-
PDF
- Split View
-
Views
-
Cite
Cite
Monika Gawałko, Thomas A Agbaedeng, Arnela Saljic, Dominik N Müller, Nicola Wilck, Renate Schnabel, John Penders, Michiel Rienstra, Isabelle van Gelder, Thomas Jespersen, Ulrich Schotten, Harry J G M Crijns, Jonathan M Kalman, Prashanthan Sanders, Stanley Nattel, Dobromir Dobrev, Dominik Linz, Gut microbiota, dysbiosis and atrial fibrillation. Arrhythmogenic mechanisms and potential clinical implications, Cardiovascular Research, Volume 118, Issue 11, July 2022, Pages 2415–2427, https://doi.org/10.1093/cvr/cvab292
- Share Icon Share
Abstract
Recent preclinical and observational cohort studies have implicated imbalances in gut microbiota composition as a contributor to atrial fibrillation (AF). The gut microbiota is a complex and dynamic ecosystem containing trillions of microorganisms, which produces bioactive metabolites influencing host health and disease development. In addition to host-specific determinants, lifestyle-related factors such as diet and drugs are important determinants of the gut microbiota composition. In this review, we discuss the evidence suggesting a potential bidirectional association between AF and gut microbiota, identifying gut microbiota-derived metabolites as possible regulators of the AF substrate. We summarize the effect of gut microbiota on the development and progression of AF risk factors, including heart failure, hypertension, obesity, and coronary artery disease. We also discuss the potential anti-arrhythmic effects of pharmacological and diet-induced modifications of gut microbiota composition, which may modulate and prevent the progression to AF. Finally, we highlight important gaps in knowledge and areas requiring future investigation. Although data supporting a direct relationship between gut microbiota and AF are very limited at the present time, emerging preclinical and clinical research dealing with mechanistic interactions between gut microbiota and AF is important as it may lead to new insights into AF pathophysiology and the discovery of novel therapeutic targets for AF.

Gut microbiota and possible molecular pathways linked to AF. Dashed lines indicate potential mechanisms in AF pathogenesis. Solid lines indicate evidence-based mechanisms in AF pathogenesis. The BAs part deals with the primary/ secondary BAs ratio. AF, atrial fibrillation; BAs, bile acids; DAD, delayed afterdepolarizations; EAD, early after depolarizations; ERP, effective refractory period; LPS, lipopolysaccharide; SCFA, short-chain fatty acids; TMA, trimethylamine; TMAO, trimethylamine N-oxide.
1. Introduction
Atrial fibrillation (AF) is the most prevalent sustained cardiac arrhythmia, affecting more than 37 million people worldwide.1 Clinical and preclinical observations suggest that a large number of concomitant modifiable cardiovascular risk factors determine the manifestation and progression of AF.2 Interestingly, most AF risk factors such as coronary artery disease,3 heart failure,4 hypertension,5 diabetes, and obesity6 are linked to dietary intake and lifestyle components.
Following transition through the stomach, food reaches the small intestine and interacts with commensal bacteria, termed gut microbiota, within the gut lumen. Disruption of the gut microbiota composition in comparison with healthy individuals, termed ‘gut dysbiosis’, may affect the integrity of the intestinal barrier and the release of neurotransmitters and gastrointestinal hormones. In turn, gut dysbiosis influences the progression of cardiovascular risk factors.7 The effects of changes in the gut microbiota on AF are poorly understood.
In this review, we report the evidence linking gut microbiota profile to AF. We consider newly discovered metabolic pathways identified on the basis of gut microbiota-derived metabolites and their potential links to AF. We also summarize effects of AF on the gut microbiota, along with the impact of alterations in gut microbiota on AF risk factors and determinants. The potential value of pharmacological and diet-induced modification of the gut microbiota composition in AF management is discussed. We end by highlighting important knowledge gaps in this evolving field and areas requiring future investigation.
2. Brief overview of gut microbiota
The term ‘microbiota’ describes a collection of microorganisms defined as the ecological community of commensal, symbiotic and pathogenic microorganisms that literally share our body space.8 The human body contains nearly the same number of microbial cells (bacteria, viruses, archaea, and eukaryotes) as human cells.9 The caecum and proximal colon are the areas of highest microbial biomass. In the healthy intestines, Firmicutes and Bacteroidetes contribute most of the total bacterial species (90% of gut microbiota population), and their ratio (F/B) is often considered a relative estimate of intestinal microbial health (lower F/B ratio) or disease state (higher F/B ratio) in the literature.10 A lower F/B ratio is often a consequence of a decrease in some of the beneficial bacteria that in parallel are replaced by harmful bacteria belonging to the same phyla, mainly Firmicutes. This change in F/B ratio depends on lifestyle components such as diet, physical activity, sleep patterns, medications, and environmental factors including antibiotic use (Figure 1).
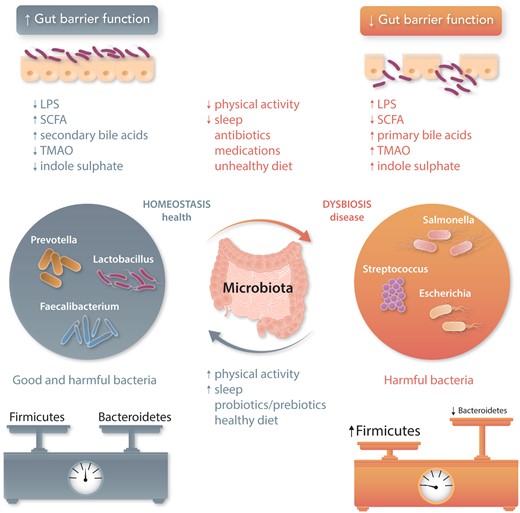
Role of the gut microbiota in health and disease. Homeostasis and dysbiosis of human gut microbiota may increase health (grey font) or promote disease (orange font), respectively. Environmental factors (e.g. physical activity or sleep) can influence homeostatic equilibrium. Normal gut microbiota represent a mixture of microbes with balanced phyla of Firmicutes and Bacteroidetes, whereas dysbiotic gut microbiota show fewer beneficial microbes from Bacteroidetes phylum and more harmful microbes from Firmicutes phylum. Pathological bacterial overgrowth induces inflammation and loss of barrier function that in turn promotes increased translocation of bacterial components and their metabolites, potentially extending into the host’s bloodstream. LPS, lipopolysaccharide; SCFA, short-chain fatty acids; TMAO, trimethylamine N-oxide.
3. Gut microbiota profile in patients with AF
To date, only small observational studies have described changes in gut microbiota in patients with AF11–14 and in patients undergoing AF ablation.15 In one study, there was a difference between patients with persistent AF and controls.14 Gut microbiota diversity did not differ between patients with persistent AF of either short (<12 months) or long duration (≥12 months).14 The above-mentioned small observational studies have not been specifically designed to address the influence of comorbidities and drugs on the changes in gut microbiota and cannot demonstrate a definitive cause–effect relationships (Supplementary material online, Figure S1).
4. Gut dysbiosis and AF risk factors
Recent studies reveal a potential contribution of gut microbiota to the manifestation of human cardiometabolic diseases.16–18 Several AF risk factors, including diabetes,19 obesity,20 hypertension,21 obstructive sleep apnoea,22 chronic obstructive pulmonary disease,23 coronary artery disease,24 and heart failure,25 which have been individually related to AF progression, are associated with gut dysbiosis (Graphical Abstract). However, these observations have not been confirmed in specifically selected AF populations, and the effect of changes in AF risk factors mediated by the modulation of the gut microbiota on AF remains unclear. Alterations of the gut microbiota during the critical early life window, when the immune system is still developing, have been hypothesized to disturb the normal pattern of immunological maturation. Altered immune function can lead to the development of chronic inflammatory and autoimmune disorders including chronic obstructive pulmonary disease26 and diabetes.19 Increased intestinal permeability to structural bacteria ligands aggravates low-grade inflammation and insulin resistance, leading to cardiovascular substrate development.20 Imbalances in specific microbial populations and their corresponding metabolites affect blood pressure,21 immune processes,26 and the autonomic nervous system, thereby promoting the onset of hypertension and obstructive sleep apnoea.22 Additionally, preclinical27,28 and clinical29 studies suggest that diet-induced dysbiosis may modify the pathophysiological responses to risk factors.
5. Gut dysbiosis and AF-promoting mechanisms
A key contribution of gut microbiota to host physiology is to harvest nutrition and energy with simultaneously production of a diverse array of metabolites (Graphical Abstract). The gut microbiota are involved in the fermentation of non-digestible dietary fibres, resulting in short-chain fatty acid (SCFA) production. Other specific products of gut microbiota include trimethylamine (TMA) from dietary choline and carnitine (from meat and dairy products) and indoxyl sulphate from dietary tryptophan (from protein-based food). Gut microbiota enzymes take part in bile acid (BA) metabolism, producing both unconjugated and secondary BAs. All aforementioned gut microbiota-derived metabolites are absorbed across the host gut, influence the immune cells of the gut, and are detectable in the host circulation. They act as signalling molecules and influence important metabolic pathways. In some cases, gut microbiota signalling molecules that are structural component of the microbiota, such as lipopolysaccharide (LPS), are released from the intestinal lumen to the systemic circulation if intestinal integrity fails, for example, as a result of dietary components. Figure 2 and Supplementary material online, Tables S1–S5 summarize the most relevant gut microbiota-derived metabolites, along with the AF-promoting mechanisms that they have been shown to modulate.

The most relevant gut microbiota-derived metabolites along with their suggested AF-promoting mechanisms. Primary BAs (primarily CDCA) activate NADPH oxidase, promoting ROS formation and inducing ATP release, which acts via P2X7 receptors to cause K+ efflux, resulting in NLRP3 inflammasome activation. This activates caspase-1, which generates IL-1β, promoting cardiac inflammation and fibrosis. CDCA may also cause myocyte apoptosis via caspase 9 and caspase 3 and decrease cAMP production via muscarinic M2 receptor activation, altering intracellular Ca2+ homeostasis. Indoxyl sulphate also promotes ROS generation and may increase the expression of pro-inflammatory and profibrotic signalling molecules, promoting cardiac inflammation and fibrosis. TMAO up-regulates NF-κB, which increases the abundance of inflammatory cytokines including IL-1β, IL-6, TNFα, and the synthesis of NGF in atrial ganglionated plexi, which activate the cardiac autonomic nervous system. TMAO also causes oxidative stress and activates the NLRP3 inflammatory and TGFβ1/Smad3 signalling pathways. LPS strongly activates the NLRP3 system, increases the expression and lateralization of connexin-43 protein, and down-regulates L-type Ca2+ channel expression. Choline activates IK, ACh at high concentrations, which could theoretically cause proarrhythmic action potential shortening. The effects of these microbiota-derived metabolites have the potential to cause atrial electrical, autonomic, structural, and Ca2+-handling remodelling that increases the likelihood of AF-promoting ectopic firing and AF-maintaining re-entry, enhancing the susceptibility to AF and its maintenance. Dashed lines indicate potential mechanisms in AF pathogenesis for which there is indirect evidence. Solid lines indicate potential mechanisms in AF pathogenesis for which there is direct evidence. Bold lines indicate mechanisms with evidence for a direct contribution of gut dysbiosis to AF pathogenesis. Further experimental evaluation is needed to confirm or disprove the involvement of all mechanisms except those shown by bold lines. AC, adenylyl cyclase; AF, atrial fibrillation; Apf-1, apoptosis protease-activating factor-1; ATP, adenosine triphosphate; BAs, bile acids; Ca2+, calcium; cAMP, cyclic adenosine monophosphate; CDCA, chenodeoxycholic acid; EGFR, epidermal growth factor receptor; IL, interleukin; IK, Ach, acetylcholine-activated potassium channel; K+, potassium; LPS, lipopolysaccharide; MPTP, mitochondrial permeability transition pore; NADPH, nicotinamide adenine dinucleotide phosphate; NF-κB, nuclear factor kappa-light-chain-enhancer of activated B cells; NGF, nerve growth factor; NLRP3, NACHT, LRR, and PYD domains-containing protein-3; P2RX7, P2X purinoceptor 7; PLB, phospholamban; ROS, reactive oxygen species; RyR2, ryanodine receptor 2; SERCA2a, sarcoendoplasmic reticulum Ca2+-ATPase; SR, sarcoendoplasmic reticulum; TGFβ1, transforming growth factor β1; TGR5, Takeda G-protein-coupled receptor 5; TMAO, trimethylamine N-oxide; TNFα, tumour necrosis factor α.
TMAO is a product of TMA oxidization by liver flavin-containing monooxygenase enzyme30 and the most extensively studied microbial metabolite involved in AF pathogenesis. In canine models, local injection of TMAO activates the atrial autonomic ganglion plexus and promotes arrhythmia, possibly by activating p65 nuclear factor-κB signalling and increasing expression of inflammatory cytokines.31 Clinical data evaluating the association between AF and TMAO are inconclusive. While some studies report that higher TMAO levels are associated with incident AF independently of traditional AF risk factors,32,33 this relationship could not be confirmed in other work.34,35 Nevertheless, marked increases in the microbial genes underlying TMA formation and TMA microbial producers are observed in the intestines of AF patients.36 Although small studies showed that elevated serum TMAO levels were predictive of thromboembolic events in AF patients,37,38 the validity and significance of this association requires further evaluation in additional larger AF populations.
TMAO administration exacerbates doxorubicin-induced cardiac fibrosis39 via activation of the NACHT, LRR, and PYD domains-containing protein-3 (NLRP3) inflammasome, which is an established contributor to AF development.40 Li et al. reported induction of cardiac hypertrophy and expression of hypertrophic markers including atrial natriuretic peptide by TMAO in vivo in Sprague–Dawley rats and in vitro in neonatal ventricular cardiomyocytes.41 While these mechanisms contribute to AF pathophysiology in other contexts, their direct role in mediating TMAO actions on AF remains to be assessed in animal models and AF patients.
In addition, TMAO may enhance the susceptibility to AF by promoting various AF risk factors, such as metabolic syndrome and hypertension via pro-atherosclerotic effects,30,42 renin–angiotensin system regulation,43 and aortic stiffening.44
In a proteomic analysis, choline, which is a TMAO precursor, was more abundant in the atrial appendage and plasma samples from AF patients vs. non-AF subjects.45 Additionally, both plasma and dietary intake of choline were positively associated with later AF risk in a pooled analysis of three prospective cohorts by Zuo et al.46 Choline has been shown to be a weak muscarinic receptor agonist that at high concentrations can activate the acetylcholine-gated potassium current and shorten the effective refractory period, thereby enhancing AF susceptibility.47,48 Whether this mechanism is operative in patients is quite unclear, given the very high choline concentrations required.
Indoxyl sulphate, the most common uraemic toxin, is derived from the metabolism of dietary tryptophan.49 In experimental studies, it increases pulmonary vein and left atrial arrhythmogenesis and reduces sinoatrial node pacemaker activity by causing oxidative stress and dysregulation of cardiomyocyte calcium handling.50 In the clinical setting, high indoxyl sulphate concentrations (≥0.65 μg/mL) independently predict a 3.7-fold risk of AF recurrence.51 Conversely, catheter ablation of AF is associated with reduced indoxyl sulphate serum concentrations, suggesting that AF per se might increase indoxyl sulphate production.52 In animal studies, indoxyl sulphate increases the expression of proinflammatory and profibrotic signalling molecules and causes oxidative stress53 that could contribute to AF.54,55 However, in these experimental studies, the concentrations of indoxyl sulphate used substantially exceed their physiologic range in plasma, making its potential role in AF promotion quite uncertain.
LPS is an endotoxin found in the outer layer of Gram-negative bacteria (in particular Escherichia genera).56 In a canine model, administration of LPS increases the atrial concentration of pro-inflammatory cytokines, thereby increasing connexin 43 expression and causing connexin lateralization.57 LPS down-regulates the expression of L-type calcium channels (α1C and β2 subunits) and abbreviates the effective refractory period.58 Abnormal calcium handling and connexin modulation are potential mechanisms underlying inducible AF.59 Although the direct effects of LPS on AF pathogenesis have not yet been studied, AF patients with increased levels of LPS appear to have a high risk of major adverse cardiovascular events.60,61 Indirectly, LPS may lead to AF through acceleration of atherosclerosis 62 and may induce left ventricular dysfunction58 and heart failure, both of which increase the risk of AF. Positive correlations between LPS levels and 11-dehydro-thromboxane B2 urinary excretion indicate that LPS may represent an important trigger for platelet activation, which can increase thromboembolic risk.61
Primary BAs, such as chenodeoxycholic acid, form bile salts by conjugation to amino acids (taurine or glycine) that are further secreted into the small intestine. Taurine-conjugated BAs can induce changes in membrane potential via cardiac sodium–calcium exchanger stimulation63 and activate muscarinic M2 receptors/acetylcholine-regulated potassium current in cardiomyocytes, which may promote AF.64 Diets high in animal protein favour taurine conjugation of BAs in humans, whereas vegetarian/vegan diets favour glycine conjugation. In the gut, the primary BAs are deconjugated via gut microbiota and bile salt hydrolase produced by gut microbiota in the ileum. They can be further converted by dehydrogenation, dihydroxylation, or epimerization by colonic bacteria to form secondary BAs, including ursodeoxycholic acid. Dysbiotic gut microbiota modulate BA ratios towards reduced concentrations of secondary BAs, including ursodeoxycholic acid, and increase primary BAs such as chenodeoxycholic acid. Chenodeoxycholic acid has been shown to cause atrial cardiomyocyte apoptosis, which may contribute to the evolution of AF-promoting structural remodelling,65 promoting cardiac injury and fibrosis via farnesoid X receptors66 and aggravating the inflammatory process by NLRP3 inflammasome activation.67 On the other hand, ursodeoxycholic acid plays a role in preventing arrhythmia by stabilizing the cell membrane potential.68,69 BAs may also induce AF indirectly by participating in glucose and lipid metabolism, as well as maintaining blood pressure within normal limits through 11β-hydroxysteroid dehydrogenase, which regulates cortisol and aldosterone levels.68
Fermentation of glucose and dietary fibre by colonic microbiota produces SCFAs, in particular acetate (60%), butyrate (20%), and propionate (20%).25 Given the significant contribution of SCFAs to AF-promoting risk factors, SCFAs are potential contributors to AF pathogenesis. SCFAs are responsible for promoting mucus production, thereby improving intestinal barrier function25 and, for some of them, affecting immune regulation by inhibiting histone deacetylases.68 Low SCFA levels contribute to poor metabolite-sensing G-protein-coupled receptor engagement, which impairs gut integrity and facilitates the passage of substances such as LPS into the blood and tissues.21 Moreover, SCFAs differ in their harmful and beneficial properties. Butyrate is thought to exert beneficial metabolic actions via anti-inflammatory70 and insulin sensitivity increasing effects.71 Propionate stimulates glucagon-like peptide-1 and peptide YY release, reducing obesity risk72 and increasing renin release and blood pressure, thus modifying two important AF risk factors.73 Otherwise, acetate contributes to dyslipidemia74 and promotes obesity by enhancing secretion of insulin (by the pancreas) and ghrelin (by the gastric mucosa) via parasympathetic nervous system activation in the brain.75
6. Experimental evidence for a direct role of gut dysbiosis in AF pathogenesis
The strongest evidence for a direct contribution of gut dysbiosis to AF pathogenesis was presented in a very recent study by Zhang et al.76 These authors demonstrated that ageing-associated gut dysbiosis promotes AF partly through increased levels of circulating LPS and glucose, along with enhanced activity of the atrial NLRP3 inflammasome, which leads to atrial fibrosis.76 A possible mechanism of age-related enhanced intestinal permeability is a decrease in mucin production, since mucin provides a protective lining within the gastrointestinal tract that prevents microbes from interacting with epithelial cells and initiating inflammatory responses. Mucin is also a nutrient source for many pathogenic bacterial strains including Bacteroidaceae and Bifidobacteriaceae families, which further enhances ageing-induced gut dysbiosis.77 Of note, faecal microbiota transplantation (FMT) from young (2–3 months old) to old (22–24 months old) rats attenuated atrial fibrosis and the age-related increase in AF susceptibility via a reduction in atrial NLRP3 inflammasome activity, pointing to systemic inflammation as a potential cause. Selective inhibition of both LPS with LPS from photosynthetic bacterium Rhodobacter sphaeroides and NLRP3 inflammasome with MCC950, reduced atrial fibrosis. They also reduced AF susceptibility and duration, supporting the causative role of systemic inflammation in general and of NLRP3 inflammatory signalling in particular as key upstream drivers. These findings are in line with previous78 and recent evidence79 for an important role of sterile inflammation in the development of the AF substrate. There is evidence that cardiomyocyte NLRP3 inflammatory signalling is enhanced in patients with paroxysmal, persistent, and post-operative AF,40,80 as well as in conditions that promote AF (e.g. diabetes and obesity).81,82 Besides causing structural remodelling, up-regulation of the NLRP3 system leads to a reentry-promoting abbreviation of the atrial action potential and to a higher frequency of spontaneous diastolic sarcoplasmic reticulum Ca2+ releases that may cause delayed afterdepolarizations and triggered ectopic activity.40,80,82,83 Although the NLRP3 system appears to play a significant role in mediating gut microbiota effects on AF, further in-depth investigations are required to elucidate the precise cellular and molecular mechanisms and confirm its importance.
7. Strategies to modify gut microbiota composition and metabolites in research and potential clinical intervention
7.1 Direct microbial interventions
There are different interventions that can be used to prevent the detrimental biological effects of dysbiosis. One approach is the direct oral consumption of beneficial live microorganisms (probiotics) or FMT. Alternatively, food ingredients such as fermentable fibres that induce the growth of beneficial microorganisms (prebiotics) can be supplemented to the diet. The most direct approach is the application of gut microbiota-derived metabolites like SCFAs (postbiotics).
7.1.1 Probiotics
Probiotics, mainly Lactobacillus spp. and Bifidobacterium spp., enhance gastrointestinal barrier function, producing anti-microbial factors including SCFAs and bacteriocin, that inhibit pathogen growth, suppress their harmful toxins, and regulate the activity and phenotype of macrophages, natural killer and T cells.84 Probiotics might also provide benefit by acting through dysbiosis-related AF risk factors, including obesity, coronary artery disease, or heart failure,85 presented in Supplementary material online, Table S6. Whether probiotics display protective effects against AF in patients requires future study.
7.1.2 Prebiotics
Prebiotics like galactooligosaccharides increase SCFAs and decrease high-fat-diet-induced LPS production.86 The red wine-derived polyphenol, resveratrol, possesses anti-arrhythmic properties through inhibition of intracellular calcium release.87 In mouse models, resveratrol attenuates atherosclerosis by decreasing TMAO levels and increasing hepatic BA neosynthesis via gut microbiota remodelling (increase in bile salt hydrolase-active bacteria, that is, Lactobacillus and Bifidobacterium). In mice treated with antibiotics, resveratrol neither decreases TMAO levels nor increases hepatic BA synthesis.88 Future clinical studies should test whether resveratrol has probiotic-like properties that could be exploited as a therapeutic option for gut microbiota-related AF.
7.1.3 Synbiotics
Combinations of pre- and probiotics may improve the response to dietary interventions. Moreover, since humans feature an individual-specific gut mucosal colonization resistance to probiotics,89 combined administration of probiotics with prebiotics could prevent the impact of resistant human microbial commensals.
7.1.4 FMT
FMT from a healthy subject into one suffering from dysbiosis-related illness can alleviate or even cure the malady. Conversely, FMT from an atherosclerosis-prone and high TMAO-producing murine strain into an atherosclerosis-resistant, low TMAO-producing murine strain enhances TMAO production and promotes atherosclerosis.90 In another study, transplant of caecal contents from normotensive rats into spontaneously hypertensive rats decreased blood pressure, while normotensive rats developed high blood pressure after FMT from spontaneously hypertensive rats.91 Despite the potential beneficial effects of FMT,91 the precise effects of FMT on the AF substrate and their mechanisms need further dissection. Given the differences between the caecal and faecal microbiomes,92 a direct comparison between FMT and caecal content transfer might provide additional invaluable insights.
7.2 Diet
In humans, diet contributes to daily fluctuations in gut microbiota composition.93 Permeability changes in the gut due to high-fat and high-sugar diets allow bacterial LPS to translocate from the gut into the systemic circulation, producing systemic endotoxemia. Polyphenols enhance the growth of Bacteroides genera that produce LPS with reduced endotoxic activity compared with LPS from other bacteria, thereby decreasing the inflammatory burden.94 Decreased consumption of fibre-rich food is associated with a reduction in SCFA-producing bacteria, while high-fibre diet and acetate supplementation reduce blood pressure and hypertensive end-organ damage in a salt-sensitive mouse model.27 Diet affects TMAO levels by enhancing particular bacteria responsible for TMA-to-TMAO conversion.95 Cruciferous vegetables (e.g. cabbage, broccoli) inhibit the activity of the flavin containing dimethylaniline monoxygenase enzyme that plays a vital role in converting TMA to TMAO. In animal studies, a high-choline diet (0.1–1.0 weight per cent) increased circulating TMAO in a dose-dependent manner,96 although this could not be confirmed in human studies.97 In addition, TMAO production following ingestion of L-carnitine is greater in omnivorous human subjects compared to vegans/vegetarians.98 Therefore, dietary habits alter the ability to synthesize TMA/TMAO from dietary L-carnitine, likely by modifying the composition or enzymatic activity of gut microbiota.30 Thus, targeting the TMA synthesis pathways in the gut might be a clinically valuable approach to reduce TMAO effects on the AF substrate. However, these ideas remain speculative pending confirmation in future studies.
Inhibition of TMA and TMAO production by 3,3-dimethyl-1-butanol significantly reduces adverse ventricular remodelling and improves haemodynamic parameters.99 These findings suggest that diet-derived nutrients could diminish the risk of AF via direct effects on ventricular remodelling and associated haemodynamic changes that affect the atria, rather than through decreases in AF risk factors like obesity and dyslipidaemia. Of note, 3,3-dimethyl-1-butanol is an extra-virgin olive oil component100 highly consumed in Mediterranean diet. Other potential TMAO-lowering drugs are resveratrol, which inhibits TMA production by gut microbiota commensals, and meldonium, which is thought to reduce the conversion of carnitine to TMA.88,101 Meldonium is approved in Eastern European countries as an anti-anginal drug and enhances the renal excretion of TMAO, hence decreasing its plasma concentrations.102 Short-term (1–12 week) dietary studies assessing effects on gut microbiota note only transient changes that lasted from the time in dietary alteration93 to 3 days post-intervention.103 Within 24–48 hours93 of dietary intervention, rapid but transient changes occur in gut microbiota composition; however, core gut microbiota profiles remain stable even during the 10-day time period of changed diet habits.104,105 As populations of microbes can double within an hour, it is possible that intermittent fasting could manipulate the composition of gut microbiota.106 Our current understanding of how dietary habits cause long-term changes in gut microbiota is limited, and the temporal stability of gut microbiota over a 6-month period associates with dietary pattern.107,108 The duration of any dietary intervention required to produce long-term effects on gut microbiota requires further investigation in well-designed randomized clinical trials that include prospective and standardized analyses of microbiota.
7.3 Drug–gut microbiota interactions
Numerous studies have demonstrated a bidirectional interaction between drugs and gut microbiota, which may also modulate drug metabolism.109,110 For example, depending on strain (with the DSM2243 strain showing the strongest effects) Eggerthella lenta inactivates digoxin by reducing the lactone ring to produce an inactive product.111 In addition, gut microbial enzyme activity is involved in first-pass clearance of some cardiovascular drugs, including calcium channel blockers112,113 and non-steroidal anti-inflammatory drugs,114,115 increasing the bioavailability of these drugs when patients are pretreated with antibiotics. Conversely, many clinically used drugs can enhance116 or reduce the growth rate of gut microbiota and/or gut microbiota-derived metabolites.117 Metagenomic sequencing of stool samples in 1135 participants from a Dutch cohort study revealed that the use of several cardiovascular agents, including statins, anti-thrombotic drugs, beta-blockers, and angiotensin-converting enzyme inhibitors, had a significant impact on the gut microbiome.118 Some of these associations have been supported by a British study of 2700 participants, which found an association of several bacterial taxa with beta-blocker and alpha-blocker use.119 Pirfenidone, duloxetine, methimazole, clozapine, alosetron, and enalapril inhibit flavin monooxygenase and may reduce TMAO levels.120 Three of the most commonly prescribed statins (atorvastatin, rosuvastatin, and simvastatin) may also modulate gut microbiota through interactions with BA metabolism pathways.121 Antibiotics promote gut dysbiosis, causing loss of diversity, increases in the proportion of potentially pathogenic bacteria, and imbalances in the proportion of bacterial phyla. A recent systematic review that evaluated the effects of antibiotics on gut microbiota showed that the gut dysbiotic effects of antibiotics like clindamycin, ciprofloxacin, metronidazole, and clarithromycin may last for several years.122
Overall, the different classes of antibiotics have distinct and complex effects on gut microbiota.123 For instance, lincosamide antibiotics (like clindamycin) may decrease the diversity of Bacteroides, while β-lactam antibiotics (e.g. penicillin v, amoxicillin, ampicillin/sulbactam, cephalosporins) decrease the growth of Actinobacteria and Firmicutes, but increase the growth of Proteobacteria and Bacteroidetes.123 Macrolide antibiotics (e.g. clarithromycin and erythromycin) decrease the growth of Firmicutes (mainly Lactobacilli) and increase the abundance of Proteobacteria and Bacteroidetes.123 Glycopeptide antibiotics (e.g. vancomycin) reduce the abundance of Firmicutes but increase the growth of Proteobacteria.123 Finally, fluoroquinolones (e.g. ciprofloxacin and levofloxacin) suppress the growth of Gram-positive and Gram-negative anaerobic bacteria, but increase the abundance of aerobic Gram-positive bacteria.123 Oral administration of antibiotics (e.g. ciprofloxacin, vancomycin, metronidazole) may reduce TMAO production by inhibiting the conversion of L-carnitine to TMA.120 Antibiotics decrease the production of chenodeoxycholic acid and increase the plasma levels of cholic acid which changes the BA pool.124,125 Finally, in a small study, antibiotics reduced the biosynthesis of amino acids126 including glutamate and taurine, an effect that was associated with paroxysmal AF occurrence.127 Antibiotic therapy can alleviate glucose intolerance128 and decrease the concentrations of cholesterol,129 potentially reducing AF risk factor burden.2
8. Assessment of gut microbiota composition
Methods used to differentiate among microbial strains are divided into culture-based techniques and high-throughput sequencing. Culture-based techniques include standard plate count and the most probable number (based on the principle of extinction dilution) techniques, and more sophisticated approaches like cell encapsulation and bioreactors. The commonly used high-throughput sequencing methods to assess microbiome are amplicon, metagenomic sequencing, and metatranscriptomic sequencing (Table 1). In amplicon sequencing, a single, highly conserved genomic locus is targeted for polymerase chain reaction amplification to identify operational taxonomic units. The major marker genes used in amplicon sequencing include 16S ribosome DNA for prokaryotes and 18S ribosome DNA and internal transcribed spacers for eukaryotes. Shotgun metagenomics can identify strains, and provide genome content, functional capacity, and some genome assembly for organisms of even modest abundance. Metatranscriptomic sequencing can profile mRNAs in a microbial community, quantify gene expression levels, and provide a snapshot for functional exploration of a microbial community in situ.130 Along with methods of profiling the gut microbiota, metabolomic analysis of small-molecule metabolites in cells, tissues, and whole organisms in biofluids is useful. Nuclear magnetic resonance and mass spectrometry techniques, often coupled with separation methods including gas-chromatography or liquid-chromatography, have been applied for large-scale profiling of metabolites.131 However, there is still no agreement on how best to assess the composition of the gut microbiota or dietary patterns.
Advantages and limitations of selected high-throughput sequencing methods used in microbiome research (A) and methods for metabolic profiling (B)
A. High-throughput sequencing methods . | |||
---|---|---|---|
Method . | 16S/18S rRNA amplicon gene sequencing . | Whole metagenomic sequencing . | Meta-transcriptomes . |
Molecule level . | DNA . | DNA . | mRNA . |
Description | Identify operational taxonomic units or high-confidence amplicon sequence variants | Identify strains and their functional capacity broadly based on their single-nucleotide variants or structural variants (gene gain and loss events) | Amplify the effects of gene gains or losses, or the effects of small variants that result in differential expression |
Advantages | • Quick analysis • Possibility to apply this technique on low-biomass samples • Applicable to samples contaminated by host DNA | • Taxonomic resolution to species or strain level • Functional potential • Uncultured microbial genome | • Identify live microbes • Evaluate microbial activity • Transcript level response |
Limitations | • Polymerase chain reaction and primer biases • Resolution limited to genus level • False positive (contamination) in low-biomass samples | • Expensive • Time-consuming in bioinformatics technology analysis • Host-derived contamination | • Expensive • Complex in sequencing, sample collection, and analysis • Host mRNA and rRNA contamination |
B. Metabolic profiling methods | |||
Method | Mass spectrometry (MS) | Nuclear magnetic resonance (NMR) spectroscopy | |
Liquid-chromatography (LC) | Gas-chromatography (GC) | ||
Advantages | • High sensitivity (pM–μM) • High resolution and dynamic range | • Small sample volume (0.01–0.1 mL) • No sample preparation • Quantification + 5%; no standard needed; linear response • Simple data structure • Rapid profiling (<5min) • Highly quantitative and reproducible • Non-destructive detection | |
• Small sample volume(0.01–0.1 mL) • Higher number of detectable metabolites (vs. GC) | • Modest sample volume (0.1–0.2 mL) • Higher resolution (vs. LC) | ||
Limitations | • Destructive (sample not recoverable) • Requires sample separation[desalting, deproteinization, extraction (LC); extraction and derivatization (GC)] • Quantification + 10%; individual isotope-labelled internal standard needed; response dependent on ionization and matrix • Data structure challenging • Slow profiling (20–40 min per sample) | • Low sensitivity (μM–mM); peak overlap • Expensive • Cannot detect salts, inorganic ions, non-protonated compounds | |
• Lower resolution (vs. GC) • Ion depression effect | • Lower number of detectable metabolites (vs. LC) • Complex sample preparation |
A. High-throughput sequencing methods . | |||
---|---|---|---|
Method . | 16S/18S rRNA amplicon gene sequencing . | Whole metagenomic sequencing . | Meta-transcriptomes . |
Molecule level . | DNA . | DNA . | mRNA . |
Description | Identify operational taxonomic units or high-confidence amplicon sequence variants | Identify strains and their functional capacity broadly based on their single-nucleotide variants or structural variants (gene gain and loss events) | Amplify the effects of gene gains or losses, or the effects of small variants that result in differential expression |
Advantages | • Quick analysis • Possibility to apply this technique on low-biomass samples • Applicable to samples contaminated by host DNA | • Taxonomic resolution to species or strain level • Functional potential • Uncultured microbial genome | • Identify live microbes • Evaluate microbial activity • Transcript level response |
Limitations | • Polymerase chain reaction and primer biases • Resolution limited to genus level • False positive (contamination) in low-biomass samples | • Expensive • Time-consuming in bioinformatics technology analysis • Host-derived contamination | • Expensive • Complex in sequencing, sample collection, and analysis • Host mRNA and rRNA contamination |
B. Metabolic profiling methods | |||
Method | Mass spectrometry (MS) | Nuclear magnetic resonance (NMR) spectroscopy | |
Liquid-chromatography (LC) | Gas-chromatography (GC) | ||
Advantages | • High sensitivity (pM–μM) • High resolution and dynamic range | • Small sample volume (0.01–0.1 mL) • No sample preparation • Quantification + 5%; no standard needed; linear response • Simple data structure • Rapid profiling (<5min) • Highly quantitative and reproducible • Non-destructive detection | |
• Small sample volume(0.01–0.1 mL) • Higher number of detectable metabolites (vs. GC) | • Modest sample volume (0.1–0.2 mL) • Higher resolution (vs. LC) | ||
Limitations | • Destructive (sample not recoverable) • Requires sample separation[desalting, deproteinization, extraction (LC); extraction and derivatization (GC)] • Quantification + 10%; individual isotope-labelled internal standard needed; response dependent on ionization and matrix • Data structure challenging • Slow profiling (20–40 min per sample) | • Low sensitivity (μM–mM); peak overlap • Expensive • Cannot detect salts, inorganic ions, non-protonated compounds | |
• Lower resolution (vs. GC) • Ion depression effect | • Lower number of detectable metabolites (vs. LC) • Complex sample preparation |
Advantages and limitations of selected high-throughput sequencing methods used in microbiome research (A) and methods for metabolic profiling (B)
A. High-throughput sequencing methods . | |||
---|---|---|---|
Method . | 16S/18S rRNA amplicon gene sequencing . | Whole metagenomic sequencing . | Meta-transcriptomes . |
Molecule level . | DNA . | DNA . | mRNA . |
Description | Identify operational taxonomic units or high-confidence amplicon sequence variants | Identify strains and their functional capacity broadly based on their single-nucleotide variants or structural variants (gene gain and loss events) | Amplify the effects of gene gains or losses, or the effects of small variants that result in differential expression |
Advantages | • Quick analysis • Possibility to apply this technique on low-biomass samples • Applicable to samples contaminated by host DNA | • Taxonomic resolution to species or strain level • Functional potential • Uncultured microbial genome | • Identify live microbes • Evaluate microbial activity • Transcript level response |
Limitations | • Polymerase chain reaction and primer biases • Resolution limited to genus level • False positive (contamination) in low-biomass samples | • Expensive • Time-consuming in bioinformatics technology analysis • Host-derived contamination | • Expensive • Complex in sequencing, sample collection, and analysis • Host mRNA and rRNA contamination |
B. Metabolic profiling methods | |||
Method | Mass spectrometry (MS) | Nuclear magnetic resonance (NMR) spectroscopy | |
Liquid-chromatography (LC) | Gas-chromatography (GC) | ||
Advantages | • High sensitivity (pM–μM) • High resolution and dynamic range | • Small sample volume (0.01–0.1 mL) • No sample preparation • Quantification + 5%; no standard needed; linear response • Simple data structure • Rapid profiling (<5min) • Highly quantitative and reproducible • Non-destructive detection | |
• Small sample volume(0.01–0.1 mL) • Higher number of detectable metabolites (vs. GC) | • Modest sample volume (0.1–0.2 mL) • Higher resolution (vs. LC) | ||
Limitations | • Destructive (sample not recoverable) • Requires sample separation[desalting, deproteinization, extraction (LC); extraction and derivatization (GC)] • Quantification + 10%; individual isotope-labelled internal standard needed; response dependent on ionization and matrix • Data structure challenging • Slow profiling (20–40 min per sample) | • Low sensitivity (μM–mM); peak overlap • Expensive • Cannot detect salts, inorganic ions, non-protonated compounds | |
• Lower resolution (vs. GC) • Ion depression effect | • Lower number of detectable metabolites (vs. LC) • Complex sample preparation |
A. High-throughput sequencing methods . | |||
---|---|---|---|
Method . | 16S/18S rRNA amplicon gene sequencing . | Whole metagenomic sequencing . | Meta-transcriptomes . |
Molecule level . | DNA . | DNA . | mRNA . |
Description | Identify operational taxonomic units or high-confidence amplicon sequence variants | Identify strains and their functional capacity broadly based on their single-nucleotide variants or structural variants (gene gain and loss events) | Amplify the effects of gene gains or losses, or the effects of small variants that result in differential expression |
Advantages | • Quick analysis • Possibility to apply this technique on low-biomass samples • Applicable to samples contaminated by host DNA | • Taxonomic resolution to species or strain level • Functional potential • Uncultured microbial genome | • Identify live microbes • Evaluate microbial activity • Transcript level response |
Limitations | • Polymerase chain reaction and primer biases • Resolution limited to genus level • False positive (contamination) in low-biomass samples | • Expensive • Time-consuming in bioinformatics technology analysis • Host-derived contamination | • Expensive • Complex in sequencing, sample collection, and analysis • Host mRNA and rRNA contamination |
B. Metabolic profiling methods | |||
Method | Mass spectrometry (MS) | Nuclear magnetic resonance (NMR) spectroscopy | |
Liquid-chromatography (LC) | Gas-chromatography (GC) | ||
Advantages | • High sensitivity (pM–μM) • High resolution and dynamic range | • Small sample volume (0.01–0.1 mL) • No sample preparation • Quantification + 5%; no standard needed; linear response • Simple data structure • Rapid profiling (<5min) • Highly quantitative and reproducible • Non-destructive detection | |
• Small sample volume(0.01–0.1 mL) • Higher number of detectable metabolites (vs. GC) | • Modest sample volume (0.1–0.2 mL) • Higher resolution (vs. LC) | ||
Limitations | • Destructive (sample not recoverable) • Requires sample separation[desalting, deproteinization, extraction (LC); extraction and derivatization (GC)] • Quantification + 10%; individual isotope-labelled internal standard needed; response dependent on ionization and matrix • Data structure challenging • Slow profiling (20–40 min per sample) | • Low sensitivity (μM–mM); peak overlap • Expensive • Cannot detect salts, inorganic ions, non-protonated compounds | |
• Lower resolution (vs. GC) • Ion depression effect | • Lower number of detectable metabolites (vs. LC) • Complex sample preparation |
9. Gaps in knowledge and future directions
The management of AF is built on three pillars: (i) anti-coagulation, (ii) symptom control, and (iii) comorbidity/cardiovascular risk factor management.2 The awareness that gut microbiota composition can contribute to the evolution of cardiovascular disease, including atrial cardiomyopathy, may lead to the development of novel therapeutic opportunities for AF management.132 These might include the suppression of risk factors promoting AF substrate development as well targeting the AF substrate itself. Nevertheless, many important issues need further clarification before considering gut microbiota as a contributory factor to AF that can be targeted, as summarized in Table 2. Mechanistically, it is still unclear to what extent and how alterations in gut microbiota contribute to AF. The effects of changes in gut microbiota and associated metabolites on cardiac electrophysiology and mechanisms that promote AF are poorly characterized. It remains to be determined precisely to what extent the gut microbiota controls AF risk and whether modulation of the gut microbiota affects arrhythmia risk via direct effects on AF-controlling mechanisms or via modification of AF-promoting risk factors. Studies in animal models and human tissue samples, along with human in vivo investigation, are needed to better define the causal relationship between gut microbiota and AF risk. Human inducible pluripotent stem-cell-derived cardiomyocytes (iPSC-CMs) are being increasingly used as models of heart disease, and protocols have been developed to preferentially drive iPSC differentiation towards an atrial cardiomyocyte or even tissue phenotype.133 Thus, testing the individual effects of gut microbiota components on the function of human iPSC-CMs might provide valuable insights into the precise proarrhythmic effects of individual gut microbiota components. To date, studies of gut microbiota in AF have mostly focused on the identification of bacteria, ignoring fungi, viruses, archaea, and protists as potentially relevant components of gut microbiota. The role of non-bacterial microorganisms, individual or whole communities, for AF development warrants further study. Issues around extracting, sequencing, and identifying microorganisms with smaller DNAs, such as bacteriophages, remain a challenge. Besides focusing on the role of single microbes for AF, it is important to study the combined effects of different communities of microbes on gut microbiota. Future investigations should also address the possibility that specific probiotics and post-biotics affect AF in humans and consider whether their supplementation can be incorporated in future risk factor modification programmes for the primary and secondary preventions of AF.
Knowledge gap . | Future directions . |
---|---|
Direct vs. indirect (via AF-promoting risk factors) proarrhythmic effects of gut microbiota |
|
Impact of non-bacterial microorganisms in AF | Well-characterized cohorts of participants with and without AF with prospective metagenomic analysis of archaea, fungi, bacteriophages, viruses |
Importance of individual bacteria vs. whole bacterial communities to reduce AF risk | Ingestion of specific bacteria vs. communities in germ-free animal models134 |
Impact of gut microbiota changes and of specific bacteria on the determinants of AF occurrence | Ingestion of specific bacteria in germ-free animal models134 and assessment of the atrial fibroblast function, cardiomyocyte electrical properties including afterdepolarization, ionic currents, connexin expression and distribution, calcium handling; tissue structure and function including atrial dimensions, function and fibrosis, histopathology; adipocyte properties and adipose tissue distribution |
Effect of specific antibiotics/probioticsa in AF | Multicentre clinical trials of well-characterized participants treated with the same antibiotics/probiotics |
Effects of post-biotics (e.g. SCFAs) in AF | Animal134 and clinical studies to delineate the role of particular post-biotic (e.g. SCFAs) in AF. |
Cell type contributing to NLRP3 inflammasome-mediated AF risk | Animal model134 and cell-culture-based studies to delineate the role of particular cells (e.g. cardiomyocytes, fibroblasts, adipocytes, immune cells) in which NLRP3 inflammasome contributes to AF risk |
Knowledge gap . | Future directions . |
---|---|
Direct vs. indirect (via AF-promoting risk factors) proarrhythmic effects of gut microbiota |
|
Impact of non-bacterial microorganisms in AF | Well-characterized cohorts of participants with and without AF with prospective metagenomic analysis of archaea, fungi, bacteriophages, viruses |
Importance of individual bacteria vs. whole bacterial communities to reduce AF risk | Ingestion of specific bacteria vs. communities in germ-free animal models134 |
Impact of gut microbiota changes and of specific bacteria on the determinants of AF occurrence | Ingestion of specific bacteria in germ-free animal models134 and assessment of the atrial fibroblast function, cardiomyocyte electrical properties including afterdepolarization, ionic currents, connexin expression and distribution, calcium handling; tissue structure and function including atrial dimensions, function and fibrosis, histopathology; adipocyte properties and adipose tissue distribution |
Effect of specific antibiotics/probioticsa in AF | Multicentre clinical trials of well-characterized participants treated with the same antibiotics/probiotics |
Effects of post-biotics (e.g. SCFAs) in AF | Animal134 and clinical studies to delineate the role of particular post-biotic (e.g. SCFAs) in AF. |
Cell type contributing to NLRP3 inflammasome-mediated AF risk | Animal model134 and cell-culture-based studies to delineate the role of particular cells (e.g. cardiomyocytes, fibroblasts, adipocytes, immune cells) in which NLRP3 inflammasome contributes to AF risk |
NLRP3, NOD-, LRR-, and pyrin domain-containing protein 3; SCFAs, short chain fatty acids.
AF, atrial fibrillation; FMT, faecal microbiota transplantation.
For a list of probiotics that might affect AF, see Supplementary material online, Table S6, which lists the probiotics that have been shown to affect cardiovascular risk factors for AF. None have yet been evaluated in AF per se.
Knowledge gap . | Future directions . |
---|---|
Direct vs. indirect (via AF-promoting risk factors) proarrhythmic effects of gut microbiota |
|
Impact of non-bacterial microorganisms in AF | Well-characterized cohorts of participants with and without AF with prospective metagenomic analysis of archaea, fungi, bacteriophages, viruses |
Importance of individual bacteria vs. whole bacterial communities to reduce AF risk | Ingestion of specific bacteria vs. communities in germ-free animal models134 |
Impact of gut microbiota changes and of specific bacteria on the determinants of AF occurrence | Ingestion of specific bacteria in germ-free animal models134 and assessment of the atrial fibroblast function, cardiomyocyte electrical properties including afterdepolarization, ionic currents, connexin expression and distribution, calcium handling; tissue structure and function including atrial dimensions, function and fibrosis, histopathology; adipocyte properties and adipose tissue distribution |
Effect of specific antibiotics/probioticsa in AF | Multicentre clinical trials of well-characterized participants treated with the same antibiotics/probiotics |
Effects of post-biotics (e.g. SCFAs) in AF | Animal134 and clinical studies to delineate the role of particular post-biotic (e.g. SCFAs) in AF. |
Cell type contributing to NLRP3 inflammasome-mediated AF risk | Animal model134 and cell-culture-based studies to delineate the role of particular cells (e.g. cardiomyocytes, fibroblasts, adipocytes, immune cells) in which NLRP3 inflammasome contributes to AF risk |
Knowledge gap . | Future directions . |
---|---|
Direct vs. indirect (via AF-promoting risk factors) proarrhythmic effects of gut microbiota |
|
Impact of non-bacterial microorganisms in AF | Well-characterized cohorts of participants with and without AF with prospective metagenomic analysis of archaea, fungi, bacteriophages, viruses |
Importance of individual bacteria vs. whole bacterial communities to reduce AF risk | Ingestion of specific bacteria vs. communities in germ-free animal models134 |
Impact of gut microbiota changes and of specific bacteria on the determinants of AF occurrence | Ingestion of specific bacteria in germ-free animal models134 and assessment of the atrial fibroblast function, cardiomyocyte electrical properties including afterdepolarization, ionic currents, connexin expression and distribution, calcium handling; tissue structure and function including atrial dimensions, function and fibrosis, histopathology; adipocyte properties and adipose tissue distribution |
Effect of specific antibiotics/probioticsa in AF | Multicentre clinical trials of well-characterized participants treated with the same antibiotics/probiotics |
Effects of post-biotics (e.g. SCFAs) in AF | Animal134 and clinical studies to delineate the role of particular post-biotic (e.g. SCFAs) in AF. |
Cell type contributing to NLRP3 inflammasome-mediated AF risk | Animal model134 and cell-culture-based studies to delineate the role of particular cells (e.g. cardiomyocytes, fibroblasts, adipocytes, immune cells) in which NLRP3 inflammasome contributes to AF risk |
NLRP3, NOD-, LRR-, and pyrin domain-containing protein 3; SCFAs, short chain fatty acids.
AF, atrial fibrillation; FMT, faecal microbiota transplantation.
For a list of probiotics that might affect AF, see Supplementary material online, Table S6, which lists the probiotics that have been shown to affect cardiovascular risk factors for AF. None have yet been evaluated in AF per se.
10. Conclusions
Intestinal dysbiosis has been associated with a wide range of disease conditions. There are multiple lines of evidence suggesting that changes in the gut microbiota may be an important predisposing factor for AF occurrence. Intestinal dysbiosis is a potentially targetable factor that might provide novel treatment avenues for AF prevention. Well-designed mechanistic research and prospective intervention studies targeting gut dysbiosis are crucial to determine the nature and mechanisms of the gut dysbiosis–AF relationship and reveal the plausibility of targeting the intestinal microbiota for primary and secondary preventions of AF.
Supplementary material
Supplementary material is available at Cardiovascular Research online.
Authors’ contributions
All authors have been involved in conception and design or analysis and interpretation of data, or both, drafting of the manuscript or revising it critically for important intellectual content.
Funding
Canadian Institutes of Health Research (148401 to S.N.) and Heart and Stroke Foundation of Canada (18-0022032 to S.N.). National Institutes of Health (R01HL136389, R01HL131517, and R01HL089598 to D.D.), the German Research Foundation (DFG, Do 769/4-1 to D.D.), and the European Union (large-scale integrative project MEASTRIA, No. 965286 to D.D.).
Data Availability
No new data were generated or analysed in support of this research.
References
Author notes
Dobromir Dobrev, Dominik Linz contributed equally and share senior authorship.
Conflict of interest: The authors have no conflict of interest.