-
PDF
- Split View
-
Views
-
Cite
Cite
Xianbin Wang, Ting Wang, Dong Zhu, Jing Wang, Weijie Han, From acute lung injury to cerebral ischemia: a unified concept involving intercellular communication through extracellular vesicle-associated miRNAs released by macrophages/microglia, Clinical and Experimental Immunology, Volume 219, Issue 1, 2025, uxae105, https://doi.org/10.1093/cei/uxae105
- Share Icon Share
Abstract
Ischemic stroke and acute lung injury are prevalent life-threatening conditions marked by intricate molecular mechanisms and elevated mortality rates. Despite evident pathophysiological distinctions, a notable similarity exists in the gene responses to tissue injury observed in both pathologies. This similarity extends to both protein-encoding RNAs and non-coding RNAs. Extracellular vesicles (EVs) are nano-scale vesicles derived through cell secretion, possessing unique advantages such as high biocompatibility, low immunogenicity, intrinsic cell targeting, and facile chemical and genetic manipulation. Importantly, miRNAs, the most prevalent non-coding RNAs, are selectively concentrated within EVs. Macrophages/microglia serve as immune defense and homeostatic cells, deriving from progenitor cells in the bone marrow. They can be classified into two contrasting types: classical proinflammatory M1 phenotype or alternative anti-inflammatory M2 phenotype. However, there exists a continuum of various intermediate phenotypes between M1 and M2, and macrophages/microglia can transition from one phenotype to another. This review will investigate recent discoveries concerning the impact of EVs derived from macrophages/microglia under various states on the progression of ischemic stroke and acute lung injury. The focus will be on the involvement of miRNAs within these vesicles. The concluding remarks of this review will underscore the clinical possibilities linked to EV-miRNAs, accentuating their potential as both biomarkers and therapeutic targets.
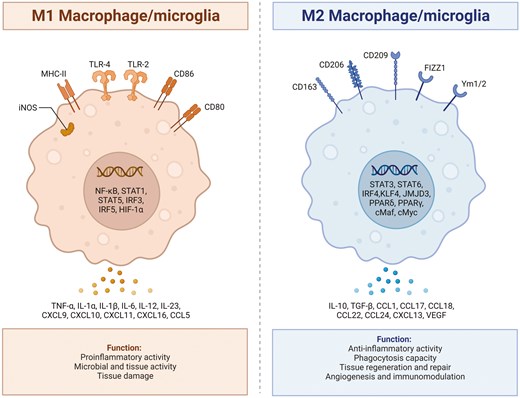
Introduction
Ischemic stroke and acute lung injury are common life-threatening diseases associated with severe inflammation, characterized by complicated molecular mechanisms and high mortality [1–3]. Acute lung injury refers to a sudden onset of hypoxic respiratory insufficiency resulting from diverse factors, both intra and extra-pulmonary. These factors include sepsis, trauma, ischemia-reperfusion, pneumonia, and mechanical ventilation. The condition leads to the breakdown of the barrier formed by capillary endothelial cells and alveolar epithelial cells [4, 5]. Ischemic stroke occurs when cerebrovascular diseases, often linked to vascular occlusions and atherosclerosis, interrupt blood supply to the brain, resulting in ischemic hypoxic necrosis within brain tissues [6, 7]. Ischemic stroke remains among the most incapacitating illnesses and a primary contributor to global mortality [8]. Despite advancements in medical interventions, the worldwide impact of stroke continues to escalate, given the absence of effective treatments capable of mitigating or reversing brain ischemic injury [8]. This initiates a cascade of inflammatory reactions, causing early-stage damage to both the brain and the blood-brain barrier [9, 10]. Despite evident pathophysiological distinctions, there is a significant resemblance in the gene responses to tissue injury across these two pathologies. These similarities extend to both protein-encoding RNAs and non-coding RNAs (ncRNAs) [11, 12].
Targeting ncRNAs, including microRNAs (miRNAs), long noncoding RNAs (lncRNAs), and circular RNAs (circRNAs), in a therapeutic context holds promise for addressing not only ischemic stroke but also acute lung injury [13, 14]. Due to alterations in gene expression, the fundamental aspects of cellular biology and communication undergo significant changes in injured conditions. Cell–cell communication has been demonstrated through various mechanisms, with the latest being the release of extracellular vesicles (EVs) [12]. As EVs transport a diverse range of cargoes, including nucleic acids, lipids, and proteins, they have become a focal point in the fields of diagnosis and treatment [12]. Significantly, miRNAs, the most prevalent ncRNAs [15], are selectively concentrated within EVs [16]. The transferred miRNAs in EVs play a crucial role in regulating diverse aspects of the onset and progression of ischemic stroke and acute lung injury, underscoring their significance as essential components.
In the realm of innate immunity, macrophages/microglia play a crucial role as versatile phagocytes. They express pattern recognition receptors to identify both pathogen-associated molecular patterns and damage-associated molecular patterns. Macrophages/microglia engage in communication with other cells within the tissue through cell-to-cell contact, secretion of molecules, and the release of EVs. Notably, the release of EVs facilitates regulatory processes across distant regions [17–19]. The demonstrated biological role of EV-derived miRNAs from macrophages/microglia in regulating distinct aspects of the onset and progression is evident in ischemic stroke and acute lung injury [20–22]. Nevertheless, there is a scarcity of data regarding the exact mechanisms that underlie this therapeutic approach. This review offers a concise overview of the influence of macrophages/microglia-derived EV-miRNAs in preclinical studies centered on the treatment of ischemic stroke and acute lung injury. The regulation of various signaling pathways in cellular processes is emphasized. Furthermore, we outline the potential clinical applications of EV-miRNAs as diagnostic and therapeutic tools for these two pathologies.
Biology of EVs and miRNAs
The biological characteristics of EVs
EVs were initially identified in plasma by Wolf in the 1960s [23]. Subsequently, various biological fluids have been examined, revealing the presence of vesicles, and in vitro cultured cell lines have demonstrated the release of vesicles to varying degrees [24]. EVs can be categorized into subgroups according to their origin, size, and physicochemical characteristics: microvesicles/endosomes (100–1000 nm), released by the plasma membrane; exosomes (30–150 nm), originating from multivesicular bodies (MVBs); and apoptotic bodies (100–5000 nm), generated through cellular apoptosis [25]. The majority of EVs originate from the direct outward budding and fission of the plasma membrane. First, the plasma membrane gives rise to endocytic vesicles (endosomes). Second, the inward budding of endosomal membranes leads to the formation of small vesicles. These small vesicles, along with various constituents, such as DNA, RNA, bioactive lipids, and proteins, are then assembled into exosomes within larger vesicles known as MVBs. Ultimately, under the regulation of the endosomal sorting complex required for transport (ESCRT) and specific proteins, EVs released into the extracellular environment are accomplished through the fusion of MVBs with the plasma membrane [26]. Upon production in the extracellular space, nanosized EVs can be internalized by recipient cells, functioning as messengers [27].
The biological characteristics of miRNAs
miRNAs are diminutive endogenous RNA molecules, typically 18–25 nucleotides in length, that exhibit a high degree of conservation [15]. Crucially involved in cell differentiation, proliferation, and survival. They achieve this by interacting with complementary target mRNAs, leading to either the inhibition of mRNA translation or its degradation [28, 29]. The biogenesis of miRNA involves a multipartite process, encompassing both nuclear and cytoplasmic phases. These molecules are generally transcribed by RNA polymerases II or III, yielding a lengthy primary miRNA (pri-miRNA) characterized by a cap and a poly-A tail [30]. The pri-miRNA then undergoes cleavage by a microprocessor complex, which consists of the RNA-binding protein DGCR8 and the type III RNase Drosha. This cleavage results in the formation of an 85-nucleotide stem-loop structure known as precursor miRNA (pre-miRNA) [31]. Afterward, RNase III DICER1 processes pre-miRNA into mature ~22-nucleotide microRNA duplexes. Following the unwinding of the duplex, one strand aligns with Ago2 (Argonaute-2) proteins, forming the RNA-induced silencing complex (RISC) [32].
miRNAs loading into EVs and subsequent release and uptake
As mentioned above, EVs encapsulate a diverse array of molecules, such as proteins, lipids, DNAs, mRNAs, and miRNAs. Among these components, miRNAs have garnered significant interest for their pivotal regulatory functions in gene expression [33]. Various mechanisms have been suggested for the selection of miRNAs as cargo in EVs. A sumoylated heterogeneous nuclear ribonucleoprotein (hnRNP), primarily comprising hnRNPA2B1, hnRNPA1, and hnRNPC, has been identified as being abundant in miRNAs within EVs. The loading of these miRNAs into MVBs seems to involve an interaction with the hnRNPA2B1 [34]. The second mechanism involves posttranscriptional modifications of miRNAs, specifically 3ʹ end uridylation, which seems to play a role in directly sorting miRNAs into EVs [35]. The third sorting pathway, AGO2, a protein associated with the RNA-induced silencing complex (RISC) responsible for RNA silencing, is believed to regulate the loading of miRNAs into EVs [36]. Following the intracellular loading of miRNAs into EVs and their binding to the cell membrane region, along with plasma membrane fusion, the ultimate release of EVs containing miRNAs occurs. Up to now, it has been suggested that cells internalize EVs either through fusion with the plasma membrane or through the process of endocytosis [37]. Endocytic uptake can be classified into various types of endocytotic processes, such as clathrin- or caveolin-mediated endocytosis, lipid raft-mediated endocytosis, macropinocytosis, and phagocytosis [38].
Macrophages/microglia activation in acute lung injury and ischemic stroke
Macrophage activation after acute lung injury
Recent research indicates that a substantial portion of tissue macrophages is sustained without reliance on blood monocytes. Instead, they originate prenatally from primitive macrophages present within the yolk sac or fetal liver [39, 40]. Macrophages in lung tissue can be classified into two main subpopulations: alveolar macrophages located in the airway lumen, characterized by surface markers CD11blow CD11c++ CD169+, and parenchymal interstitial macrophages, identified by CD11b+ CD11clow CD169−. Alveolar macrophages, more prevalent than their interstitial counterparts, serve as the initial defense against foreign invaders, playing a crucial role in host defense and maintaining immune homeostasis in the local microenvironment of lung tissue [41, 42]. Macrophages are versatile cells with the capacity to transition through a spectrum of phenotypes and activation states, primarily influenced by the surrounding microenvironment. Broadly speaking, two primary macrophage phenotypes have been proposed: classically activated or pro-inflammatory (M1) macrophages and alternatively activated or anti-inflammatory (M2) macrophages [43]. In terms of phenotype, cytokines primarily released by Th1 cells, such as IFN-γ and TNF-α, along with lipopolysaccharide and granulocyte-macrophage colony-stimulating factor, are potent inducers that promote the polarization of macrophages into the M1 phenotype. In addition, M1 macrophages display heightened expression levels of CD16, CD32, CD40, CD64, CD68, CD80, CD86, MHCII, TLR4, and iNOS. They also produce increased quantities of pro-inflammatory Th1 cytokines (e.g. IL-6, IL-12, IL-1β, and TNF-α), monocyte chemotactic protein 1, macrophage inflammatory protein 2, cyclooxygenase 2, and chemokines (e.g. CCL2, CCL5, CCL8) [44, 45]. These elements play a central role in the clearance of intracellular pathogens and the recruitment and activation of T and B cells. On the contrary, M2 macrophages, also known as ‘selectively activated macrophages’, arise in response to macrophage colony-stimulating factors, immune complexes, Th2 cytokines such as IL-4 and IL-13, as well as anti-inflammatory cytokines like IL-10 and TGF-β [46, 47]. M2 macrophages primarily showcase expression of CD163, CD206, CD64, and CD209 and release anti-inflammatory cytokines, including IL-8, IL-10, and IL-13 as well as chemokines such as CCL1-4, CCL13-14, and CCL22-24 [47–49]. This expression profile contributes to their anti-inflammatory effects, support for tissue remodeling, facilitation of tumor development, and elimination of parasites. Depending on distinct microenvironments and stimuli, M2 macrophages can be categorized into four subtypes: M2a, M2b, M2c, and M2d [50]. M2a macrophages arise from non-polarized macrophages upon stimulation with IL-4 and IL-13, associating with allergic reactions [50, 51]. The M2b subset, distinguished by high expression of CD14 and CD80, can be activated by either LPS or IL-1β, resulting in the production of inflammatory cytokines [50]. M2c macrophages, induced by IL-10, TGF-β, and glucocorticoids, play a crucial role in suppressing immune responses and facilitating tissue repair [52, 53]. M2d macrophages, also known as tumor-associated macrophages, are triggered by the adenosine A2 receptor, leukemia inhibitory factor, and IL-6. Their primary function involves dampening inflammatory reactions while promoting angiogenesis and supporting tumor growth [54, 55].
Microglia activation after ischemic brain injury
Microglia represent specialized macrophages exclusive to the central nervous system. Microglia arise from primitive myeloid progenitor cells that exit the yolk sac around 8.5–9 days of embryonic development [56]. Subsequently, they migrate into the neural tube through primitive blood flow to the central nervous system. During this process, they undergo genealogy-specific gene expression changes and mature into fully differentiated microglia [57]. Under normal conditions, microglia in a resting state usually exhibit a highly branched morphology, often referred to as the resting state [56]. Serving as integral components of the innate immune system, these microglia actively survey the surrounding environment, staying vigilant for indications of damage, which can encompass damage-associated molecular patterns. Upon detecting signals of ischemia in the central nervous system microenvironment, microglia undergo morphological changes, marked by cell body hypertrophy and the retraction of cell processes. Subsequently, they promptly activate specific genes essential for initiating an inflammatory process. This inflammatory response is orchestrated with the goal of containing the progression of damage and promoting repair. The activation of microglia was traditionally classified based on intracellular dynamics and protein production into two categories: classical pro-inflammatory activation (M1) and alternative anti-inflammatory activation (M2). M1-type microglia exhibit distinctive features, producing pro-inflammatory cytokines such as IL-1β, IL-6, IL-12, IL-17, IL-18, IL-23, and TNF-α [56]. They generate redox molecules like phagocytic oxidase and iNOS, express chemokines such as CCL2 and CXCL10, and generate substantial amounts of reactive oxygen species [58, 59]. These combined factors actively contribute to the stimulation of inflammatory responses and play a role in neurotoxicity. On the flip side, M2-type microglia liberate anti-inflammatory cytokines like TGF-β, IL-4, IL-10, IL-13, and growth factors such as vascular endothelial growth factor, brain-derived neurotrophic factor, insulin-like growth factor, and platelet-derived growth factor [60, 61]. This function is geared towards mitigating inflammation and promoting tissue recovery. The M2 phenotypes are subdivided into three states: M2a, M2b, and M2c. Despite sharing common biochemical functions, these states vary regarding the stimuli that activate them, marker expression, and mechanisms of action. M2a microglia demonstrate a robust association with IL-13 and IL-4, exerting potent anti-inflammatory effects. These cells also exhibit substantial production of arginase-1, Ym-1, CD206, Fizz1, and different scavenger receptors [62, 63]. In contrast, the M2b phenotype, activated by the fusion of TLR and FCγ receptors, lacks the capacity to generate the latter [63, 64]. The M2c phenotype, also recognized as deactivated microglia, is linked to the promotion of tissue regeneration during later stages of disease progression when inflammation is diminishing [65, 66]. In animal models of ischemic stroke, the activation of microglia dynamically fluctuates over time and space during distinct stages, showing a correlation with the severity of the ischemic stroke [67]. Following a stroke, M1-type microglia are predominantly concentrated in the penumbra within the first 24 hours, and there is a noteworthy increase in the level of its marker CD68 starting from 24 hours post middle cerebral artery occlusion. Subsequently, later point, M1-type microglia can be observed in both the penumbra and the ischemic center [68]. In the early stages of stroke, the presence of M2-type microglia is confined to the ischemic core area, peaking around 3–5 days post middle cerebral artery occlusion. As time progresses, this expression diminishes, transitioning into M1-type microglia within the infarct margin area. Between days 14 and 28, the abundance of M2-type microglia has returned to its pre-injury baseline [69, 70]. In contrast, M1-type microglia continue to show a sustained increase over the 14-day period following middle cerebral artery occlusion. Moreover, Fig. 1 illustrates the polarization process between the M1 and M2 phenotypes of macrophage/microglia, highlighting the distinct signaling pathways and functional roles associated with each phenotype.
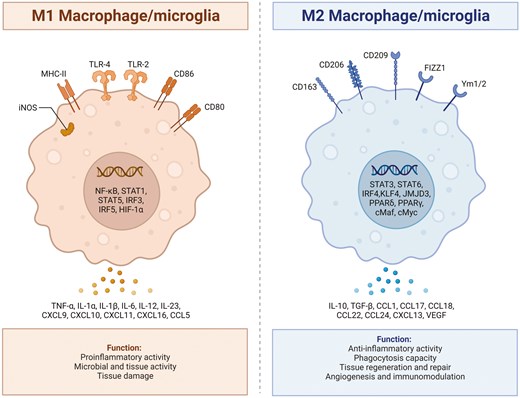
: illustrates the polarization process between the M1 and M2 phenotypes of macrophage/microglia, highlighting the distinct signaling pathways and functional roles associated with each phenotype. The polarization of macrophage/microglia into the M1 or M2 phenotype is a dynamic process influenced by the surrounding microenvironment and specific signaling molecules. Microglia that adopt the M1 phenotype are typically activated in response to proinflammatory stimuli such as interferon-gamma or lipopolysaccharide. On the other hand, macrophage/microglia that polarize towards the M2 phenotype are typically induced by anti-inflammatory signals such as interleukin-4 or interleukin-13. Created with Biorender.com
The potential differences between macrophage and microglia
While macrophages and microglia share many functional similarities as immune cells involved in inflammation and tissue repair, they also exhibit distinct differences in origin, function, and behavior that are important to clarify. Macrophages are derived from circulating monocytes, which originate in the bone marrow and migrate to various tissues throughout the body, where they differentiate into macrophages [71, 72]. These cells are highly adaptable and can be recruited to sites of injury or infection anywhere in the body, differentiating into various phenotypes, such as the pro-inflammatory M1 or the anti-inflammatory M2, depending on environmental signals [71–73]. This plasticity allows them to participate in a wide range of immune responses across different tissues. In contrast, microglia are resident immune cells of the central nervous system (CNS), originating from yolk sac progenitors during early development [74]. They remain in the CNS throughout life, providing critical immune surveillance and responding to CNS-specific injuries or diseases. Microglia have a more restricted range of functions compared to macrophages, being involved in maintaining homeostasis, pruning synapses, and responding to CNS-specific conditions [75, 76]. Upon activation, microglia can also polarize into M1-like or M2-like states, but their response is finely tuned to the unique environment of the CNS, including the presence of neurons and the blood-brain barrier [75]. Additionally, macrophages secrete a broad range of cytokines, chemokines, and growth factors that influence immune responses throughout the body, with their secretion profile being influenced by the tissue environment and signals they receive [77]. Microglia, while also capable of secreting cytokines and other factors, have a secretion profile specifically adapted to the CNS [77]. For example, they are involved in the production of neurotrophic factors that support neuron survival and function, a role less common in peripheral macrophages [77]. Moreover, macrophages are exposed to a wide variety of signals from different tissues and pathogens, leading to diverse responses depending on the context [78, 79]. Microglia, however, are more sensitive to subtle changes in the CNS environment, such as fluctuations in neurotransmitter levels or the presence of misfolded proteins, which is crucial for their role in CNS-specific diseases like neurodegenerative disorders [78–80]. These distinctions between macrophages and microglia reflect their specialization for roles in different parts of the body and are important to consider when evaluating their roles in diseases and therapeutic strategies, particularly in the context of EV and miRNA research, where the source and behavior of these cells can significantly influence outcomes.
The emerging role of EV-miRNAs from macrophage in acute lung injury
Critical pulmonary conditions, such as acute lung injury, present a substantial danger to critically ill patients, affecting around 200 000 individuals annually in the USA [81–83]. This condition comprises approximately 10% of all patients admitted to intensive care units and is linked to a considerable mortality rate, reaching around 40% [84, 85]. Acute lung injury manifests as a consequence of the pathophysiological response to a harmful trigger, leading to compromised pulmonary gas exchange attributed to widespread damage in the alveoli. The absence of prognostic biomarkers has impeded detection methods and the development of effective therapies for acute lung injury. Utilizing quantitative miRNA-based approaches in systemic circulation has been suggested as a way to improve diagnostic strategies and facilitate pharmacotherapy in cases of acute lung injury. It has been elucidated that several miRNAs, specifically miR-34a [86], miR-300 [87], miR-132 [88], miR-155 [89], miR-887-3p [90], miR-34a-5p [91], and miR-1246 [92], exhibit decreased levels in acute lung injury conditions. Conversely, others, such as miR-27a [93], miR-16 [94], miR-182 [95], miR-145-5p [96], and miR-140 [97], show increased expression at defined time points. The intricate balance between protective and adverse effects following acute lung injury is governed by a precisely orchestrated process regulated by various factors. These factors include but are not limited to, TLR4, FOXO3, Ang1, IκBα, FOXO3, SOCS1, SIRT1, and ACE2. Furthermore, with regard to EVs, it has been clarified that EVs originating from various stem cells, such as human adipose mesenchymal stem cells, human umbilical cord mesenchymal stem cells, and bone marrow mesenchymal stem cells, exhibit promise in the treatment of acute lung injury [98]. This potential is attributed to their favorable biological characteristics, ease of cultivation, low immunogenicity, and abundant sources.
In discussions surrounding EV-derived miRNAs from macrophages, a growing body of literature underscores their significance in regulating the prognosis of acute lung injury. A comprehensive search strategy was employed to identify relevant studies on the impact of macrophage-derived EVs, including macrophage-derived exosomes, in the treatment of acute lung injury. Databases such as PubMed, the Cochrane Library (last accessed in Nov 2023), and pertinent platforms like Web of Science and EMBASE (covering the period from 1990 to Nov 2023) were queried. The search utilized keywords such as ‘lung injury’, ‘extracellular vesicles’, or ‘exosomes’, in conjunction with ‘macrophage’ or ‘microRNA’, employing Boolean logic. This process adopted the Preferred Reporting Items for Systematic Reviews and Meta-Analyses (PRISMA) statement as guidelines. Additionally, a manual check of the reference lists in the identified studies was conducted to identify other potential qualifying studies. This iterative process was repeated until no further publications could be obtained. In this section, a total of six publications were discovered [22, 99–102], originating from Belgium, Italy, and China, and conducted between 2020 and 2023. EVs carrying miRNAs from macrophages in different states have been identified, and these miRNAs, such as miR-1249-5p [99], miR-15a-5p22, miR-29a-3p22, miR-143-3p22, miR-122-5p22, miR-370 [100], miR-7219–3p [101], miR-155-5p [102], and miR-142-3p [103], exhibit either promotive or inhibitory effects on the prognosis of acute lung injury, as outlined in Table 1.
preclinical studies assessing the effects of microRNA transferred via EVs-derived from macrophage in lung injury
Author, year . | miRNA . | Expression . | Purification . | Macrophage type . | Function . | Target . |
---|---|---|---|---|---|---|
Zhu et al. 2022 [99] | miR-1249-5p | Downregulation | Ultracentrifuge | Cell line RAW264.7 | Regulate influenza A virus-induced acute lung injury in RAW246.7 cells. | SLC4A1 and NF-κB |
Albano et al. 2023 [22] | miR-15a-5p, miR-29a-3p, miR-143-3p, miR-122-5p | Downregulation: miR-122-5p Upregulation: miR-15a-5p, 29a-3p, 143-3p | Ultracentrifuge | PBDE-47 and LPS | Modulate the expression of markers of epithelial integrity, epithelial mesenchymal transition, inflammation, and muco-secretion. | Not report |
Li et al. 2021 [100] | miR-370 | Downregulation | Ultracentrifuge | M2 macrophage | Alleviate asthma progression in asthma-like mouse and cell models. | FGF1/MAPK/STAT1 |
Niu et al. 2022 [101] | miR-7219–3p | Upregulation | Ultracentrifuge | Cell line RAW264.7 | Mediate fibroblast trans-differentiation in silicosis. | SPRY1 |
Xu et al. 2023 [102] | miR-155-5p | Upregulation | Purification kit | Cell line RAW264.7 | Widespread macrophage M1 polarization in hypervirulent Klebsiella pneumoniae-induced acute lung injury | MSK1/p38-MAPK |
Guiot et al. 2020 [103] | miR-142-3p | Upregulation | Ultracentrifuge | Cell line RAW264.7 | Attenuate fibrosis in airway epithelial cells through delivery of antifibrotic miR-142-3p | TGFβ-R1 |
Author, year . | miRNA . | Expression . | Purification . | Macrophage type . | Function . | Target . |
---|---|---|---|---|---|---|
Zhu et al. 2022 [99] | miR-1249-5p | Downregulation | Ultracentrifuge | Cell line RAW264.7 | Regulate influenza A virus-induced acute lung injury in RAW246.7 cells. | SLC4A1 and NF-κB |
Albano et al. 2023 [22] | miR-15a-5p, miR-29a-3p, miR-143-3p, miR-122-5p | Downregulation: miR-122-5p Upregulation: miR-15a-5p, 29a-3p, 143-3p | Ultracentrifuge | PBDE-47 and LPS | Modulate the expression of markers of epithelial integrity, epithelial mesenchymal transition, inflammation, and muco-secretion. | Not report |
Li et al. 2021 [100] | miR-370 | Downregulation | Ultracentrifuge | M2 macrophage | Alleviate asthma progression in asthma-like mouse and cell models. | FGF1/MAPK/STAT1 |
Niu et al. 2022 [101] | miR-7219–3p | Upregulation | Ultracentrifuge | Cell line RAW264.7 | Mediate fibroblast trans-differentiation in silicosis. | SPRY1 |
Xu et al. 2023 [102] | miR-155-5p | Upregulation | Purification kit | Cell line RAW264.7 | Widespread macrophage M1 polarization in hypervirulent Klebsiella pneumoniae-induced acute lung injury | MSK1/p38-MAPK |
Guiot et al. 2020 [103] | miR-142-3p | Upregulation | Ultracentrifuge | Cell line RAW264.7 | Attenuate fibrosis in airway epithelial cells through delivery of antifibrotic miR-142-3p | TGFβ-R1 |
FGF1: Fibroblast Growth Factor 1; MAPK: Mitogen-Activated Protein Kinase; miR: microRNA; MSK1: Mitogen- and Stress-Activated Protein Kinase 1; NF-κB: Nuclear Factor kappa B; SLC4A1: Solute Carrier Family 4 Member 1; SPRY1: Sprouty 1; STAT1: Signal Transducer and Activator of Transcription 1; TGFβ-R1: Transforming Growth Factor Beta Receptor 1.
preclinical studies assessing the effects of microRNA transferred via EVs-derived from macrophage in lung injury
Author, year . | miRNA . | Expression . | Purification . | Macrophage type . | Function . | Target . |
---|---|---|---|---|---|---|
Zhu et al. 2022 [99] | miR-1249-5p | Downregulation | Ultracentrifuge | Cell line RAW264.7 | Regulate influenza A virus-induced acute lung injury in RAW246.7 cells. | SLC4A1 and NF-κB |
Albano et al. 2023 [22] | miR-15a-5p, miR-29a-3p, miR-143-3p, miR-122-5p | Downregulation: miR-122-5p Upregulation: miR-15a-5p, 29a-3p, 143-3p | Ultracentrifuge | PBDE-47 and LPS | Modulate the expression of markers of epithelial integrity, epithelial mesenchymal transition, inflammation, and muco-secretion. | Not report |
Li et al. 2021 [100] | miR-370 | Downregulation | Ultracentrifuge | M2 macrophage | Alleviate asthma progression in asthma-like mouse and cell models. | FGF1/MAPK/STAT1 |
Niu et al. 2022 [101] | miR-7219–3p | Upregulation | Ultracentrifuge | Cell line RAW264.7 | Mediate fibroblast trans-differentiation in silicosis. | SPRY1 |
Xu et al. 2023 [102] | miR-155-5p | Upregulation | Purification kit | Cell line RAW264.7 | Widespread macrophage M1 polarization in hypervirulent Klebsiella pneumoniae-induced acute lung injury | MSK1/p38-MAPK |
Guiot et al. 2020 [103] | miR-142-3p | Upregulation | Ultracentrifuge | Cell line RAW264.7 | Attenuate fibrosis in airway epithelial cells through delivery of antifibrotic miR-142-3p | TGFβ-R1 |
Author, year . | miRNA . | Expression . | Purification . | Macrophage type . | Function . | Target . |
---|---|---|---|---|---|---|
Zhu et al. 2022 [99] | miR-1249-5p | Downregulation | Ultracentrifuge | Cell line RAW264.7 | Regulate influenza A virus-induced acute lung injury in RAW246.7 cells. | SLC4A1 and NF-κB |
Albano et al. 2023 [22] | miR-15a-5p, miR-29a-3p, miR-143-3p, miR-122-5p | Downregulation: miR-122-5p Upregulation: miR-15a-5p, 29a-3p, 143-3p | Ultracentrifuge | PBDE-47 and LPS | Modulate the expression of markers of epithelial integrity, epithelial mesenchymal transition, inflammation, and muco-secretion. | Not report |
Li et al. 2021 [100] | miR-370 | Downregulation | Ultracentrifuge | M2 macrophage | Alleviate asthma progression in asthma-like mouse and cell models. | FGF1/MAPK/STAT1 |
Niu et al. 2022 [101] | miR-7219–3p | Upregulation | Ultracentrifuge | Cell line RAW264.7 | Mediate fibroblast trans-differentiation in silicosis. | SPRY1 |
Xu et al. 2023 [102] | miR-155-5p | Upregulation | Purification kit | Cell line RAW264.7 | Widespread macrophage M1 polarization in hypervirulent Klebsiella pneumoniae-induced acute lung injury | MSK1/p38-MAPK |
Guiot et al. 2020 [103] | miR-142-3p | Upregulation | Ultracentrifuge | Cell line RAW264.7 | Attenuate fibrosis in airway epithelial cells through delivery of antifibrotic miR-142-3p | TGFβ-R1 |
FGF1: Fibroblast Growth Factor 1; MAPK: Mitogen-Activated Protein Kinase; miR: microRNA; MSK1: Mitogen- and Stress-Activated Protein Kinase 1; NF-κB: Nuclear Factor kappa B; SLC4A1: Solute Carrier Family 4 Member 1; SPRY1: Sprouty 1; STAT1: Signal Transducer and Activator of Transcription 1; TGFβ-R1: Transforming Growth Factor Beta Receptor 1.
In a lung injury associated with an asthma mouse model induced by ovalbumin, EVs derived from M2 macrophages markedly mitigated ovalbumin-induced fibrosis and inflammatory responses in the lung tissues of mice [100]. Additionally, they inhibited abnormal proliferation, invasion, and the production of fibrosis-related proteins in primary mouse airway smooth muscle cells treated with platelet-derived growth factor [100]. The administration of ovalbumin in mice or the treatment of airway smooth muscle cells with platelet-derived growth factor-BB led to a decrease in the expression of miR-370. Interestingly, the introduction of M2 macrophage-derived EVs reversed the inhibitory effect on miR-370 expression in both vivo and vitro. Conversely, the downregulation of miR-370 by Lv-miR-370 inhibitor counteracted the protective effects of M2 macrophage-derived EVs in asthma-like mouse and cell models. Collectively, miR-370 was involved in the therapeutic effects of M2 macrophage-derived EVs by alleviating asthma progression [100]. In the context of lung injury associated with silicosis, Niu et al. [101] demonstrated that exposure to silica stimulates macrophages to release an increased quantity of EVs. These vesicles play a crucial role in fibroblast-to-myofibroblast transition, and this impact is, at least in part, mediated by miR-7219-3p carried within EVs. The authors additionally observed that these effects were attained by inhibiting SPRY1 and activating ERK/MAPK phosphorylation. Herein, this phenomenon could be reversed after treatment with a miR-7219-3p inhibitor and antagomir. In a lung injury associated with fibrosis, Guiot et al. [103] showed that EVs derived from macrophages alleviate fibrosis in airway epithelial cells by delivering the antifibrotic miR-142-3p. miRNAs within EVs originating from various cells can also modulate macrophage polarization and contribute to the advancement of lung injury. For example, in a lung injury induced by influenza A virus, Zhu et al. [99] indicated that miR-1249-5p derived from mouse lung epithelial cell-EVs modulates acute lung injury in macrophages by targeting SLC4A1. In addition, macrophages under stimulation can autonomously influence their polarization by releasing EV-derived miRNAs. As indicated by Xu et al. [102], they revealed that EVs miR-155-5p originating from macrophages activated by hypervirulent Klebsiella pneumoniae can impact the M1 polarization and inflammatory response of quiescent macrophages. This occurs by activating the p38-MAPK signaling pathway, thereby influencing lung inflammation and tissue damage.
The emerging role of EV-miRNAs derived from microglia in ischemic stroke
Numerous investigators exploring EV-miRNAs from macrophages have unveiled a fresh perspective on the interplay in acute lung injury, as previously mentioned. Additionally, the pivotal involvement of miRNAs encapsulated in EVs in ischemic stroke has been acknowledged. In the initial phase, a specific group of miRNAs, including miR-22, miR-26a, miR-124, miR-146a, miR-181c, miR-183, miR-203, miR-210, and miR-424, exhibit an elevation following ischemic stroke [104]. Conversely, others such as miR-130a, miR-150, miR-155, miR-182, and miR-320 are diminished during this early stage [104]. Meanwhile, EVs possess the capability to be internalized by adjacent or distant cells due to their diverse targets. These EVs modulate gene expression through posttranscriptional regulation, with the miRNAs they carry serving as potent factors for repair. A growing body of evidence indicates that miRNAs are preferentially concentrated in EVs, exerting biological functions by modulating specific facets of ischemic stroke. Consequently, they play a role in cell death, apoptosis, and neuroinflammation [20, 21, 105–111], as outlined in Table 2 and depicted in Figure 2. These aspects are further explored in the subsequent sections.
preclinical studies assessing the effects of microRNA transferred via EVs-derived from microglia in stroke
Author, year . | miRNA . | Expression . | Purification . | Microglial status . | Function . | Target . |
---|---|---|---|---|---|---|
Li et al. 2021 [20] | miR-124 | Upregulation | Ultracentrifugation | M2-BV2 | Reduce glial scar formation and promote ischemic stroke recovery. | STAT3 |
Li et al. 2022 [21] | miR-23a-5p | Upregulation | Ultracentrifugation | M2-BV2 | Promote white matter repair and functional ischemic stroke recovery. | Olig3 |
Li et al. 2023 [105] | miR-212-5p | Upregulation | Ultracentrifugation | Microglia | Promote synaptic plasticity and attenuate axonal degeneration. | PlexinA2 |
Song et al. 2019 [106] | miR-124 | Upregulation | Ultracentrifugation | M2-BV2 | Attenuate ischemic brain injury and promote neuronal survival. | USP14 |
Song et al. 2023 [107] | miR-124 | Upregulation | Ultracentrifugation | M2-BV2 | Enhance neural stem cell proliferation and differentiation. | AAK1/Notch |
Wang et al. 2023 [108] | miR-9-5p | Downregulation | Ultracentrifugation | BV2 | Attenuate LPS-induced microglia cell inflammation. | NO, IL-6, TNF-α |
Wei et al. 2021 [109] | miR-383-3p | Upregulation | Ultracentrifugation | M2 microglia | Promote necroptosis of neurons in intracerebral hemorrhage rats. | ATF4 |
Xie et al. 2020 [110] | miR‑424-5p | Upregulation | Ultracentrifugation | OGD-treated microglia | Aggravate BBB destruction and neurological damage. | FGF2/STAT3 |
Zhang et al. 2022 [111] | miR-137 | Upregulation | Ultracentrifugation | M2-BV2 | Alleviate neuronal apoptosis and ischemia-reperfusion brain injury. | Notch1 |
Author, year . | miRNA . | Expression . | Purification . | Microglial status . | Function . | Target . |
---|---|---|---|---|---|---|
Li et al. 2021 [20] | miR-124 | Upregulation | Ultracentrifugation | M2-BV2 | Reduce glial scar formation and promote ischemic stroke recovery. | STAT3 |
Li et al. 2022 [21] | miR-23a-5p | Upregulation | Ultracentrifugation | M2-BV2 | Promote white matter repair and functional ischemic stroke recovery. | Olig3 |
Li et al. 2023 [105] | miR-212-5p | Upregulation | Ultracentrifugation | Microglia | Promote synaptic plasticity and attenuate axonal degeneration. | PlexinA2 |
Song et al. 2019 [106] | miR-124 | Upregulation | Ultracentrifugation | M2-BV2 | Attenuate ischemic brain injury and promote neuronal survival. | USP14 |
Song et al. 2023 [107] | miR-124 | Upregulation | Ultracentrifugation | M2-BV2 | Enhance neural stem cell proliferation and differentiation. | AAK1/Notch |
Wang et al. 2023 [108] | miR-9-5p | Downregulation | Ultracentrifugation | BV2 | Attenuate LPS-induced microglia cell inflammation. | NO, IL-6, TNF-α |
Wei et al. 2021 [109] | miR-383-3p | Upregulation | Ultracentrifugation | M2 microglia | Promote necroptosis of neurons in intracerebral hemorrhage rats. | ATF4 |
Xie et al. 2020 [110] | miR‑424-5p | Upregulation | Ultracentrifugation | OGD-treated microglia | Aggravate BBB destruction and neurological damage. | FGF2/STAT3 |
Zhang et al. 2022 [111] | miR-137 | Upregulation | Ultracentrifugation | M2-BV2 | Alleviate neuronal apoptosis and ischemia-reperfusion brain injury. | Notch1 |
AAK1: adaptor-associated protein kinase 1; BBB: blood-brain barrier; miR: microRNAs; OGD: oxygen-glucose deprivation; USP14: ubiquitin-specific protease 14.
preclinical studies assessing the effects of microRNA transferred via EVs-derived from microglia in stroke
Author, year . | miRNA . | Expression . | Purification . | Microglial status . | Function . | Target . |
---|---|---|---|---|---|---|
Li et al. 2021 [20] | miR-124 | Upregulation | Ultracentrifugation | M2-BV2 | Reduce glial scar formation and promote ischemic stroke recovery. | STAT3 |
Li et al. 2022 [21] | miR-23a-5p | Upregulation | Ultracentrifugation | M2-BV2 | Promote white matter repair and functional ischemic stroke recovery. | Olig3 |
Li et al. 2023 [105] | miR-212-5p | Upregulation | Ultracentrifugation | Microglia | Promote synaptic plasticity and attenuate axonal degeneration. | PlexinA2 |
Song et al. 2019 [106] | miR-124 | Upregulation | Ultracentrifugation | M2-BV2 | Attenuate ischemic brain injury and promote neuronal survival. | USP14 |
Song et al. 2023 [107] | miR-124 | Upregulation | Ultracentrifugation | M2-BV2 | Enhance neural stem cell proliferation and differentiation. | AAK1/Notch |
Wang et al. 2023 [108] | miR-9-5p | Downregulation | Ultracentrifugation | BV2 | Attenuate LPS-induced microglia cell inflammation. | NO, IL-6, TNF-α |
Wei et al. 2021 [109] | miR-383-3p | Upregulation | Ultracentrifugation | M2 microglia | Promote necroptosis of neurons in intracerebral hemorrhage rats. | ATF4 |
Xie et al. 2020 [110] | miR‑424-5p | Upregulation | Ultracentrifugation | OGD-treated microglia | Aggravate BBB destruction and neurological damage. | FGF2/STAT3 |
Zhang et al. 2022 [111] | miR-137 | Upregulation | Ultracentrifugation | M2-BV2 | Alleviate neuronal apoptosis and ischemia-reperfusion brain injury. | Notch1 |
Author, year . | miRNA . | Expression . | Purification . | Microglial status . | Function . | Target . |
---|---|---|---|---|---|---|
Li et al. 2021 [20] | miR-124 | Upregulation | Ultracentrifugation | M2-BV2 | Reduce glial scar formation and promote ischemic stroke recovery. | STAT3 |
Li et al. 2022 [21] | miR-23a-5p | Upregulation | Ultracentrifugation | M2-BV2 | Promote white matter repair and functional ischemic stroke recovery. | Olig3 |
Li et al. 2023 [105] | miR-212-5p | Upregulation | Ultracentrifugation | Microglia | Promote synaptic plasticity and attenuate axonal degeneration. | PlexinA2 |
Song et al. 2019 [106] | miR-124 | Upregulation | Ultracentrifugation | M2-BV2 | Attenuate ischemic brain injury and promote neuronal survival. | USP14 |
Song et al. 2023 [107] | miR-124 | Upregulation | Ultracentrifugation | M2-BV2 | Enhance neural stem cell proliferation and differentiation. | AAK1/Notch |
Wang et al. 2023 [108] | miR-9-5p | Downregulation | Ultracentrifugation | BV2 | Attenuate LPS-induced microglia cell inflammation. | NO, IL-6, TNF-α |
Wei et al. 2021 [109] | miR-383-3p | Upregulation | Ultracentrifugation | M2 microglia | Promote necroptosis of neurons in intracerebral hemorrhage rats. | ATF4 |
Xie et al. 2020 [110] | miR‑424-5p | Upregulation | Ultracentrifugation | OGD-treated microglia | Aggravate BBB destruction and neurological damage. | FGF2/STAT3 |
Zhang et al. 2022 [111] | miR-137 | Upregulation | Ultracentrifugation | M2-BV2 | Alleviate neuronal apoptosis and ischemia-reperfusion brain injury. | Notch1 |
AAK1: adaptor-associated protein kinase 1; BBB: blood-brain barrier; miR: microRNAs; OGD: oxygen-glucose deprivation; USP14: ubiquitin-specific protease 14.
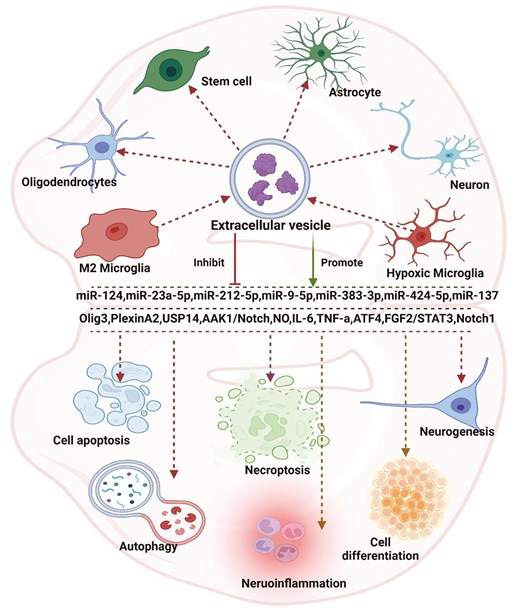
: examining the impact of microRNA transferred through extracellular vesicles released by microglia in ischemic stroke reveals a broad range of effects. Originating predominantly from microglial cells in the extracellular space, these vesicles primarily influence processes such as cell differentiation, autophagy, apoptosis, necroptosis, regeneration, and inflammation through diverse pathways. These vesicles can interact with multiple cell types, including neurons, astrocytes, stem cells, and oligodendrocytes. MicroRNAs assume crucial roles in various pathways within this context. Created with Biorender.com
The occurrence of cell death in neurological diseases results from a variety of cellular processes, encompassing mitochondrial dysfunction, protein aggregation, the generation of free radicals, excitotoxicity, and inflammation [112]. Numerous studies have revealed that EV-miRNAs derived from microglia are involved in cell death regulation. For example, as indicated by Zhang et al. [111], in the LDH assay, it was observed that a microglia-conditioned medium led to a decrease in LDH activity, signifying reduced cellular injury in neurons following OGD treatment. Interestingly, Zhang et al. [111] noted that the introduction of GW4869, an inhibitor of EV secretion, partially reversed the impact of the microglia-conditioned medium on OGD-treated neurons. These findings collectively suggest the potential involvement of microglia-derived EVs in a neuroprotective mechanism against OGD. Furthermore, LDH assays revealed that neurons, when cocultured with microglia-derived EVs for 24 hours followed by OGD, displayed a significant enhancement in cell viability. Importantly, it was observed that miR-137 was associated with the decreased infarct volume and behavioral deficits in ischemic mice mediated by microglia-derived EVs. As such, Li et al. [105] indicated that focal ischemic stroke alters EV-miR-212-5p derived from microglia, with a potential implication of miR-212-5p in promoting neuronal survival and contributing to functional recovery. Furthermore, the miRNAs in these EVs can also promote the survival rates of oligodendrocytes. As revealed by Li et al. [21], M2-type microglia-EVs were found to enhance oligodendrogenesis in a model of MCAO, promoting the proliferation, survival, and differentiation of oligodendrocyte precursor cells in vitro OGD model. MiR-23a-5p was identified as an enriched component in these EVs, exerting a positive influence on the proliferation, survival, and maturation of oligodendrocyte precursor cells. Knocking down miR-23a-5p in these EVs reversed the beneficial effects observed both in vitro and in vivo. To facilitate the restoration of neurological function following a stroke, the miRNAs contained within these EVs can additionally impede the proliferation of specific cells, such as astrocytes [106]. EVs derived from M2 microglia attenuated the formation of glial scars and facilitated post-stroke recovery. These vesicles were notably enriched in miR-124. Additionally, treatment with M2 microglial EVs resulted in reduced expression of the astrocyte proliferation gene Signal Transducer and Activator of Transcription 3, a target of miR-124. This treatment also led to decreased levels of glial fibrillary acidic protein, inhibiting astrocyte proliferation both in vitro and in vivo. Knocking down miR-124 in such EVs hindered their impact on glial scar formation and impaired the recovery process after a stroke [106]. It is worth noting that not all the miRNAs in these EVs are neuroprotective by regulating cell survival. Xie et al. [110] showed that EVs derived from OGD-activated microglia exacerbated OGD-induced damage to brain microvascular endothelial cells, including reduced viability and integrity, as well as loss of vascular formation. However, these detrimental effects were significantly mitigated by inhibiting miR-424-5p. Furthermore, the overexpression of miR-424-5p notably intensified OGD-induced damage and increased permeability in brain microvascular endothelial cells.
Among the diverse pathways leading to programmed cell death [113, 114], apoptosis constitutes a significant portion of cell demise associated with brain injury [114]. This process efficiently eliminates damaged cells resulting from DNA damage or during developmental stages [115]. Apoptosis is crucial for maintaining the homeostasis of normal tissues, and researchers have identified that EV-miRNAs from microglia play essential roles in the regulation of cellular apoptosis. As indicated by Zhang et al. [111] utilizing the TUNEL assay to identify apoptotic changes in neurons subjected to control conditions, OGD, OGD with M2-phenotype microglia EVs, mitigation of neuronal apoptosis induced by OGD was observed. Notably, RNA-seq analysis revealed an upregulation of miR-137 in EVs derived from M2-phenotype microglia. To elucidate the role of miR-137, it was found that the expression of cleaved caspase-3 significantly decreased in neurons treated with such EVs following OGD. This effect was partially reversed when neurons were treated with EVs derived from microglia subjected to a miR-137 inhibitor. Additionally, TUNEL assay results demonstrated that EVs with reduced miR-137 expression partially counteracted the pro-apoptotic impact of these EVs after OGD. In addition to apoptosis, the regulation of EV-miRNAs from microglia on necroptosis has been reported [109]. Programmed cell death takes on different characteristics in the forms of apoptosis and necroptosis. Necroptosis is orchestrated by the receptor-interacting proteins RIP1/RIP3 and the substrate of RIP3/MLKL [116]. These proteins collaborate to form a necrosis signaling complex, actively participating in the process of necroptosis [116]. Wei et al. demonstrated that the EVs derived from activated microglia play a mediating role in promoting neuronal necroptosis through the inhibition of ATF4 expression via miR-383-3p in stroke [109]. This also indicates that EV-miRNAs in microglial cells are not all protective.
The inflammatory response presents a dual role following ischemia, acting as a double-edged sword by exacerbating secondary brain injury while also fostering the recovery of neurological function. This dual effect highlights the association between inflammation, pathogenesis, and the prognosis of ischemia [117]. Numerous studies have demonstrated the close association of EV-miRNAs from microglial cells with the regulation of inflammation in the context of ischemia. The research revealed that Xiaoxuming decoction cutting possesses anti-neuroinflammatory properties [108]. Small RNA-seq analysis of EVs identified miR-9-5p as a crucial miRNA in Xiaoxuming decoction cutting’s mechanism for addressing neuroinflammation. Inhibition of miR-9-5p was observed to decrease the expression of inflammatory factors, including IL-1β, IL-6, iNOS, and TNF-α. Consequently, Xiaoxuming decoction cutting modulates the inflammatory response by influencing the expression of miR-9-5p in EVs derived from microglia [108].
EV-miRNAs: emerging candidates as novel biomarkers and therapeutic targets
EV-mediated miRNAs as potential biomarkers
EVs serve as mediators of cell-to-cell communication, facilitating the transport and delivery of their cargo to target cells. The contents of these vesicles serve as a molecular bioprinter, reflecting the characteristics of their parent cells. Consequently, EVs found in blood and other biofluids hold promise as potential noninvasive biomarkers for the early diagnosis and prognosis of various diseases. EVs, acting as natural carriers of miRNAs, possess several noteworthy attributes. Effectively harnessing the delivery of these vesicles poses a considerable challenge, given the need to attain heightened stability, even at room temperature over extended periods, while simultaneously minimizing toxicity and mitigating off-target effects [118]. Furthermore, EVs are plentiful in various body fluids such as blood, urine, and cerebrospinal fluids, which not only enables straightforward isolation but also bestows non-invasive advantages. The changes in expression of numerous miRNAs subsequent to ischemic stroke or acute lung injury, proposed as potential biomarkers, emphasize the possibility of manipulating EV-derived miRNAs to elucidate the progression of both injury conditions. Under ischemic stroke conditions, it is already known that miR-15, miR-16, miR-17-5p, miR-30a, and miR-126 from plasma, serum, or peripheral leukocytes were significantly downregulated in ischemic stroke patients [119], and the upregulated miR-16, miR-15, miR-17-5p, miR-29b, and miR-132 derived from the plasma of ischemic stroke patients also served as diagnostic biomarkers [119]. Interestingly, EVs as delivery carriers for miRNAs have also been identified as biomarkers for diagnosis. miR-134, miR-21-5p, miR-30a-5p, miR-223, miR-9, and miR-124 related to EVs and originating from the plasma or serum of stroke patients show an upregulated expression. Conversely, miR-422a and miR-125b-2-3p exhibit downregulated expression in the same context [120–124]. Under acute lung injury, in a study by Parzibut et al. [125], the expression of plasma EV-miRNAs was compared between 8 patients with acute respiratory distress syndrome and 10 healthy subjects using small RNA sequencing analysis. Notably, among the differentially expressed miRNAs, namely miR-221-3p, miR-24-3p, miR-130a-3p, Let-7d-3p, miR-1273a, miR-98-3p, and miR-193a-5p, were demonstrated to effectively distinguish between acute respiratory distress syndrome and hemorrhagic shock through receiver operating characteristic curve analysis. Elevated levels of miR-146a, miR-126, miR-27a, and miR-155 were identified in serum EVs from individuals with severe community-acquired pneumonia-related acute lung injury compared to those in the control group [126]. Despite traditional markers being widely used for clinical diagnosis, low sensitivity, and specificity limitations exist. A combination of conventional markers with EV-miRNAs is illustrated to boost diagnostic accuracy [13].
Utilizing EVs as carriers for miRNA-based therapy
EV-miRNAs also emerge as promising targets for therapeutic interventions in the management of conditions such as ischemic stroke and acute lung injury. EVs serve as protective carriers for miRNA cargo, shielding them from endogenous RNases and facilitating their delivery to target cells [127]. Generally, miRNA-based therapy aims to achieve therapeutic objectives by either replenishing or inhibiting the sequences of target miRNAs. The essential stages of miRNA-based therapy encompass identifying optimal miRNA targets, deciphering miRNA chemistry, refining delivery methods, conducting preclinical testing, and progressing to clinical trials. Under ischemic stroke conditions, in the work of Cai et al. [128], the expression of miR-542-3p was upregulated in EVs derived from mesenchymal stem cells through the transfection of miR-542-3p mimics. Administration of EV-miR-542-3p increased the expression of miR-542-3p and provided protection against ischemia-induced damage in mice subjected to middle cerebral artery occlusion and HA1800 cells exposed to hypoxia. This protective effect was achieved through the reduction of inflammation. Under acute lung injury conditions, the primary approach to treating acute lung injury continues to be symptomatic, involving interventions such as mechanical ventilation, fluid management, corticosteroid supplementation, and the use of inhaled pulmonary vasodilators. While these treatments can alleviate patient symptoms, they do not address the root cause of the condition. There is evidence indicating that miRNAs derived from EVs of mesenchymal stem cells can engage in intercellular interactions with resident lung cells, suggesting potential therapeutic benefits for acute lung injury [129]. EV-associated miR-384-5p, originating from bone marrow-derived mesenchymal stem cells, has demonstrated the capacity to mitigate macrophage apoptosis and autophagy stress in rats with acute lung injury [130]. This effect is achieved by targeting Beclin-1 in alveolar macrophages, ultimately contributing to improved survival. Additionally, in another study, EV-miR-127-5p derived from bone marrow-derived mesenchymal stem cells has been observed to impede the formation of neutrophil extracellular traps in acute lung injury associated with sepsis by targeting CD64. More importantly, EVs commonly contain CD47, an integrin-associated transmembrane protein that acts as a ‘don’t eat me’ signal, offering protection against phagocytosis [131–134]. Research findings indicate that EVs with an overexpression of CD47 demonstrate an extended half-life in circulation when compared to EVs lacking CD47. This observation suggests that the presence of CD47 on EVs contributes to their increased stability and prolonged presence in the bloodstream.
Challenges and potential risks of using EVs in clinical settings
The article presents a compelling case for the use of EVs as diagnostic and therapeutic tools, particularly in the context of neuroinflammatory conditions. However, translating these promising findings into clinical practice involves several significant challenges and limitations that require careful consideration. One of the primary clinical challenges lies in the standardization and isolation techniques for EVs [135, 136]. The inherent heterogeneity of EVs, combined with the lack of universally accepted protocols for their isolation and characterization, complicates the ability to achieve consistent and reproducible results [135, 136]. This variability can significantly impact the purity, yield, and functionality of EV preparations, making it difficult to ensure consistency in clinical applications where it is paramount. Additionally, the scalability and manufacturing of EVs for clinical use present another substantial hurdle. Producing EVs in sufficient quantities under good manufacturing practice conditions is not only technically demanding but also costly, potentially limiting the accessibility, and widespread adoption of EV-based therapies [12]. The safety profile of EVs also remains an area of concern [12]. Although EVs hold promise as therapeutic agents, they can carry a variety of bioactive molecules, including miRNAs, proteins, and lipids, some of which could inadvertently induce off-target effects, such as promoting oncogenesis or unwanted immune responses [12]. Moreover, the long-term effects of EV administration are not well understood, raising concerns about potential adverse outcomes over time. Regulatory and ethical concerns further complicate the clinical translation of EVs. The regulatory landscape for EV-based therapies is complex and still evolving, with stringent requirements for demonstrating safety, efficacy, and consistency [137]. Navigating these regulatory hurdles could be time-consuming and may delay the clinical implementation of these technologies. Ethical issues also arise, particularly regarding the source of EVs, especially if they are derived from human stem cells or other tissues [137]. Ensuring ethical compliance in terms of donor consent, potential exploitation, and the use of human-derived materials is crucial for the responsible development of EV-based therapies. Furthermore, there are limitations in current research that must be addressed. Much of the existing knowledge about EVs and their therapeutic potential is derived from in vitro studies or animal models [16]. Translating these findings to human patients presents challenges due to differences in physiology and the complexity of human diseases, and the efficacy and safety observed in preclinical studies may not always translate directly to clinical success [16]. Additionally, variability in EV isolation techniques, experimental conditions, and even patient populations can lead to inconsistent results, making it difficult to draw definitive conclusions about their therapeutic potential [12, 16]. This variability underscores the need for standardized methods and large-scale clinical trials to validate the efficacy of EV-based therapies. In summary, while the potential of EVs as diagnostic and therapeutic tools is promising, there are significant clinical challenges and limitations that need to be addressed before these technologies can be widely adopted in clinical practice. Further research, standardized protocols, and rigorous clinical trials will be essential to overcome these challenges and fully realize the therapeutic potential of EVs.
Conclusion
EVs play a pivotal role in the progression of ischemic stroke and acute lung injury, with their cargo holding significant importance. Enclosed miRNAs within EVs contribute to biological functions by influencing various aspects thereby decisively impacting tissue progression. Nevertheless, there are substantial challenges to address before EV-associated miRNAs can be effectively utilized as clinical assays for such disorders. Further exploration is essential to devise techniques for generating pure and homogeneous EVs. Additionally, addressing variations in EVs secreted by diverse cells under different environmental or stimulatory conditions, and developing methods to prepare stable decoy EVs for disease treatment, are additional challenges that require careful consideration. Significant hurdles persist in the clinical translation of EVs, particularly regarding the large-scale isolation and preparation of EVs. Ensuring the reproducibility of EV preparations under specific culturing conditions is also a substantial challenge. Additionally, the development of potency assays is essential to guarantee that the activity characteristics of EVs remain consistent across different preparations before their administration to human patients [138, 139]. The nuclear RNA exosome complex stands out as one of the most versatile RNA-degradation mechanisms in eukaryotes [140]. Hence, it is highly valuable to showcase how the regulation of the nuclear RNA exosome complex can be influenced by modulating the levels of exosomal miRNAs [16]. The functionality of EV-miRNAs is predominantly studied through animal and cellular models; however, additional validation in clinical samples is imperative.
Abbreviations
- Ago2
argonaute-2
- circRNAs
circular RNAs
- CNS
central nervous system
- ESCRT
endosomal sorting complex required for transport
- EVs
extracellular vesicles
- lncRNAs
long noncoding RNAs
- MCAO
middle cerebral artery occlusion
- miRNAs
microRNAs
- MVBs
multivesicular bodies
- ncRNAs
non-coding RNAs
- OGD
oxygen-glucose deprivation
- pre-miRNA
precursor miRNA
- pri-miRNA
primary miRNA
- PRISMA
preferred reporting items for systematic reviews and meta-analyses
- RISC
RNA-induced silencing complex
Conflict of interest
None declared.
Data availability
The data that support the findings of this study are available from the corresponding authors upon reasonable request.
Author contributions
Xianbin Wang (Conceptualization, Formal analysis, Funding acquisition, Investigation, Methodology, Project administration, Resources, Software, Validation, Visualization, Writing—original draft, Writing—review & editing), Ting Wang (Data curation, Formal analysis, Funding acquisition, Investigation, Methodology, Project administration, Resources, Software, Writing—original draft, Writing—review & editing), Dong Zhu (Data curation, Formal analysis, Investigation, Software, Validation, Visualization, Writing—original draft), Jing Wang (Data curation, Funding acquisition, Methodology, Project administration, Software, Validation, Writing—original draft), and Weijie Han (Conceptualization, Data curation, Formal analysis, Funding acquisition, Investigation, Methodology, Project administration, Resources, Software, Supervision, Validation, Visualization, Writing—original draft, Writing—review & editing)
References
Author notes
Xianbin Wang and Ting Wang contribute equally to this work and are considered co-first authors.