and engage into mind wandering.
Structural and Functional MRI Data Preprocessing and Analyses
A flowchart summarizes brain connectivity preprocessing and analyses, see Figure 1.
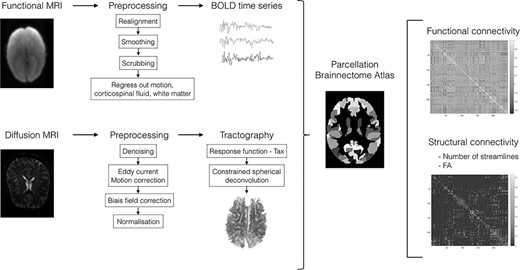
Flowchart summarizing the computation and analysis of functional and structural brain connectivity. Briefly, fMRI BOLD time courses/DWI fiber pathways were extracted, both data were parcellated using the Brainnetome atlas. Individual connectivity matrices were generated by computing pairwise functional connectivity (i.e., Pearson’s correlations) between regional timeseries and structural connectivity (i.e., number of streamlines and mean FA) between brain regions.
Briefly, DW images were preprocessed using MRtrix 3 (MRtrix package, 2010). Probabilistic tractograms and subsequent filtering using spherical deconvolution informed filtering of tractograms were completed (Smith et al. 2013). For each participant, the structural connectome matrix was generated from the resulting tractography using the registered Brainnetome Atlas (Fan et al. 2016). Two 246 × 246 connectivity matrices, referred to as SC, were obtained using the following connectivity metrics: 1) number of streamlines, that is, the number of reconstructed white matter fibers generated by fiber tractography, weighted for the sum of the volumes of the connected regions—more specifically, this matrix consisted of the number of reconstructed streamlines connecting each pair of the 246 regions of the Brainnetome atlas and 2) average fractional anisotropy (FA) computed by averaging FA values on all voxels within streamlines connecting each pair of the 246 regions of the Brainnetome atlas. The FA metric reflects the degree of water diffusion directionality; voxels containing water that moves predominantly along a single direction have higher FA. In white matter, FA is believed to depend on the microstructural features of fiber tracts, including the relative alignment of individual axons, the degree of myelination and axonal density and/or integrity (Arfanakis et al. 2002; Harsan et al. 2006; Paus 2016).
Resting-state fMRI data were preprocessed and analyzed using SPM12 using the pipeline described by Preti and Van De Ville (2017). Following Power et al. recommendations, we implemented volume censoring (“scrubbing”) for head-motion correction using a framewise displacement of 0.5-mm threshold for exclusion (Power et al. 2012; Power et al. 2014). Participants with less than 125 frames remaining after scrubbing were excluded. Using this approach, six AgCC and one TDC participants were removed from further analyses showing much more motion in the AgCC group than in the TDC group before scrubbing. After scrubbing, there was no difference in framewise displacement between the AgCC (mean (SD) = 0.22 (0.12)) and the TDC (mean (SD) =0.18 (0.09)) groups, t(42) = 1.297, P = 0.202. Region-averaged time series were extracted in each participant’s individual space using the registered Brainnetome Atlas. We computed pairwise Pearson correlation coefficients between all pairs of regions to obtain a symmetric correlation matrix of 246 × 246 for each participant, referred to as functional connectivity (FC).
For both SC and FC measures, average values of all interhemispheric connections (left–right), and intrahemispheric connections (right to right and left to left) were computed for each participant. Lobewise SC and FC was also computed for intra- and interhemispheric connectivity, see Supplemental Methods and Materials.
Neurobehavioral Outcome Measures
Associations with SC and FC and variation in neurobehavioral outcomes were examined using 33 age-standardized measures administered as part of the “Pediatric Agenesis of the Corpus Callosum Project” assessing five neurobehavioral domains; general cognitive functioning, short-term and working memory, executive and attentional functions, learning and memory, as well as social functions, see Supplemental Methods and Materials and supplementary Table S1.
Statistical Analyses
Group differences in whole brain intrahemispheric and interhemispheric connectivity for SC (number of streamlines and mean FA) and FC were compared between the following groups: 1) children with AgCC versus TDC, 2) complete versus partial AgCC, and 3) isolated versus complex AgCC (i.e., AgCC associated with other brain malformations). Group differences between children with AgCC and TDC were also examined for lobewise intra- and interhemispheric SC and FC. When exploring data distributions in the two groups, none of the assumptions for parametric tests were respected. Therefore, group differences were investigated with the Wilcoxon signed-ranks test using R software Version 1.1.463 (http://www.r-project.org; R Project for Statistical Computing, Vienna, Austria). P values, as well as q values corrected for multiple comparisons using a false discovery rate (FDR, q < 0.05) correction (Benjamini and Hochberg 1995), were reported for all SC and FC comparisons (n = 75).
To evaluate associations between connectivity (i.e., intra- and interhemispheric SC and FC) and neurobehavioral measures, we used partial least squares correlation (PLSC) analyses. PLSC is a multivariate data-driven statistical technique that maximizes the covariance between two matrices by deriving latent components, which are optimal linear combinations of the original matrices (McIntosh and Lobaugh 2004; Krishnan et al. 2011) and described in previous work (Kebets et al. 2019; Zoller et al. 2019). PLSC allows comparison between multiple response variables and multiple explanatory variables. PLSC was designed to deal with multiple regressions in the context of small sample sizes or multicollinearity (Pirouz 2006). The neurobehavioral (behavior) and brain connectivity variables were first converted to z-scores within each group (AgCC and TDC). The upper triangular part of the FC and SC matrices were vectorized, and both structural connectivity vectors (containing FA measures and number of streamlines) were stacked together. A cross-covariance matrix (which is effectively a correlation matrix, since the data are z-scores) was then computed between the neurobehavioral and brain connectivity matrices for each group, which were then concatenated. Singular value decomposition was then applied to this cross-covariance matrix, resulting in latent components. Each latent component is composed of a set of neurobehavioral weights (different for each group) and brain connectivity weights (common to all participants), which indicate how strongly each neurobehavioral and brain connectivity variable contribute to the multivariate brain connectivity-neurobehavioral association. Therefore, each group has its own neurobehavioral pattern associated to a common brain connectivity pattern across both the AgCC and TDC groups. The significance of latent components was determined by permutation testing (1000 permutations). Stability of neurobehavioral and brain connectivity weights was estimated using bootstrapping (500 bootstrap samples with replacement). Bootstrap ratio z-scores for each neurobehavioral and brain connectivity variables were obtained by dividing each neurobehavioral and brain connectivity weight by its bootstrap-estimated standard deviation, and a P value was obtained for each bootstrap ratio z-score. The contribution of neurobehavioral and brain connectivity weights for a given latent component was considered robust at P < 0.01 (i.e., absolute bootstrap ratio z-scores above 3). We used an in-house Matlab code to perform the analysis: https://miplab.epfl.ch/index.php/software/PLS. Two separate PLSC analyses were conducted to test for associations between neurobehavioral variables and SC, and between neurobehavioral variables and FC, respectively.
Results
Sample Characteristics
The characteristics of the participants in the AgCC and the TDC groups are presented in Table 1 for each of the SC and FC analyses, given the samples differed for the two analyses. For detailed clinical characteristics of the AgCC, see Supplementary Table S2. Twenty children with AgCC, including 13 with complete AgCC (four isolated, nine complex) and seven with partial AgCC (three isolated, four complex), as well as 29 TDC, were included in the SC analysis. For the FC analysis, 16 children with AgCC, including 10 with complete AgCC (three isolated, seven complex) and six with partial AgCC (three isolated, three complex), as well as 28 TDC met the inclusion criteria.
Characteristics of the agenesis of the corpus callosum and typically developing control groups included in the structural connectivity and functional connectivity analyses
. | AgCC . | TDC . | Group comparison . |
---|---|---|---|
Structural connectivity (SC) | |||
n | 20 | 29 | - |
Age in years, mean (SD)[range] | 12.08 (2.63)[8.67-17.08] | 11.75 (2.32)[8–16.42] | t(47) = 0.048, P = 0.642 |
Sex, n | 7 females, 13 males | 13 females, 16 males | X2(1, n = 49) = 0.473, P = 0.491 |
Handedness, n | 11 R, 8 L, 1 M | 26 R, 3 L | - |
Full-scale IQ, median [range] | 75.5 [66–126] | 113 [88–136] | W = 964.5, P < 0.001 |
Functional connectivity (FC) | |||
n | 16 | 28 | - |
Age in years, mean (SD)[range] | 12.16 (2.75)[8.67–17.08] | 11.71 (2.35)[8–16.42] | t(42) = 0.567, P = 0.572 |
Sex, n | 6 females, 10 males | 12 females, 16 males | X2(1, n = 44) = 0.121, P = 0.728 |
Handedness, n | 10 right, 5 left, 1 Mix | 25 Right, 3 Left | - |
Full-scale IQ, median [range] | 76 [67–126] | 114.5 [88–136] | W = 808.5, P < 0.001 |
. | AgCC . | TDC . | Group comparison . |
---|---|---|---|
Structural connectivity (SC) | |||
n | 20 | 29 | - |
Age in years, mean (SD)[range] | 12.08 (2.63)[8.67-17.08] | 11.75 (2.32)[8–16.42] | t(47) = 0.048, P = 0.642 |
Sex, n | 7 females, 13 males | 13 females, 16 males | X2(1, n = 49) = 0.473, P = 0.491 |
Handedness, n | 11 R, 8 L, 1 M | 26 R, 3 L | - |
Full-scale IQ, median [range] | 75.5 [66–126] | 113 [88–136] | W = 964.5, P < 0.001 |
Functional connectivity (FC) | |||
n | 16 | 28 | - |
Age in years, mean (SD)[range] | 12.16 (2.75)[8.67–17.08] | 11.71 (2.35)[8–16.42] | t(42) = 0.567, P = 0.572 |
Sex, n | 6 females, 10 males | 12 females, 16 males | X2(1, n = 44) = 0.121, P = 0.728 |
Handedness, n | 10 right, 5 left, 1 Mix | 25 Right, 3 Left | - |
Full-scale IQ, median [range] | 76 [67–126] | 114.5 [88–136] | W = 808.5, P < 0.001 |
Note: Full-scale IQ was measured using the Wechsler Abbreviated Intelligence Scale (WASI) or the Wechsler Intelligence Scale for Children, 4th edition (WISC-IV; n = 3) where the mean standardized score is M = 100 and SD = 15; handedness was estimated by the Edinburgh Handedness Inventory with the following scores: right-handed = +40 to +100, left-handed = −40 to −100, mixed handed = −40 to +40.
Characteristics of the agenesis of the corpus callosum and typically developing control groups included in the structural connectivity and functional connectivity analyses
. | AgCC . | TDC . | Group comparison . |
---|---|---|---|
Structural connectivity (SC) | |||
n | 20 | 29 | - |
Age in years, mean (SD)[range] | 12.08 (2.63)[8.67-17.08] | 11.75 (2.32)[8–16.42] | t(47) = 0.048, P = 0.642 |
Sex, n | 7 females, 13 males | 13 females, 16 males | X2(1, n = 49) = 0.473, P = 0.491 |
Handedness, n | 11 R, 8 L, 1 M | 26 R, 3 L | - |
Full-scale IQ, median [range] | 75.5 [66–126] | 113 [88–136] | W = 964.5, P < 0.001 |
Functional connectivity (FC) | |||
n | 16 | 28 | - |
Age in years, mean (SD)[range] | 12.16 (2.75)[8.67–17.08] | 11.71 (2.35)[8–16.42] | t(42) = 0.567, P = 0.572 |
Sex, n | 6 females, 10 males | 12 females, 16 males | X2(1, n = 44) = 0.121, P = 0.728 |
Handedness, n | 10 right, 5 left, 1 Mix | 25 Right, 3 Left | - |
Full-scale IQ, median [range] | 76 [67–126] | 114.5 [88–136] | W = 808.5, P < 0.001 |
. | AgCC . | TDC . | Group comparison . |
---|---|---|---|
Structural connectivity (SC) | |||
n | 20 | 29 | - |
Age in years, mean (SD)[range] | 12.08 (2.63)[8.67-17.08] | 11.75 (2.32)[8–16.42] | t(47) = 0.048, P = 0.642 |
Sex, n | 7 females, 13 males | 13 females, 16 males | X2(1, n = 49) = 0.473, P = 0.491 |
Handedness, n | 11 R, 8 L, 1 M | 26 R, 3 L | - |
Full-scale IQ, median [range] | 75.5 [66–126] | 113 [88–136] | W = 964.5, P < 0.001 |
Functional connectivity (FC) | |||
n | 16 | 28 | - |
Age in years, mean (SD)[range] | 12.16 (2.75)[8.67–17.08] | 11.71 (2.35)[8–16.42] | t(42) = 0.567, P = 0.572 |
Sex, n | 6 females, 10 males | 12 females, 16 males | X2(1, n = 44) = 0.121, P = 0.728 |
Handedness, n | 10 right, 5 left, 1 Mix | 25 Right, 3 Left | - |
Full-scale IQ, median [range] | 76 [67–126] | 114.5 [88–136] | W = 808.5, P < 0.001 |
Note: Full-scale IQ was measured using the Wechsler Abbreviated Intelligence Scale (WASI) or the Wechsler Intelligence Scale for Children, 4th edition (WISC-IV; n = 3) where the mean standardized score is M = 100 and SD = 15; handedness was estimated by the Edinburgh Handedness Inventory with the following scores: right-handed = +40 to +100, left-handed = −40 to −100, mixed handed = −40 to +40.
Of note, there was a large variability in the Full-Scale Intellectual Quotient (IQ) score, ranging from 66 to 126 in children with AgCC, and three children had an FSIQ below 70 (i.e., 2 SD or more below the test mean, in the extremely low range). A sensitivity analysis excluding these three children from the FC and SC analyses did not change our results.
Structural Connectivity
For a summary of the whole brain and lobe-based SC and FC findings, see Figure 2.
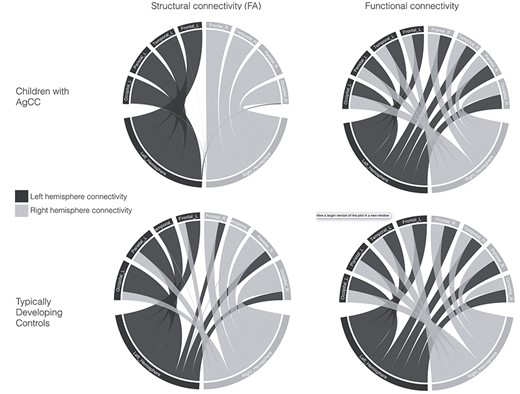
Summary of structural connectivity (fractional anisotropy, FA) and functional connectivity in children with AgCC and TDC. Gray lines represent connectivity in the right hemisphere, and blue lines represent connectivity in the left hemisphere. There is a shift from interhemispheric to intrahemispheric structural connectivity in children with AgCC compared to the TDC group, while the pattern of functional connectivity is similar between the two groups.
For whole-brain SC measures (number of streamlines and FA), interhemispheric connectivity was significantly reduced in children with AgCC compared with the TDC group (q < 0.0001), see Supplementary Figure S1 and Supplementary Table S3. In contrast, whole-brain intrahemispheric SC was significantly increased in children with AgCC compared with the TDC group (q < 0.0001 to q = 0.006). Within the AgCC group, there were no significant differences in either intra- or interhemispheric whole-brain SC in children with complete compared with partial AgCC, or in children with isolated AgCC compared with complex AgCC, see Supplementary Tables S4 and S5.
At the lobe level, children with AgCC had significantly reduced interhemispheric connectivity (q ≤ 0.0001 to q = 0.0339) and increased intrahemispheric connectivity for frontal, parietal and temporal lobes (q ≤ 0.0001 to q = 0.0201) compared with the TDC group, except for the number of interhemispheric streamlines connecting temporal lobes to all contralateral lobes and the mean FA of streamlines connecting the left temporal lobe to all contralateral lobes, see Supplementary Table S6. Regarding occipital lobes, children with AgCC showed a significant increase in the number of streamlines in the left occipital lobe for intrahemispheric connectivity and a significant decreased in interhemispheric connectivity compared to the TDC group. Moreover, children with AgCC had reduced mean FA in all occipital intra- and interhemispheric streamlines compared to the TDC group (q ≤ 0.001 to q = 0.01).
Functional Connectivity
For whole-brain FC, intra- and interhemispheric connectivity did not differ significantly between the AgCC and TDC groups. Within the AgCC group, there were no significant differences in either intrahemispheric or interhemispheric whole-brain FC in children with complete compared with partial AgCC, or between children with isolated compared with complex AgCC, see Supplementary Figure S2 and Supplementary Tables S7–S9.
For all lobes considered (frontal, parietal, temporal, occipital), there was no difference in either intra- or interhemispheric FC between the AgCC and TDC groups, see Supplementary Table S10.
Associations Between Brain Connectivity and Neurobehavioral Outcomes
For neurobehavioral scores and group comparisons, see Supplementary Table S11.
The PLSC analysis applied on SC and neurobehavioral measures in the AgCC and TDC groups identified three statistically significant latent components: latent component 1 (P = 0.002) explained 35% of the covariance between structural connectivity and neurobehavioral measures, while latent component 2 (P = 0.032) and latent component 3 (P = 0.020) explained 22% and 5%, respectively. Based on the amount of explained covariance and clinical interpretability of the results (Krishnan et al. 2011), we chose to focus on latent component 1. Latent component 1 revealed common as well as distinct patterns of associations in each group, see Figure 3. Both the AgCC and TDC groups showed increased intrahemispheric and reduced interhemispheric mean FA and number of streamlines associated with better dual task performance, indexing multimodal divided attention, and working memory. In the AgCC group, increased intrahemispheric and reduced interhemispheric mean FA and number of streamlines were associated with better neurobehavioral outcomes, including verbal learning and memory (including working memory), as well as attentional and executive functions, specifically sustained, selective and divided attention, processing speed, switching, verbal fluency, and inhibition. In contrast, in the TDC group, increased interhemispheric and reduced intrahemispheric mean FA and number of streamlines were associated with better performance in similar neurobehavioral measures, including verbal learning and memory, as well as attentional and executive functioning such as processing speed, switching and verbal fluency, but also in general neurobehavioral functioning (FSIQ and PIQ).
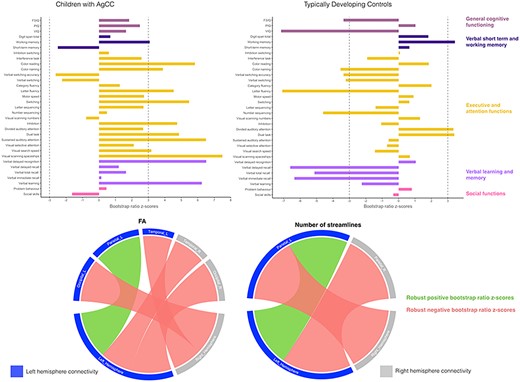
Associations between neurobehavioral outcomes and structural connectivity in the AgCC and TDC groups based on the PLSC analysis. Neurobehavioral weights are specific to each group, while brain connectivity weights are common to both the AgCC and TDC groups. Diverging graphs show bootstrap ratio z-scores (x-axis) for each neurobehavioral measure (y-axis); neurobehavioral measures are colored by domains. Neurobehavioral measures with an absolute bootstrap ratio z-score above or equal to 3, indicated by a dash-dotted line on the graph, yield a robust contribution to the component. Circular graphs show robust bootstrap ratio z-scores (absolute bootstrap ratio z-scores above or equal to 3) for FA and number of streamlines lobewise in inter- and intrahemispheric connectivity. Original saliences as well as their bootstrap-estimated standard deviations and bootstrap ratio z-scores for all neurobehavioral and connectivity measures are reported in Supplementary Table S12.
The second PLSC analysis was applied on FC and neurobehavioral measures and identified only one significant latent component (P = 0.002), which explained 96% of the covariance between FC and neurobehavioral measures. In both groups, increased intrahemispheric and interhemispheric FC were associated with specific aspects of executive and attentional functioning, including visual scanning, selective attention and processing speed, see Figure 4. In the AgCC group, a robust negative association between FC and verbal recall was found. Interestingly, the association with dual task performance had an opposite effect in the two groups, with higher scores associated with an overall increase in FC in the AgCC group, whereas higher scores in the TDC group were associated with a decrease in FC. Finally, in the TDC group, a general increase in FC was also associated with VIQ.
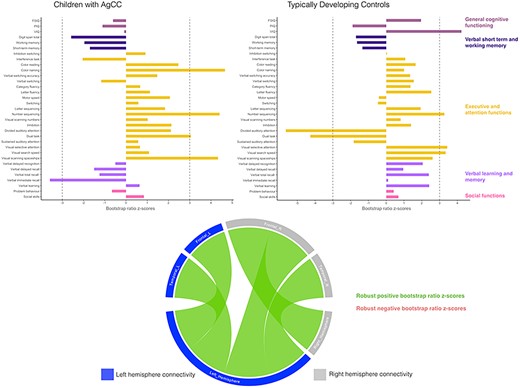
Associations between neurobehavioral outcomes and functional connectivity in the AgCC and TDC groups based on PLSC analyses. Neurobehavioral weights are specific to each group, while brain connectivity weights are common to both the AgCC and TDC groups. Diverging graphs show bootstrap ratio z-scores (x-axis) for each neurobehavioral measure (y-axis). Bootstrap ratio z-scores above or equal to 3 specify by a dash-dotted line on the graph and indicate a robust positive correlation; bootstrap ratio z-scores below or equal to −3 specify by a dash-dotted line on the graph and indicate a robust negative correlation. Circular graphs show robust bootstrap ratio z-scores for FC lobewise in inter- and intrahemispheric connectivity; left regions and left hemisphere are in dark blue, right regions and hemisphere are in gray, positive robust correlations are indicated in green and negative robust correlations are indicated in orange at P < 0.01. Salience, bootstrap-estimated standard deviations, and bootstrap ratio z-scores for all neurobehavioral and connectivity measures are reported in Supplementary Table S13.
Discussion
This is the first study to explore neuroplastic responses in a uniquely large cohort of children born without a corpus callosum using both structural and functional brain connectivity, and to examine their association with neurobehavioral outcomes. We show evidence of structural strengthening of intrahemispheric pathways in children with AgCC, which seems to allow for inter- and intrahemispheric functional connectivity comparable to typically developing brain. In children with AgCC, this increase in intrahemispheric SC was associated with better neurobehavioral outcomes including verbal learning and long-term memory, verbal working memory, as well as executive and attentional functioning.
While whole-brain interhemispheric SC was reduced in children with AgCC compared to TDC, a significant increase in whole-brain intrahemispheric SC was demonstrated in children with AgCC. These findings are in accordance with SC results of a previous study conducted in AgCC fetuses (Jakab et al. 2015), suggesting that the neuroplastic responses began in utero and not in response to postnatal environmental factors. They are also in line with network metrics computed from to SC data in AgCC individuals showing abnormally reduced global connectivity but increased local connectivity compared to healthy controls (Owen et al. 2013b). The neuroplastic responses observed could be linked to microstructural alterations that are known to take place at the cellular or synaptic level (Klug et al. 2012). It is possible that early in utero disruption in cortical developmental mechanisms allows for long-distance plasticity through atypical pivotal cellular and molecular mechanisms that divert axon growth and guidance through entirely new pathways and connections (Tovar-Moll et al. 2007; Tovar-Moll et al. 2014). Regarding FC, and in contrast to the reduced interhemispheric FC reported in callosotomy patients (Roland et al. 2017), we found no difference between children with AgCC and TDC children for either intrahemispheric or interhemispheric FC. These findings are in accordance with previous FC results showing normal resting-state networks and intact functional coupling between hemispheres in individuals with AgCC compared to healthy controls (Tyszka et al. 2011; Owen et al. 2013a). Altogether, our findings are in line with the hypothesis of strengthening of intrahemispheric pathways at the structural level as a neuroplastic response in children with AgCC, with a shift from interhemispheric connectivity to intrahemispheric connectivity. They also imply that the relationship between SC and FC is regulated by complex and complementary processes (Uddin 2013). Structural neuroplastic responses through strengthening of intrahemispheric pathways might underlie the compensatory mechanisms that lead to comparable patterns of intrahemispheric and interhemispheric FC that is observed in a typically developing brain. It is also possible that other commissural structures, in particular, the anterior commissure, play a role in maintaining interhemispheric FC comparable to the typically developing brain as shown in rhesus monkeys (O'Reilly et al. 2013) and suggested in humans (Tovar-Moll et al. 2014).
Complete and partial AgCC showed no difference in intrahemispheric and interhemispheric SC or FC. As the corpus callosum develops very early during brain development, the immature human brain might engage similar neuroplastic responses at the whole brain level, independent of extent of the corpus callosum agenesis. In addition, children with isolated AgCC and complex AgCC (i.e., associated with other brain malformations) did not show any difference in either whole-brain intrahemispheric or interhemispheric SC and FC. Therefore, despite the presence of additional brain malformations, children with complex AgCC seem to have a comparable level of plasticity in term of strengthening of intrahemispheric pathways to those with isolated AgCC.
When exploring connectivity in different brain lobes, patterns of both SC and FC in frontal, parietal, and temporal lobes were consistent with results obtained at the whole-brain level. Occipital lobes showed a different profile, with a global FA reduction in AgCC, but similar streamlines count and FC for intrahemispheric and interhemispheric connectivity compared to the TDC group. This reduction in FA values observed for all intrahemispheric and interhemispheric occipital streamlines could reflect sparse, poorly myelinated, or divergent fibers (Le Bihan et al. 2001; Beaulieu 2002). Regarding interhemispheric streamlines, these results are consistent with the potential existence of an atypical pathway connecting primary visual areas via the anterior commissure in individuals with AgCC showing a reduction in mean FA (Tovar-Moll et al. 2014; van Meer et al. 2016). The reduction of mean FA in intrahemispheric streamlines in occipital lobes could be associated with the presence of colpocephaly, a congenital brain abnormality characterized by enlargement of the posterior or rear portion of the lateral ventricles, almost uniformly seen when the corpus callosum is absent. This typically results in an enlargement of occipital horns of the lateral ventricles and is known to affect the development of occipital lobes, including white matter integrity in these regions (Ayflegül et al. 2016; Bartolome et al. 2016; Siffredi et al. 2018).
Different patterns of association between SC and neurobehavioral measures were observed between the AgCC and TDC groups. While in TDC, increased interhemispheric SC were associated with better neurobehavioral outcomes (including verbal learning and memory, as well as attentional and executive functioning), we observed the reverse pattern in AgCC with higher intrahemispheric SC and reduced interhemispheric SC associated with these neurobehavioral domains. The pattern observed in the AgCC group is in line with the young age plasticity privilege or “Kennard principle” (Kennard 1938; Anderson et al. 2011b). A very early disruption of embryological callosal development might lead to a complete (or almost complete) absence of axons crossing the midline. If disruption occurs slightly later in gestation, an increased number of callosal fibers might cross the midline and form interhemispheric tracks (Richards et al. 2004; Huang et al. 2009; Paul 2011). Therefore, it seems that a greater neuroplastic response and brain reorganization arise in the case of a marked reduction in interhemispheric pathways, observed when callosal disruption occurs at an earlier embryological stage. Conversely, and consistent with the literature, TDC seem to rely mostly on interhemispheric SC for verbal learning and memory, attentional, and executive functioning such as processing speed, switching, and verbal fluency, as well as general cognitive abilities (Hynd et al. 1991; Christman and Propper 2001; Luders et al. 2007; Siffredi et al. 2017; Chopra et al. 2018).
Patterns of association between FC and neurobehavioral outcomes were comparable for children with AgCC and TDC. Both intrahemispheric and interhemispheric FC were positively associated with executive and attentional functioning, including visual scanning, selective attention, and processing speed. Remarkably, dual task measures (including multi- or uni-modal divided attention and working memory), showed a comparable positive association with increased intrahemispheric and reduced interhemispheric SC in the two groups. However, FC also revealed distinct patterns of association between the two groups. In the AgCC group, higher dual task scores were associated with an overall increase in FC, whereas higher scores in the TDC group were associated with a global decrease in FC. These phenomena could be linked to hemispheric symmetry and parallel processing during divided attention task, as previously observed during dichotic listening in individuals with AgCC (Ocklenburg et al. 2015). However, this requires further investigation. Together, these findings show that the neuroplastic responses observed at the structural level might, at least partly, explain the heterogeneity in neurobehavioral outcomes in the AgCC population.
Remarkably, IQ was not found to be associated with intra- nor interhemispheric SC in children with AgCC. One possible explanation is that IQ in this population is probably more related to other factors such as the presence of additional brain anomalies, that is, isolated versus complex AgCC (Siffredi et al. 2018). Moreover, the neuropsychological syndrome of AgCC, as proposed by Brown and Paul (2019), describes specific difficulties in the domains of attention, executive functions, learning and memory, which were the domains found to be associated with strengthening of intrahemispheric pathways in AgCC. It is possible that neuroplastic responses specific to the AgCC population are associated with specific neurobehavioral difficulties observed in this same population. Finally, despite strong multicollinearity within one set of latent variables (e.g., IQ scores and executive functions scores), PLSC allows to disentangle the contribution of each (neurobehavioral or imaging) variable to the latent components, and to estimate the reliability of that contribution (using the bootstrapping procedure).
These findings can also be discussed in light of developmental neurobiological mechanisms regulating corpus callosum formation. These mechanisms are complex and involve a large number of genes (reviewed in Paul et al. 2007; Edwards et al. 2014). Classical embryological studies described a unique anatomical region within the brains of many mammalian species, where all telencephalic commissures initially cross the interhemispheric midline, called “the commissural plate” (Rakic and Yakovlev 1968). Rakic and Yakovlev (1968) proposed that the human commissural plate can be anatomically subdivided into the massa commissuralis through which the corpus callosum and hippocampal commissure cross the midline, and the area septalis through which the anterior commissure crosses. More recently, neuroimaging studies of human embryology and animal models have contributed a clearer understanding of neurobiological mechanisms implicated in corpus callosum formation. In early stages of development, it has been shown that expression of the morphogen FGF8 at the midline plays an important role in patterning and formation of midline tissue (Moldrich et al. 2010). Expression of FGF8 also regulates the further differentiation of the commissural plate into four distinct molecular subdomains, each specified by midline patterning molecules (Moldrich et al. 2010). The corpus callosum passes through a domain of EMX1 and NFIA expression (Boncinelli et al. 1993; Plachez et al. 2008). Midline glial structures, including the glial wedge, midline zipper glia, and indusium griseum, are then involved in guiding callosal axons (Shu and Richards 2001; Shu et al. 2003; Smith et al. 2006; Tole et al. 2006).
At 11–12 weeks’ postconception, pioneering axons are the first axons to grow along a specific trajectory and cross the commissural plate in the hippocampal primordium (Barkovich et al. 1992; Kier and Truwit 1996; Huang et al. 2009). These pioneer axons arise from the cingulate cortex and provide a necessary path for the fasciculation of later-arriving neocortical axons (Rash and Richards 2001). In humans, these pioneer axons express the guidance receptor neuropilin 1 NRP1 (Ren et al. 2006). Notably, this receptor protein NRP1 has been associated with increased risk for neuropsychiatric and neurodevelopmental disorders, including autism spectrum disorder (de Anda et al. 2012; Chen et al. 2014), schizophrenia (Ganapathiraju et al. 2016), and major depressive disorder (Goswami et al. 2013; Shiflett et al. 2017). After crossing the midline, callosal axons grow into the contralateral hemisphere towards their designated target region, usually homotopic to their region of origin (Paul 2011). Shortly after the pioneers cross the midline, the anterior sections begin to grow by 14–15 weeks’ postconception, whereas growth of the posterior sections occurs around the 18th and 19th week postconception (Barkovich et al. 1992; Kier and Truwit 1996; Huang et al. 2009). Callosal axons must recognize their target area and synapse with target neurons, presumably through molecular-recognition and activity-dependent mechanisms (Edwards et al. 2014).
By 20 weeks’ postconception, the corpus callosum has reached its final shape, although significant exuberant axonal growth continues after birth (Innocenti and Price 2005). The exuberant development of neural circuits involves the formation of long, transient axonal projections, the overproduction of short axonal branches and synapses, and the overproduction of dendritic branches and/or spines (Innocenti and Price 2005). In monkeys at birth, the number of callosal axons exceeds the number of callosal axons present in the adult monkey by at least 3.5 times (LaMantia and Rakic 1990a, 1990b). This exuberant axonal growth, salient in callosal formation, is then accompanied by selection and refinement that eventually leads to maintenance of some of the juvenile structures and elimination of others by molecular- and activity-dependent axonal pruning (Innocenti and Price 2005). In the human brain, decrease in size of the midsagittal cross-sectional area, suggesting the beginning of a retraction of exuberant callosal axons, has been observed after 32 weeks’ postconception and lasts until the second postnatal month (Clarke et al. 1989). It occurs concomitantly with the resolution of waiting compartments (Kostovic and Rakic 1990) and the resolution of the majority of the callosal septa (Jovanov-Milosević et al. 2006). The remnants of septa that continue after birth may help in the process of withdrawal of exuberant callosal axons, since these structures and processes temporally overlap (Jovanov-Milosevic et al. 2009). It is possible that these processes of selection and refinement of exuberant callosal axons play a role in the formation of strengthening of ipsilateral pathways, but this requires further investigation.
As a consequence of these complex cellular and molecular mechanisms, AgCC can result from alteration of numerous developmental events from early midline telencephalic patterning to neuronal migration and specification, axon guidance, and postguidance development. This complexity reflects the heterogeneous nature of clinical presentations of individuals with AgCC. A better understanding and identification of genetic and environmental determinant mechanisms in individuals with AgCC could inform the different neurological symptoms and neuroplastic responses occurring in this population. Moreover, molecular disruptions regulating corpus callosum development, such as NRP1 involved in axonal guidance, are candidates for increased risk of autism spectrum disorder, schizophrenia, and major depressive disorder (de Anda et al. 2012; Goswami et al. 2013; Chen et al. 2014; Ganapathiraju et al. 2016; Shiflett et al. 2017). These findings are also in line with studies showing callosal structural alterations in these neurodevelopmental and psychiatric disorder (Barnea-Goraly et al. 2004; Hardan et al. 2009; Kumar et al. 2010), schizophrenia (Foong et al. 2000), and major depressive disorder (Cole et al. 2012). Together, this previous work suggests that AgCC can serve as a model developmental disorder and allow for the identification of a shared repertoire of genetic, neurological, and behavioral markers with a number of neurodevelopmental and psychiatric disorders.
A major strength of our study is the use of both functional and structural connectivity with a comprehensive set of neurobehavioral measures in a large cohort of children with AgCC. To our knowledge, the present study is the first to use a whole-brain data-driven approach in this population. In light of the literature, our cohort is uniquely large for this pathology with regard to measuring structural and functional connectivity alongside neurobehavioral outcomes. Studies combining neuroimaging and behavioral assessment in this population are rare, and sample sizes are typically smaller than in the present study (Lum et al. 2011; Owen et al. 2013a; Tovar-Moll et al. 2014). Nevertheless, our findings should be considered in light of some limitations. Our cohort is heterogeneous in terms of corpus callosum agenesis (i.e., complete or partial AgCC) and associated brain malformations (i.e., isolated or complex AgCC). Even if we did not find any significant differences across these subgroups, further investigations with larger samples of each phenotypic group, possibly achieved through recruitment across multiple sites, are necessary to better understand underlying mechanisms, as well as replicate our findings. Such an approach would also allow exploring the heterogeneous clinical and neuroimaging profiles of children with AgCC, and the factors that might contribute to a better understanding of neurobehavioral outcomes. The combination and extension of these findings in animal models could also significantly improve our understanding of the mechanisms involved. Finally, future studies using graph analysis of structural and functional neuroimaging data combined with clinical measures could lead to a better characterization of brain dysfunction in terms of aberrant reconfiguration of brain networks in AgCC.
Conclusions
In conclusion, we provide evidence of structural strengthening of intrahemispheric pathways in children born without corpus callosum, which seems to allow for functional connectivity comparable to a typically developing brain. In children with AgCC, this increase in intrahemispheric SC, probably reflecting very early disruption of callosal development during gestation, seems to be associated with better verbal learning and long-term memory, verbal working memory, as well as executive and attentional functioning. This work provides novel evidence improving our current understanding of the properties of structural and functional neuroplastic responses in individuals with AgCC. In turn, these neuroplastic responses might be relevant to explain the broad range of clinical presentations and the high heterogeneity in neurobehavioral outcomes observed in this population. Our findings may apply more broadly to other congenital brain malformations in which a similar process of compensation by in utero reorganization may occur, as well as to neurodevelopmental disorder in which corpus callosum alteration is observed such as autism spectrum disorder, attention deficit hyperactivity disorder, or developmental delay.
Funding
The Boninchi Foundation from the University of Geneva; the Victorian Government’s Operational Infrastructure Support Program; and the Murdoch Children’s Research Institute. European Research Council Consolidator Fellowship (682734 to A.W.). A Melbourne Children’s Clinician Scientist Fellowship (to R.L.). The Australian National Health and Medical Research Council Senior Practitioner Fellowship (to V.A.); The CIBM Center for Biomedical Imaging (to M. G. P.).
Notes
We gratefully acknowledge the families who participated in this study and Kate Pope for her assistance in recruitment of the families.