-
PDF
- Split View
-
Views
-
Cite
Cite
Benjamin Grimmer, Adrienn Krauszman, Xudong Hu, Golam Kabir, Kim A Connelly, Mei Li, Jana Grune, Christian Madry, Brant E Isakson, Wolfgang M Kuebler, Pannexin 1: a novel regulator of acute hypoxic pulmonary vasoconstriction, Cardiovascular Research, Volume 118, Issue 11, July 2022, Pages 2535–2547, https://doi.org/10.1093/cvr/cvab326
- Share Icon Share
Abstract
Hypoxic pulmonary vasoconstriction (HPV) is a physiological response to alveolar hypoxia that diverts blood flow from poorly ventilated to better aerated lung areas to optimize ventilation-perfusion matching. Yet, the exact sensory and signalling mechanisms by which hypoxia triggers pulmonary vasoconstriction remain incompletely understood. Recently, ATP release via pannexin 1 (Panx1) and subsequent signalling via purinergic P2Y receptors has been identified as regulator of vasoconstriction in systemic arterioles. Here, we probed for the role of Panx1-mediated ATP release in HPV and chronic hypoxic pulmonary hypertension (PH).
Pharmacological inhibition of Panx1 by probenecid, spironolactone, the Panx1 specific inhibitory peptide (10Panx1), and genetic deletion of Panx1 specifically in smooth muscle attenuated HPV in isolated perfused mouse lungs. In pulmonary artery smooth muscle cells (PASMCs), both spironolactone and 10Panx1 attenuated the increase in intracellular Ca2+ concentration ([Ca2+]i) in response to hypoxia. Yet, genetic deletion of Panx1 in either endothelial or smooth muscle cells did not prevent the development of PH in mice. Unexpectedly, ATP release in response to hypoxia was not detectable in PASMC, and inhibition of purinergic receptors or ATP degradation by ATPase failed to attenuate HPV. Rather, transient receptor potential vanilloid 4 (TRPV4) antagonism and Panx1 inhibition inhibited the hypoxia-induced [Ca2+]i increase in PASMC in an additive manner, suggesting that Panx1 regulates [Ca2+]i independently of the ATP-P2Y-TRPV4 pathway. In line with this notion, Panx1 overexpression increased the [Ca2+]i response to hypoxia in HeLa cells.
In the present study, we identify Panx1 as novel regulator of HPV. Yet, the role of Panx1 in HPV was not attributable to ATP release and downstream signalling via P2Y receptors or TRPV4 activation, but relates to a role of Panx1 as direct or indirect modulator of the PASMC Ca2+ response to hypoxia. Panx1 did not affect the development of chronic hypoxic PH.
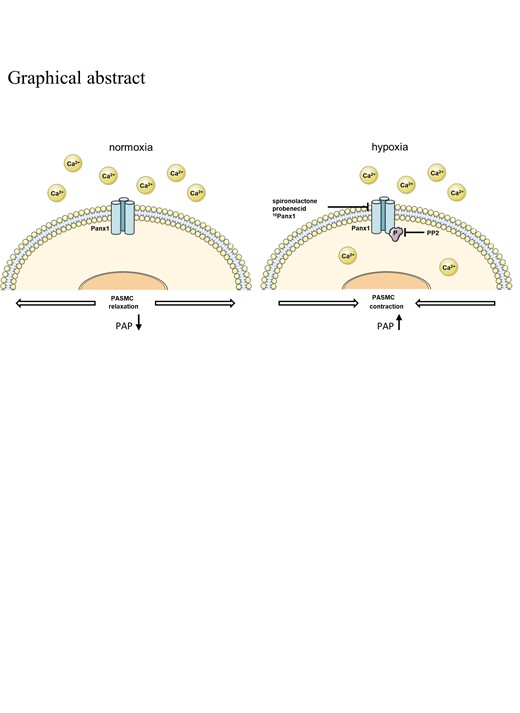
See the editorial comment for this article ‘Pannexin 1: a key regulator of intracellular calcium levels and hypoxic pulmonary vasoconstriction‘, by Jane A. Leopold, https://doi.org/10.1093/cvr/cva042.
1. Introduction
Hypoxic pulmonary vasoconstriction (HPV) was probably first described by Beutner1 as an increase in pulmonary artery pressure (PAP) in a cat during cessation of mechanical ventilation. In their 1946 hallmark paper, Ulf von Euler and Göran Liljestrand2 recognized the paramount physiological relevance of this response, as it facilitates redistribution of pulmonary blood flow from poorly ventilated, hypoxic lung areas to normoxic regions with better aeration in order to optimize ventilation/perfusion (V/Q) matching. Attenuated HPV, as seen in infectious diseases such as pneumonia or sepsis or in response to volatile anaesthetics causes V/Q mismatch and, as a consequence, hypoxaemia in critical ill patients.3,4 On the other hand, sustained HPV e.g. in global hypoxia at high altitude or chronic hypoxic lung diseases including chronic obstructive pulmonary disease, sleep apnoea, and lung fibrosis, causes lung vascular remodelling leading to the development of pulmonary hypertension (PH), right ventricular hypertrophy and, ultimately, global heart failure.5–7 Despite its clinical relevance, the molecular signalling pathways underlying HPV remain incompletely defined.
As putative mechanisms of oxygen sensing in HPV both changes in redox status via altered production of reactive oxygen species from mitochondria and/or NAD(P)H oxidase as well as inhibition of oxygen-sensitive potassium channels have been proposed. The key downstream signalling pathways mediating pulmonary artery smooth muscle cell (PASMC) contraction comprise changes in intracellular Ca2+ concentration ([Ca2+]i)8–10 and activation of src family kinase (SFK)/Rho kinase pathways.11 In line with a central role for [Ca2+]i transients as second messenger triggering PASMC constriction,9,10,12 we and others have recently identified a critical contribution of transient receptor potential (TRP) family members TRPC613,14 and TRP vanilloid 4 (TRPV4)15 in the hypoxia-induced Ca2+ influx. However, the molecular mechanisms regulating TRP channel activity in response to hypoxia and their potential links to SFK/Rho kinase signalling have so far not been resolved. Here, we hypothesized that autocrine signalling via pannexin 1 (Panx1) may constitute a missing link between these signalling events.16–18
Panx1 is a widely expressed, large transmembrane channel permeable to ions and molecules up to 1 kDa in size19 that is best recognized for its emerging role as purine channel facilitating the release of ATP into the extracellular space.20 Extracellular ATP acts, in turn, as local autocrine (or paracrine) signal and stimulates cellular processes such as proliferation, inflammation, or cytokine production via its binding to purinergic receptors.21–23 Of relevance, Panx1-mediated ATP release has recently been identified as an important regulator of vascular tone in systemic arteries.24 We speculated that Panx1 may play a similar role in the pulmonary circulation and its response to hypoxia, as (i) Panx1 channels can be activated by hypoxia as recently demonstrated in neurons,25 (ii) Panx1 phosphorylation, which increases channel open probability, is commonly regulated by SFKs,26 and (iii) Panx1 has been closely linked to TRP channel signalling.18 Specifically, a hypothetical model has been proposed suggesting ATP release across Panx1 channels and subsequent Ca2+ influx through TRPC6 in wound closure.27 In line with this view, stimulation of purinergic P2X and P2Y receptors can activate protein kinases A and C,28,29 which in turn phosphorylate regulatory sites of TRP channels resulting in Ca2+ influx.30,31 Conversely, stimulation of TRPV4 has been shown to induce ATP release through Panx1 in airway epithelia and pulmonary fibroblasts, respectively.16,18 These findings raise the intriguing possibility of an oxygen-sensitive functional crosstalk between SFK, Panx1, and TRP channels in HPV that could link several key players of HPV into a single common pathway.
So far, a vasoregulatory role of Panx1 in the pulmonary circulation has not been tested for. Notably, such a role would implicate Panx1 as a potential therapeutic target for the treatment of diseases characterized by sustained elevations in lung vascular tone, such as PH or high altitude pulmonary oedema (HAPE). As Panx1 has recently been identified as a novel molecular target of the mineralocorticoid receptor (MR) antagonist spironolactone,32 pharmacological intervention with a well-established clinical drug could become rapidly implementable.
2. Methods
2.1 Animals
All animal procedures were approved by the local responsible animal care and use committee (St. Michael’s Toronto and LaGeSO Berlin, respectively), and all experiments were performed in accordance with the ‘Guide for the Care and Use of Laboratory Animals’ (Institute of Laboratory Animal Resources, 7th edition, 1996). Male C57BL/6J mice with a body weight (bw) of approximately 20 g were obtained from Charles River Laboratories (Wilmington, USA). Endothelial-specific (Cdh5-CreERT2/Panx1fl/fl) as well as smooth muscle specific (SMMHC-CreERT2/Panx1fl/fl) Panx1-deficient mice were provided by Brant Isakson (University of Virginia, Charlottesville, USA) and were bred on a C57BL/6J background. Cre-mediated Panx1 depletion was induced by feeding mice with tamoxifen (500 mg/kg bw; Harlan Laboratories, Indianapolis, USA) for 14 days prior to experiments.
2.2 Isolated perfused mouse lung
Mice were anaesthetized with a single intraperitoneal injection of ketamine (Ketamin 100 mg/kg bw) and xylazine (Xylavet 16 mg/kg bw; both cp-pharma, Burgdorf, Germany), and sacrificed via cervical dislocation. A tracheostomy was instantly performed and mouse lungs were ventilated in situ with normoxic gas (20% O2, 5% CO2, 75% N2). The pulmonary artery and the left atrium were cannulated and lungs were perfused continuously with HBSS (Biochrome; Berlin, Germany) containing 20% fetal bovine serum (FBS; Biochrom, Berlin, Germany) at 37°C and a flow rate of 50 mL min−1 kg−1. After stabilization of PAP, baseline PAP was recorded for 4–5 min under normoxic conditions via a P75 pressure sensor (Hugo Sachs Elektronik-Harvard Apparatus GmbH; Hugstetten, Germany). After switching to ventilation with hypoxic gas (1% O2, 5% CO2, 94% N2), the resulting increase in PAP (ΔPAP) was monitored for 5 min as a measure of the HPV response before ventilation was returned to normoxia.
2.3 Chronic hypoxic pulmonary hypertension
To investigate the role of Panx1 in the development of PH, tamoxifen-fed C57BL/6J, Cdh5-CreERT2/Panx1fl/fl as well as SMMHC-CreERT2/Panx1fl/fl mice were kept in animal chambers (BioSpherix, Parish, USA) under chronic hypoxic conditions (10% O2) for 5 weeks. C57BL/6J as well as Panx1fl/fl control mice were kept in normoxic conditions (21% O2) for the same time period. After 5 weeks of hypoxia or normoxia, respectively, mice were anaesthetized with a single intraperitoneal injection of ketamine (Ketamin 100 mg/kg bw) and xylazine (Xylavet 20 mg/kg bw; both cp-pharma, Burgdorf, Germany) and a 1F microtip Millar catheter (Millar, Houston, TX, USA) was induced via the right jugular vein into the right ventricle to monitor right ventricular systolic pressure (RVSP; DasyLab, Norton, USA). Next, mice were euthanized by exsanguinations and left lungs were excised and fixed in 10% paraformaldehyde solution for histological analysis. For assessment of right ventricular hypertrophy, the Fulton index was calculated as the ratio of the right ventricular weight to the weight of the left ventricle plus the septum (RV/[LV + S]). For analysis of vascular remodelling, dehydrated lungs were paraffin-embedded and cut into 5 µm slices. Lung sections were stained with haematoxylin–eosin, and medial wall thickness was determined in 15 pulmonary arterioles of 25–50 µm diameter at four different locations and mean wall thickness was normalized to the shortest cross-sectional diameter.
2.4 Cell culture
Primary human PASMCs (hPASMCs) and primary human pulmonary artery endothelial cells (hPAECs) from five different male caucasian donors were purchased from PromoCell (Heidelberg, Germany) and cultured at 95% relative humidity, 37°C and 5% CO2 using Smooth Muscle Cell Growth Medium (PromoCell; Heidelberg, Germany) and Endothelial Cell Growth Medium MV2 (PromoCell; Heidelberg, Germany), respectively, containing 10% FBS, 100 U/mL penicillin and 100 µg/mL streptomycin. Primary cells were used at passage 2-7. To facilitate multiple parallel measurements for Ca2+ imaging and ATP bioluminescence assay, immortalized SV40-hPASMC were utilized for their higher proliferation rate. SV40-hPASMC were obtained from abm® (Richmond, Canada) and cultured with DMEM (Gibco™ Thermo Scientific, Hamburg, Germany) under the same conditions as described above. HeLa cells (Sigma-Aldrich, Sreinheim, Germany) were used for overexpression experiments and cultured with DMEM as well. Prior to in vitro experiments, cells were serum starved over overnight.
2.5 Cell transfection
SV40-hPASMCs were seeded in eight well-chamber slides (ibidi; Gräfelfing, Germany) and cultured under normoxic conditions as per manufacturere’s instructions. For silencing of Panx1 and of the MR (NR3C2), SV40-hPASMCs were transfected with either siPanx1, siNR3C2 or scrambled control siRNA (25 nM: ON-TARGETplus; Dharmacon; Lafayette, USA). Panx1 and NR3C2 mRNA levels were assessed after 48 h by quantitative reverse transcriptase real-time polymerase chain reaction (qRT-PCR). Panx1 overexpression experiments were performed in HeLa cells to minimize confounding effects from basal Panx1 expression. To this end, HeLa cells were transfected with either a pcDNA3.1+/c-(K)DYK plasmid containing a Panx1 open reading frame (GenScript; Piscataway Township, USA) or a pcDNA3.1+/c-(K)DYK backbone control plasmid (GenScript; Piscataway Township, USA). Selection for plasmid carrying cells was made using G418. Panx1 expression was estimated by Western blotting and transfected cells were used for Ca2+ imaging.
2.6 Ca2+ imaging
For analysis of the intracellular Ca2+ concentration ([Ca2+]i) by real-time fluorescence imaging, SV40-hPASMC were seeded in perfusion slides (ibidi; Gräfelfing, Germany), loaded with Fura 2-AM (PromoKine; Heidelberg, Germany) as previously described,33 and constantly perfused with HBSS containing Ca2+ and Mg2+ (0.5 mL/min) at 37°C. After recording of baseline values for 3 min, the perfusion system was switched from a normoxic (pO2 ≈ 160 mmHg) to a hypoxic perfusate (pO2 < 35 mmHg). Fura 2 fluorescence was imaged on an inverse microscope (Axiovert 100; Zeiss; Jena, Germany) using TILLvisION software (TILLvisION 4.01; T.I.L.L. Photonics, Martinsried, Germany). Fluorescence was excited at 340, 360, and 380 nm via monochromatic illumination (Polychrome IV; T.I.L.L. Photonics; Martinsried, Germany) and detected via an appochromat objective (UAPO 40× W2/340; Olympus; Hamburg, Germany) and appropriate dichroic and emission filters (FT 425 and BP 505-530; Zeiss, Jena, Germany) by a SensiCam (T.I.L.L. Photonics; Gräfelfing, Germany). Changes in [Ca2+]i were assessed as fluorescence ratio at 340/380 nm and expressed relative to baseline.
2.7 ATP bioluminescence assay
SV40-hPASMCs were seeded in 12 well plates and cultured under normoxic conditions. On the day of the experiment, medium was removed and cells were carefully washed twice with warm serum-free DMEM medium supplemented with 1% BSA. Cells were then incubated for 30 min at 37°C to allow for degradation of extracellular ATP released in response to mechanical stimulation during washes. Endogenous ecto-nucleotidases were inhibited by addition of ARL 67156 (300 mMol/L; Tocris; Bristol, UK). Next, SV40-hPASMCs were exposed to normoxic (20% O2, 5% CO2, 75% N2) or hypoxic conditions (1% O2, 5% CO2, 94% N2) and the supernatant as well as cell lysate were collected after 5 and 15 min, respectively. ATP concentration was measured using an ATP bioluminescence assay HSII kit (Roche; Basel, Switzerland) according to manufacturer’s instructions.
2.8 Western blot analysis
Ten- to 20 µg protein of each sample were loaded on a 10% SDS-polyacrylamide gel. Protein lysates were separated for 90 min at 120 V using a Mini-PROTEAN electrophoresis system (Bio-Rad) followed by protein transfer to a nitrocellulose membrane for 90 min at 100 V using a wet blotting system. Next membranes were cut and blocked with tris buffered saline (TBS) containing 0.1% Tween (TBS-T), 3% BSA for 30 min followed by overnight incubation with specific primary antibodies dissolved in TBS-T containing 3% BSA at 4°C. Primary antibodies comprised rabbit anti-human Panx1 rAb, anti-human Panx1Tyr 198 rAb (Brant Isakson; University of Virginia; Charlottesville, USA) and anti-human NR3C2 rAb (Abcam; Cambridge, UK) as well as anti-human/mouse GAPDH rAb (Abcam; Cambridge, UK). The following day, membranes were washed and incubated with the appropriate horseradish peroxidase-conjugated secondary antibody at room temperature for 60 min. Proteins were visualized using a chemiluminescent substrate (ECL™ Prime western blotting detection reagent, Amersham, UK) and a Celvin® chemiluminescence imaging system.
2.9 Statistics
Results are shown as mean ± SEM (standard error of the mean) and statistical analyses were performed using GraphPad Prism 8.1 (GraphPad Software, USA). Mann–Whitney U-test was used to compare means of two groups. For more than two groups, Kruskal–Wallis test was performed followed by Dunn’s post hoc test. A P-value of <0.05 was considered statistically significant. The number of experiments (n) is indicated in each figure legend.
3. Results
3.1 Smooth muscle Panx1 is required for the intact HPV response
HPV has previously been shown to be mediated by signalling mechanisms in both pulmonary artery endothelial and smooth muscle cells.34 Western blot analysis revealed that both hPASMC and hPAEC express Panx1 at comparable levels (Figure 1A and B). To test for a potential role of Panx1 in HPV, the ΔPAP response to hypoxia was monitored in isolated perfused mouse lungs pretreated with the non-specific Panx1 antagonist probenecid (50 mg/kg bw; Sigma-Aldrich; Steinheim, Germany),35 the Panx1 specific inhibitory peptide 10Panx1 (800 µMol/L; Tocris; Bristol, UK),36 or respective vehicle controls. While untreated WT lungs showed a characteristic PAP increase in response to hypoxia, ΔPAP was markedly attenuated by both probenecid (Figure 1C and D) and 10Panx1 (Figure 1E). In line with Panx1 activation mediating pulmonary vasoconstriction, 10Panx1 also reduced the vasoconstrictive response to KCl (80 mMol/L), a known activator of Panx137 (Figure 1F). The HPV response was similarly reduced in lungs of mice with a smooth muscle cell (SMC)-specific Panx1 deficiency, yet not in EC-specific Panx1 deficient mice, suggesting a physiological role for SMC Panx1 in HPV (Figure 1G). Compared to the respective control mice, basal PAP resting values were neither lowered by Panx1 inhibition nor by Panx1 deficiency (data not shown). Hypoxia did, however, not regulate Panx1 expression in SMC at the mRNA or protein level, indicating a regulation by posttranslational modification (Figure 1H–J).
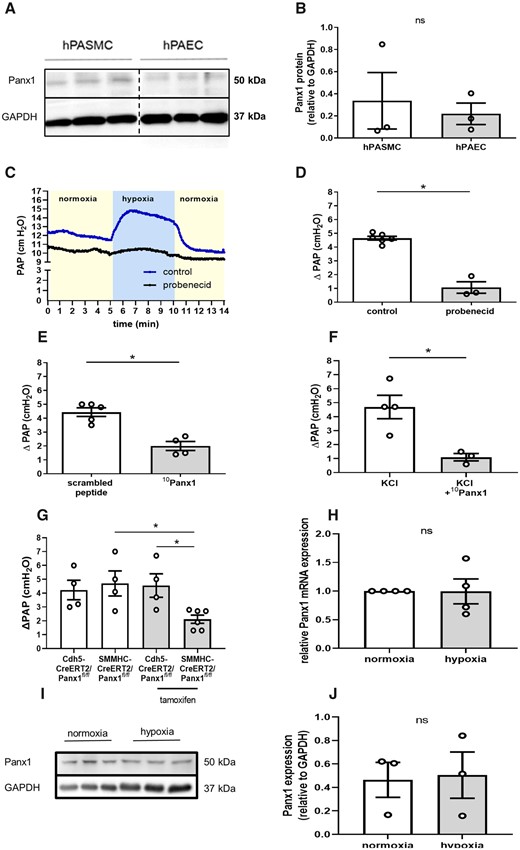
Smooth muscle Panx1 is required for the intact HPV response. Representative western blot (A) and quantitative densitometric analysis (B) show expression of Panx1 in human pulmonary artery smooth muscle cells (hPASMCs) and human pulmonary artery endothelial cells (hPAECs). GAPDH served as loading control (n = 3 per group). Panx1 expression on hPASMC and hPAEC were assessed on the same gel. Interspersed non-relevant lanes were removed and relevant sections were re-composed at the dotted line. Representative tracing (C) of pulmonary artery pressure (PAP) in isolated perfused mouse lungs and quantitative group data analysis (D) show attenuation of the vasoconstrictive response to hypoxia (1% O2)—measured as increase in PAP (ΔPAP)—by probenecid (50 mg/kg bw) (control n = 6, probenecid n = 3). (E) The pannexin 1 (Panx1) specific inhibitory peptide (10Panx1; 800 µMol/L) reduces the ΔPAP response to hypoxia by more than 50% as compared to scrambled peptide control (scrambled peptide n = 5, 10Panx1 n = 4). (F) Pulmonary vasoconstriction in response to KCl was attenuated by 10Panx1 (800 µMol/L) (KCl n = 4, KCl+10Panx1 n = 3). (G) Tamoxifen induced deletion of Panx1 in smooth muscle cells (SMMHC-CreERT2/Panx1fl/fl), yet not Panx1 deletion in endothelial cells (Cdh5-CreERT2/Panx1fl/fl) attenuates ΔPAP response to hypoxia in isolated perfused murine lungs (n = 4–5 per group). (I and J) Hypoxia did neither change Panx1 RNA levels (n = 4 per group) nor Panx1 protein expression (n = 3 per group). Data are mean ± SEM; Data were analysed using Mann–Whitney U-test (A–F, H–J) or Kruskal–Wallis test (Figure test); *P < 0.05; ns, not significant.
3.2 The Panx1 inhibitor spironolactone impairs HPV
As the MR antagonist spironolactone (Sigma-Aldrich, Steinheim, Germany) has recently been identified as a potent inhibitor of Panx1,32 we next tested the effect of spironolactone on HPV. In murine isolated perfused lung (IPL), quantification of ΔPAP in response to hypoxia revealed a progressive attenuation of HPV with increasing concentrations of spironolactone and an IC50 of 28 µMol/L (Figure 2A and B). To obtain insights into the mechanistic role of Panx1, we next monitored the PASMC [Ca2+]i response as an essential requirement for an intact HPV response. In hPASMC, hypoxia induced a characteristic increase in [Ca2+]i that was, however, significantly attenuated by spironolactone at 20 µMol/L, i.e. a dose in the range of the IC50 for HPV inhibition. A similar inhibitory effect of approximately 50% was achieved by Panx1 inhibitory peptide 10Panx1 (Figure 2C–F) as well as by Panx1 knockdown (Figure 2G–J). Notably, neither 10Panx1 nor spironolactone altered baseline [Ca2+]i. To test for a potential contribution of the MR (NR3C2) as alternative target of spironolactone, NR3C2 was silenced by siRNA knockdown in hPASMC, which was confirmed by RT-qPCR and western blot. However, knockdown of NR3C2 did not affect the [Ca2+]i response to hypoxia as compared to cells transfected with scrambled siRNA (Figure 2K–N).
![The Panx1 inhibitor spironolactone attenuates HPV. Representative tracing (A) of pulmonary artery pressure (PAP) in isolated perfused mouse lungs and quantitative group data analysis (B) show attenuation of the vasoconstrictive response to hypoxia (1% O2)—measured as increase in PAP (ΔPAP)—by spironolactone (20 µMol/L in (A); 0–40 µMol/L in (B)) in a dose-dependent manner (n = 5 per group). Time-resolved group data (C) and quantification of area-under-the-curve (AUC) (D) show attenuated increase in intracellular Ca2+ concentration in response to hypoxia—as measured by ratiometric imaging of Fura 2 at 340 and 380 nm (F340/F380) in hPASMC—by spironolactone as compared to vehicle (n = 4 each). Time-resolved group data (E) and quantification of area under the curve (AUC) (F) show attenuated increase in intracellular Ca2+ concentration in response to hypoxia in hPASMC by the Panx1 specific inhibitory peptide 10Panx1 (200 µMol/L, n = 9) as compared to scrambled peptide (n = 7). (G–J) Knockdown of Panx1 decreased both RNA as well as protein levels and led to a marked attenuation of the [Ca2+]i response to hypoxia. (K–M) In hPASMC, knockdown of NR3C2 by siRNA (siNR3C2, n = 7) reduced NR3C2 mRNA expression as well as NR3C2 protein levels compared to control cells treated with non-targeting scrambled siRNA (siScr, n = 4–6) but (N) did not affect the [Ca2+]i increase in response to hypoxia. Data are mean ± SEM; Data were analysed using Kruskal–Wallis test (B) or Mann–Whitney U-test (D, F, G, I, J, K, M, N); *P < 0.05; ns, not significant.](https://oup.silverchair-cdn.com/oup/backfile/Content_public/Journal/cardiovascres/118/11/10.1093_cvr_cvab326/2/m_cvab326f2.jpeg?Expires=1749832000&Signature=0HK-cZLfbgHIa4vm~MSCeSx5mcMCZDMbm3P7JK8S94hHA~2nZSXFCfSg7~7b1xUKcEQAO9rMkwSQjf~4tM9gmmShxim9jQjEkUAQWE~I-iSwUkVl21NCTFuqVcOJnwwo5j4G1v3YxYpFHzvjBUR4RJiL6WBdNIDs7OemNnO7IQkLIUYmad8C6uZQknDP0jM~SFsyOAchfikkLHDenMQNA~6x0DgVXcUKAaJStogBipwfcsYuocZvTprEPEi2ZXG2IwCf7xZ3trcYoL~PFq8u6TdKQe4GPQUYEhvy79Yf7a4lhKZqgNPTeqbocikkBFUnqPJCiOHcWleuOdx6mXnc4w__&Key-Pair-Id=APKAIE5G5CRDK6RD3PGA)
The Panx1 inhibitor spironolactone attenuates HPV. Representative tracing (A) of pulmonary artery pressure (PAP) in isolated perfused mouse lungs and quantitative group data analysis (B) show attenuation of the vasoconstrictive response to hypoxia (1% O2)—measured as increase in PAP (ΔPAP)—by spironolactone (20 µMol/L in (A); 0–40 µMol/L in (B)) in a dose-dependent manner (n = 5 per group). Time-resolved group data (C) and quantification of area-under-the-curve (AUC) (D) show attenuated increase in intracellular Ca2+ concentration in response to hypoxia—as measured by ratiometric imaging of Fura 2 at 340 and 380 nm (F340/F380) in hPASMC—by spironolactone as compared to vehicle (n = 4 each). Time-resolved group data (E) and quantification of area under the curve (AUC) (F) show attenuated increase in intracellular Ca2+ concentration in response to hypoxia in hPASMC by the Panx1 specific inhibitory peptide 10Panx1 (200 µMol/L, n = 9) as compared to scrambled peptide (n = 7). (G–J) Knockdown of Panx1 decreased both RNA as well as protein levels and led to a marked attenuation of the [Ca2+]i response to hypoxia. (K–M) In hPASMC, knockdown of NR3C2 by siRNA (siNR3C2, n = 7) reduced NR3C2 mRNA expression as well as NR3C2 protein levels compared to control cells treated with non-targeting scrambled siRNA (siScr, n = 4–6) but (N) did not affect the [Ca2+]i increase in response to hypoxia. Data are mean ± SEM; Data were analysed using Kruskal–Wallis test (B) or Mann–Whitney U-test (D, F, G, I, J, K, M, N); *P < 0.05; ns, not significant.
3.3 Panx1 deficiency does not protect from chronic hypoxic pulmonary hypertension
Since mechanisms of HPV commonly also play critical roles in the development of chronic hypoxic PH, we next tested for the role of Panx1 in chronic hypoxic mice. As compared to normoxic mice, chronic hypoxic C57BL/6J developed characteristic signs of PH and lung vascular remodelling. Specifically, RVSP was found to be elevated in haemodynamic monitoring (Figure 3A), right ventricular hypertrophy was evident as increased Fulton index, and lung vascular remodelling as medial thickening of small (25–50 µm diameter) pulmonary arterioles (Figure 3B–D). Analogous changes in pulmonary haemodynamics and vascular structure were equally evident in mice with either an endothelial- or a smooth muscle cell-specific Panx1 deficiency with no significant differences between genotypes (Figure 3), indicating that despite its role in HPV, Panx1 does not play a major role in lung vascular remodelling in response to chronic hypoxia. Notably, right ventricular hypertrophy was less pronounced in chronic hypoxic mice with an endothelial-specific Panx1 deficiency as compared to C57BL/6J (Figure 3B), yet whether this finding points to specific role of endothelial Panx1 in RV remodelling remains to be determined.
![Panx1 deficiency does not protect from chronic hypoxic pulmonary hypertension. Mice with a tamoxifen-induced deletion of Panx1 in smooth muscle cells (SMMHC-CreERT2/Panx1fl/fl) or endothelial cells (Cdh5-CreERT2/Panx1fl/fl) or corresponding wild-type controls (C57BL/6J) were kept under normoxic (21% O2) or hypoxic (10% O2) conditions for weeks for induction of chronic hypoxic pulmonary hypertension. As compared to normoxia (white bars), hypoxia (grey bars) caused (A) an increase in right ventricular systolic pressure (RVSP) as well as (B) right ventricular hypertrophy [right ventricular weight/(left ventricular + septal weight); Fulton index] in C57BL/6J mice. Similar effects were observed in Cdh5-CreERT2/Panx1fl/fl and SMMHC-CreERT2/Panx1fl/fl mice with no significant differences between genotypes except for Fulton index which was lower in Cdh5-CreERT2/Panx1fl/fl as compared to C57BL/6J mice. (C) Representative haematoxylin and eosin stainings show lung vascular remodelling in chronic hypoxic mice. Black arrows highlight pulmonary arterioles of 25–50 µm diameter. Wall thickness was determined at four different locations and mean wall thickness was normalized to the shortest cross-sectional diameter. (D) Medial thickening in pulmonary arterioles, was observed in C57BL/6J, Cdh5-CreERT2/Panx1fl/fl, and SMMHC-CreERT2/Panx1fl/fl mice as characteristics of pulmonary hypertension (n = 8–10 per group). Data are mean ± SEM; data were analysed using one-way ANOVA; *P < 0.05.](https://oup.silverchair-cdn.com/oup/backfile/Content_public/Journal/cardiovascres/118/11/10.1093_cvr_cvab326/2/m_cvab326f3.jpeg?Expires=1749832000&Signature=gktdSunbqxOijn3FTGSakRas7mCV4iY60e-8atT3yKin-BIi9htBl9XyVirxaH7qYL1v6BnAuOaQTiLZETRYbBUjFtDv-EF-UJBWMTPpQzlvf5gpFehyoPovgIoIXO6vo3651OwGaxxqTMQ-2oSR1P-hYJ0ht6ZnrBvkKoBvQ8r56b4clnSR2zw8-GDaD-rUjOqZeKb6zTRVD~5SK5bBXlEleCVxDUiUt74~ap6yga~w9~nK5MbPF859JUXttjvSOWH8Oz9i7Gxlpih1zj8WKNuuBRRHdxOXDv-f1mNrMfQ10Ctm6RLnSxOeMQ~jXG~tezLr71ajCBdzRIGPudbVuw__&Key-Pair-Id=APKAIE5G5CRDK6RD3PGA)
Panx1 deficiency does not protect from chronic hypoxic pulmonary hypertension. Mice with a tamoxifen-induced deletion of Panx1 in smooth muscle cells (SMMHC-CreERT2/Panx1fl/fl) or endothelial cells (Cdh5-CreERT2/Panx1fl/fl) or corresponding wild-type controls (C57BL/6J) were kept under normoxic (21% O2) or hypoxic (10% O2) conditions for weeks for induction of chronic hypoxic pulmonary hypertension. As compared to normoxia (white bars), hypoxia (grey bars) caused (A) an increase in right ventricular systolic pressure (RVSP) as well as (B) right ventricular hypertrophy [right ventricular weight/(left ventricular + septal weight); Fulton index] in C57BL/6J mice. Similar effects were observed in Cdh5-CreERT2/Panx1fl/fl and SMMHC-CreERT2/Panx1fl/fl mice with no significant differences between genotypes except for Fulton index which was lower in Cdh5-CreERT2/Panx1fl/fl as compared to C57BL/6J mice. (C) Representative haematoxylin and eosin stainings show lung vascular remodelling in chronic hypoxic mice. Black arrows highlight pulmonary arterioles of 25–50 µm diameter. Wall thickness was determined at four different locations and mean wall thickness was normalized to the shortest cross-sectional diameter. (D) Medial thickening in pulmonary arterioles, was observed in C57BL/6J, Cdh5-CreERT2/Panx1fl/fl, and SMMHC-CreERT2/Panx1fl/fl mice as characteristics of pulmonary hypertension (n = 8–10 per group). Data are mean ± SEM; data were analysed using one-way ANOVA; *P < 0.05.
3.4 Hypoxia induces Panx1 phosphorylation at Tyr198
SFK has been reported to play an important role in the activation of Panx1 in response to α1-adrenergic stimulation and, consequently, in vasoconstrictive responses in the systemic circulation.38,39 To test whether SFK may also be involved in the activation of Panx1 and the subsequent vasoconstrictive response to hypoxia in the pulmonary circulation, we probed for Panx1 phosphorylation at the SFK-sensitive phosphorylation site Tyr198 that is localized within the intracellular loop of Panx1.40 As compared to normoxic controls, hypoxia (1% O2 for 5 min) increased Panx1 phosphorylation at Tyr198 which could be blocked by the SFK inhibitor PP2 (10 mMol/L) (Figure 4A and B), while neither hypoxia nor PP2 affected total Panx1 expression (Figure 4C). In line with this finding, PP2 (20 mMol/L) largely blocked the HPV response in isolated perfused mouse lungs (Figure 4D).
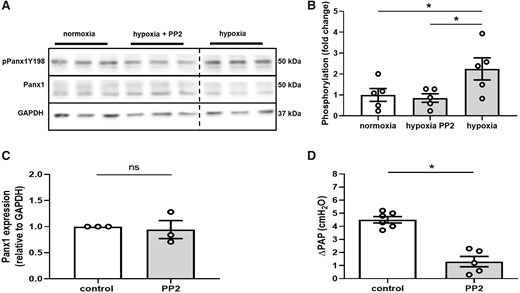
Hypoxia induces Panx1 phosphorylation at Tyr198. Representative western blot (A) and quantitative densitometric analysis (B) show increased phosphorylation of Panx1 at residue Tyr198 (pPanx1Y198) in hypoxic as compared to normoxic hPASMC that is blocked by PP2 (10 µMol/L) (n = 5 per group). Panx1 phosphorylation on hPASMC and hPAEC were assessed on the same gel. Interspersed non-relevant lanes were removed and relevant sections were re-composed at the dotted line. (C) PP2 did not alter Panx1 expression. (D) In isolated perfused mouse lungs, PP2 (20 µMol/L) attenuated the vasoconstrictive response to hypoxia (1% O2), measured as increase in pulmonary artery pressure (ΔPAP) (control n = 6, PP2 n = 5). Data are mean ± SEM; data were analysed using Kruskal–Wallis test (B) or Mann–Whitney U-test (C and D); *P < 0.05.
3.5 Panx1 does not regulate HPV via ATP release and purinergic signalling
In the past, the functional effects of Panx1 in vascular or parenchymatous cells have been largely attributed to its role as an ATP channel with Panx1-mediated ATP release triggering autocrine or paracrine responses via purinergic receptor signalling. To probe for a similar role of Panx1 in the regulation of HPV, we next measured ATP release from hPASMC in response to hypoxia. Surprisingly, hypoxia failed to increase extracellular ATP levels, but instead resulted in a significant drop in extracellular ATP after 5 min of hypoxia, i.e. within the time frame of the PASMC Ca2+ response (Figure 5A). A similar loss of extracellular ATP was detected in longitudinal measurements of extracellular ATP over 15 min of hypoxia (Figure 5B). Rapid degradation of extracellular ATP in these assays was excluded by addition of the ecto-nucleotidase inhibitor ARL 67156 (300 µMol/L), which effectively blocked the degradation of exogenous ATP in this setting (data not shown). Notably, hypoxia also reduced intracellular ATP levels in hPASMC (Figure 5A), a finding that likely accounts for the parallel decrease in extracellular ATP.
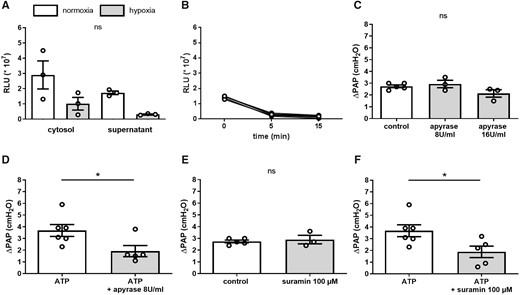
Panx1 does not regulate HPV via ATP release and purinergic signalling. (A) Group data show ATP concentration in supernatant and cytosolic fraction of hPASMCs under normoxic (21% O2; white bars) and hypoxic (1% O2; grey bars) conditions after 5 min. Hypoxia decreased both extracellular and intracellular ATP levels (n = 3 per group). (B) Longitudinal measurements over 15 min of hypoxia show a corresponding decrease in extracellular ATP concentration. (C) In isolated perfused mouse lungs, pretreatment with the ATP degrading enzyme apyrase (8 U/mL and 16 U/mL) did not alter the vasoconstrictive response to hypoxia (1% O2), measured as increase in pulmonary artery pressure (ΔPAP) (control n = 5, apyrase 8 U/mL n = 3, apyrase 16 U/mL n = 3). (I) In isolated perfused mouse lungs, administration of an ATP bolus (0.9 µmol) induced pulmonary vasoconstriction (n = 6), measured as increase in pulmonary artery pressure (ΔPAP), which was reduced by apyrase (8 U/mL; n = 5). (J) In isolated perfused mouse lungs, pretreatment with the purinergic receptor blocker suramin (100 µMol/L) did not alter the vasoconstrictive response to hypoxia (control n = 5, suramin n = 3). (K) Suramin (100 µMol/L) attenuated the vasoconstrictive response to an ATP bolus (0.9 µmol) (ATP = 6, ATP + suramin n = 5). Data are mean ± SEM; data were analysed using Mann–Whitney U-test (A, D–F) or Kruskal–Wallis test (C); *P < 0.05; ns, not significant.
To verify the unexpected finding that the role of Panx1 in HPV may be independent of ATP release, we perfused isolated mouse lungs with either the ATP-degrading enzyme apyrase or the purinergic receptor blocker suramin. Neither of the two interventions, however, altered the HPV response in isolated mouse lungs, although both proved effective to attenuate the pulmonary vasoconstrictive response to exogenous ATP (Figure 5E–H).
3.6 Panx1 modulates the Ca2+ response to hypoxia but acts independently of TRPV4
These data indicate that the role of Panx1 in HPV is independent of its classic function as ATP channel, yet relates to Panx1 modulating the PASMC Ca2+ response to hypoxia. In line with this view, re-supplementation of extracellular Ca2+ to HeLa cells kept under hypoxic conditions in Ca2+ free medium induced a more pronounced Ca2+ influx in HeLa cells overexpressing Panx1 as compared to cells treated with empty control vector (Figure 6A–D). Regulation of vasomotor tone by Panx1 has previously been closely linked to activation of the polymodal cation channel TRPV4, with either TRPV4-mediated Ca2+ influx activating Panx1, or Panx1-mediated ATP release activating TRP channels. The concept of a signalling interaction between Panx1 and TRPV4 in HPV is particularly intriguing in view of our previous identification of a critical role for TRPV4 in the PASMC Ca2+ response to hypoxia and the subsequent HPV response.15 However, inhibition of Panx1 by 10Panx1 (800 µMol/L) failed to attenuate the pulmonary vasoconstrictive response to the TRPV4 activator GSK1016790A (100 nMol/L) (Figure 6E). Rather, parallel administration of the TRPV4 antagonist HC-067047 (20 µMol/L) and 10Panx1 (200 µMol/L) resulted in an additive inhibition of the hypoxia-induced [Ca2+]i increase in hPASMC as compared to treatment with either HC-067047 or 10Panx1 (Figure 6F). These findings suggest a role of Panx1 in HPV that is largely independent of upstream or downstream TRPV4.
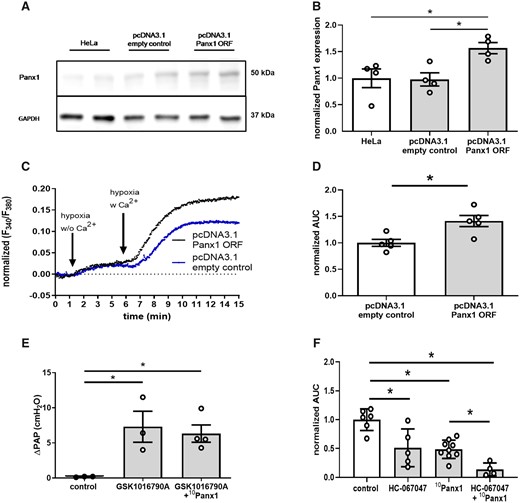
Panx1 acts independent of TRPV4 and may constitute a novel Ca2+ entry channel in HPV. (A) Representative western blot and (B) quantitative densitometric analysis show increased expression of Panx1 in HeLa cells transfected with pcDNA3.1+/c-(K)DYK vector containing Panx1 open reading frame (pcDNA3.1 Panx1 ORF) as compared to pcDNA3.1+/c-(K)DYK backbone control vector (pcDNA3.1 empty control) or non-transfected HeLa cells (HeLa). GAPDH served as loading control (n = 4 per group). Representative tracing (C) of intracellular Ca2+ concentration as measured by ratiometric imaging of fura-2 at 340 and 380 nm (F340/F380) and quantitative analysis of area under the curve (AUC) in HeLa cells (D). In the absence of extracellular Ca2+ (w/o Ca2+), hypoxia (1% O2) causes a slight increase in intracellular Ca2+ concentration in both Panx1 overexpressing (pcDNA3.1 Panx1 ORF) and control (pcDNA3.1 empty control) cells. Following re-supplementation of extracellular Ca2+ (w Ca2+), the increase in intracellular Ca2+ concentration is more pronounced in pcDNA3.1 Panx1 ORF as compared to pcDNA3.1 empty control HeLa cells (n = 5 per group). (E) Group data show vasoconstrictive response to the TRPV4 agonist GSK1016790A (100 nMol/L; n = 3) in isolated perfused mouse lungs, measured as increase in pulmonary artery pressure (ΔPAP), which was unabated by the Panx1 specific inhibitory peptide 10Panx1 (800 µMol/L; n = 4). (F) Simultaneous treatment of PASMC by the TRRPV4 antagonist HC-067047 (20 µMol/L) and 10Panx1 (200 µMol/L) additively attenuated the increase in intracellular Ca2+ concentration in response to hypoxia as measured by ratiometric imaging of Fura-2. Data are mean ± SEM; data were analysed using Kruskal–Wallis test (B, E, F) or Mann–Whitney U-test (D); *P < 0.05; ns, not significant.
4. Discussion
The present findings establish a critical role for Panx1 in the acute pulmonary vascular response to hypoxia. Specifically, hypoxia-induced PP2-sensitive Tyr198-phosphorylation of Panx1, and pharmacological inhibition or genetic deficiency of Panx1 as well as PP2 reduced HPV by >50%. In line with its functional role in HPV, Panx1 was required for an unabated Ca2+ response to hypoxia in PASMC. Surprisingly, this effect was not attributable to Panx1-mediated ATP release and subsequent auto- or paracrine purinergic signalling, but rather relates to Panx1 either directly or indirectly modulating the PASMC Ca2+ response to hypoxia.
First identified in 2000,41 pannexins are now recognized as hexameric42 or—as recently proposed—heptameric43 transmembrane channels that share structural similarities with connexin hemichannels.44 In contrast to the latter, however, pannexins do not form intercellular gap junctions, but constitute large, regulated channels that are best known for their ability to transport ATP, ions and other molecules up to 1 kDa in size.45 Of the three known pannexin family members, pannexin 2 and 3 are largely restricted to the central nervous system and bony structure, respectively, whereas Panx1 is ubiquitously expressed.46,47 Of late, Panx1 expression in vascular smooth muscle cells has become implicated in sympathetic vasoconstriction, in that α1 adrenoreceptor activation triggers ATP release via Panx1 which in turn increases systemic vascular resistance thus contributing to blood pressure regulation.40 While originally described in murine models, similar results could recently be replicated in the human leg vasculature by use of the non-specific Panx1 inhibitor probenecid.48 In contrast, vasoconstrictive responses to other stimuli such as serotonin or endothelin-1 seem to be independent of Panx1,40 suggesting that the role of Panx1 in vasoregulation is stimulus-specific. Albeit previous reports by us and others had implicated both connexins49 as well as purinergic signalling50 in the vasoconstrictive response of the pulmonary circulation to hypoxia, the role of Panx1 in HPV had so far not been addressed.
In the present study, we identify Panx1 to be expressed in hPASMC as well as in hPAEC, both of which have been previously implicated in HPV.49 Quantitative measurement of HPV in isolated perfused mouse lungs identified a critical requirement for Panx1, as not only the non-specific Panx1 inhibitor probenecid but also the highly specific mimetic peptide 10Panx1 attenuated the acute ΔPAP in response to hypoxia by 80% and 50%, respectively. Subsequent analyses in lungs of conditional SMC- and EC-specific Panx1 deficient mice revealed that the role of Panx1 in HPV was attributable to its expression in PASMC rather than PAEC. As such, these findings mirror the previously reported role of SMC Panx1 in sympathetic vasoconstriction of systemic resistance vessels, and identify hypoxia as a novel activator of Panx1 in the pulmonary circulation.
Recently, spironolactone has been identified as potent inhibitor of Panx1.32 This effect is seemingly independent of spironolactone’s role as MR antagonist, as non-steroidal MR antagonists such as finerenone do not interfere with Panx1 function.32 In line with a role for Panx1 in HPV, spironolactone effectively inhibited HPV in a dose-dependent manner. It should be noted, however, that the spironolactone concentrations for Panx1 inhibition in our present as well as in previous studies markedly exceed the maximum blood concentration in humans after taking 100 mg dose by a factor of 12.51 It is hence not to be expected that spironolactone therapy in routine clinical practice will necessarily result in an impaired HPV response. Yet, it should be noted that several studies from the 1970’s and 1980’s reported that two to three times of 25 mg/day spironolactone could prevent the formation of acute pulmonary oedema and dyspnoea during mountain trekking, i.e. of symptoms commonly attributed to an excessive HPV response at high altitude.52–54 As such, it remains to be determined whether low-dose spironolactone may have direct effects on HPV in such a scenario.
Spironolactone has recently also been proposed as a novel strategy for the treatment of pulmonary arterial hypertension (PAH) based on studies in chronic hypoxic mice and monocrotaline-treated rats in which spironolactone (15 mg·kg−1·day−1) attenuated the respective increase in RVSP, pulmonary arterial muscularization, and right ventricular fibrosis.55 These promising results have fuelled several clinical trials to test the therapeutic potential of spironolactone for the treatment of PAH which showed that spironolactone is safe, well-tolerated, and potentially clinically beneficial in PAH patients.56,57 While the underlying rationale for these studies is primarily based on a proposed role of MR signalling in PASMC proliferation, it is worthwhile to consider to which extent these findings may be alternatively attributable to the inhibitory effect of spironolactone on Panx1. Our present findings, however, do not support the latter hypothesis, as neither the endothelial nor the smooth muscle cell-specific deletion of Panx1 in mice prevented or attenuated the development of chronic hypoxic PH, or the associated lung vascular remodelling. Although we cannot exclude that previously reported systemic haemodynamic effects of vascular cell-specific deletion of Panx1 32 may have influenced the PH phenotype in chronic hypoxic mice, the present findings suggest that pharmacological effects of spironolactone in PH are likely unrelated to Panx1. As such, while many of the key signalling molecules involved in HPV including TRPC6, connexin 40, or TRPV4 also play important roles in lung vascular remodelling in PAH, this does not seem to be the case for Panx1.
Previous work by DeLalio et al.38 suggested that src kinase mediated phosphorylation of Panx1-Tyr198 is essential for the activation of Panx1 in the vascular system. In line with this concept and an active role of Panx1 in HPV, we found Panx1-Tyr198 in PASMC to become phosphorylated under hypoxic conditions. This phosphorylation was again blunted by the SFK antagonist PP2. As such, our present findings demonstrate that hypoxia induces Tyr198 phosphorylation of Panx1 in a PP2-sensitive manner, which aligns with the inhibition of HPV by PP2 in isolated lungs. The latter finding is in agreement with previous data demonstrating hypoxia-induced phosphorylation of several SFKs including src, fyn, and yes in rat pulmonary arteries, and prevention of hypoxia-induced Rho-associated protein kinase (ROCK) activation by siRNA-mediated silencing of src and—in part—also fyn in PASMC.11 Yet, albeit PP2 has been used extensively in the past as an SFK-selective inhibitor, recent kinome profiling has identified that PP2 may also regulate several other kinases with similar affinities.58 Moreover, PP2-sensitive kinases may phosphorylate other kinases such as ROCK which may be involved in the regulation of the contractile machinery as well.59 As such, it remains to be shown whether the observed Tyr198 phosphorylation of Panx1 in hypoxia is indeed specific for SFKs, and if so, for which family members, and whether it is required for an intact HPV response.
Notably, hypoxia-dependent phosphorylation of Panx1-Tyr198 appears to be specific for PASMC, as Panx1-Tyr198 was found to be constitutively phosphorylated in human coronary smooth muscle cells.38 As constitutive activation of Panx1 in vascular smooth muscle cells has previously been implicated in the generation of basal vascular tone in the systemic circulation,40 this specificity may—at least in part—explain the low resting tone of the pulmonary vasculature. In line with the unique role of HPV in the lung, Panx1-Tyr198 phosphorylation in response to hypoxia may then trigger vasoconstriction selectively in pulmonary, yet not in systemic blood vessels where Panx1-Tyr198 is already constitutively phosphorylated.
In contrast to its well-established role as ATP channel, our findings both in isolated lungs as well as in cultured PASMCs suggest that Panx1 acts via mechanisms other than ATP release in HPV. This notion is based on the findings (i) that hypoxia did not increase but rather decreased extracellular ATP in PASMC, (ii) that degradation of extracellular ATP by apyrase did not attenuate HPV, and (iii) that inhibition of purinergic receptor signalling by suramin likewise did not alter the haemodynamic response to hypoxia in the murine IPL. Hence, we considered alternative mechanisms by which activation of Panx1 may trigger pulmonary vasoconstriction.
Our findings that Panx1 antagonists, such as spironolactone or 10Panx1, as well as siRNA-mediated knockdown of Panx1 markedly attenuated the [Ca2+]i response to hypoxia point to an important role for Panx1 in the regulation of Ca2+ influx as the final trigger of HPV. In line with this notion, overexpression of Panx1 increased the influx of extracellular Ca2+ into HeLa cells in response to hypoxia. Simultaneous inhibition of TRPV4 by HC-067047 and Panx1 by 10Panx1 resulted in an additive inhibitory effect on the PASMC [Ca2+]i response to hypoxia suggesting that Panx1 may regulate [Ca2+]i influx independent of classic HPV-related Ca2+ influx channels such as TRPV4. The exact molecular mechanisms by which Panx1 modulates the PASMC [Ca2+]i response remain to be elucidated. Potentially, these may involve a direct role of Panx1 as Ca2+ channel per se, as suggested by several recent studies.60,61 Specifically, Panx1 shows structural and functional similarities to the Ca2+-permeable cation channel Calcium Homeostasis Modulator 1 (CALHM1)62 and Panx1 overexpression or silencing has been associated with corresponding changes in transmembrane Ca2+ leak rate.63 However, and in contrast to this hypothesis, divalent cations such as Ca2+ have also been reported to suppress Panx1 activation to its large conductance state64 which permits permeation of molecules up to 1 kDa. Conversely, Panx1’s low conductance state is highly selective permeable to Cl− ions.64 This raises the alternative hypothesis that Panx1 might regulate hypoxia-induced [Ca2+]i responses indirectly via Cl− efflux and subsequent Ca2+ influx through voltage-operated Ca2+ channels, which play a well-established role in HPV.65 Other mechanisms and pathways may exist by which Panx1 may indirectly modulate PASMC Ca2+ homeostasis and signalling, such as Panx1-P2X7 coupling via ATP-independent mechanisms.37 This area of research clearly deserves further in-depth exploration.
Supplementary material
Supplementary material is available at Cardiovascular Research online.
Authors’ contributions
B.G., A.K., J.G., B.E.I., and W.M.K. conceived the study; B.G., A.K., X.H., and M.L. performed experiments and data analysis; C.M. provided expert advice on the electrophysiology of Panx1 channels; B.G. and W.M.K. drafted; and all authors edited and approved the original manuscript.
Acknowledgements
We thank the Advanced Medical Bioimaging Core Facility of the Charité-Universitätsmedizin (AMBIO) for support in acquisition (and/or analysis) of the imaging data. Moreover, we thank Jörg Geiger for expert advice on electrophysiological assessment of Ca2+ channels.
Funding
This work was supported by the German Society of Cardiology (DGK) and by the Sonnenfeld-Foundation.
Data Availability
The data underlying this article will be shared on reasonable request to the corresponding author.
Translational perspective
Hypoxic pulmonary vasoconstriction (HPV) optimizes lung ventilation-perfusion matching, but also contributes to pulmonary pathologies including high altitude pulmonary edema (HAPE) or chronic hypoxic pulmonary hypertension. Here, we demonstrate that pharmaceutical inhibition as well as genetic deletion of the hemichannel pannexin 1 (Panx1) in pulmonary artery smooth muscle cells attenuates the physiological HPV response. Panx1 deficiency did, however, not prevent the development of chronic hypoxic pulmonary hypertension in mice. Panx1 inhibitors such as the mineralocorticoid receptor antagonist spironolactone may thus present a putative strategy for the prevention or treatment of HAPE, yet not for chronic hypoxic lung disease.
References
Author notes
Conflict of interest: none declared.