-
PDF
- Split View
-
Views
-
Cite
Cite
Libia Alejandra García-Flores, Cara L Green, Of Mice and Men: Impacts of Calorie Restriction on Metabolomics of the Cerebellum, The Journals of Gerontology: Series A, Volume 76, Issue 4, April 2021, Pages 547–551, https://doi.org/10.1093/gerona/glab041
- Share Icon Share
Abstract
The main purpose of research in mice is to explore metabolic changes in animal models and then predict or propose potential translational benefits in humans. Although some researchers in the brain research field have mentioned that the mouse experiments results still lack the complex neuroanatomy of humans, caution is required to interpret the findings. In mice, we observed in article seventeenth of the series of the effects of graded levels of calorie restriction, metabolomic changes in the cerebellum indicated activation of hypothalamocerebellar connections driven by hunger responses. Therefore, the purpose of the current perspective is to set this latest paper into a wider context of the physiological, behavioral, and molecular changes seen in these mice and to compare and contrast them with previous human studies.
There is strong evidence accumulated over many decades that calorie restriction (CR) reliably increases life span and healthspan across multiple organisms (1,2). Nevertheless, the molecular mechanisms underlying the impact of CR in humans and animal models are not well understood. In the present issue of The Journal of Gerontology, Series A: Biological Sciences and Medical Sciences, Green et al. (3) highlight changes in the cerebellar metabolome of male mice under graded levels of CR. This is the latest study in a series that have concerned the responses of the same individual mice to graded CR, including comprehensive studies of their morphology (4), behavior (5,6), and physiology (7). This series of publications has elucidated a broad range of changes in the metabolic behavior of these mice when exposed to CR. However, one of the main purposes of exploring metabolic changes in animal models is to predict or propose potential translational benefits in humans. Therefore, the purpose of the current perspective is to set this latest paper into a wider context of the physiological, behavioral, and molecular changes seen in these mice, and to compare and contrast them with previous human studies. We are conscious that the changes in the cerebellum metabolome of these mice cannot be directly linked to humans since there are still many molecular and physiological mechanisms that are scarcely known and not well understood. We carried out a robust and accurate analysis, and given the lack of research on the effects of graded CR on the cerebellum, here we propose some ideas to explain our results and link them with research in humans. Thus, it is our ambition to allow the reader to infer and raise questions on the manner in which cerebellar function in humans may respond to graded CR, given what is already known and what we have discovered in mice.
A Quick Overview of the Field
At present, research on the brain has focused on resolving the paradox of failures in the use of the mouse for preclinical studies, despite conserved structure across mammals (8). Hodge et al. (8) suggested that the magnitude of differences between humans and mice means that profiling of more closely related nonhuman primates is necessary to study many aspects of human brain structure and function; however, due to ethical concerns, the high costs of housing, and the relatively long life span of nonhuman primates relative to other model organisms, this is often not a practical option. Nevertheless, with expanding molecular technologies, the use of mouse models has shown great promise for accelerating mechanistic understanding of brain evolution, aging, and disease. The use of metabolomic technologies is a good example of this, and its use in the studies of human and mouse brain function is gaining a significant attention. Despite the many advantages of this technique, it should be kept in mind that there remain real limitations, primarily the inaccessibility of the extraction of the samples in humans, in addition to its unsuitability for longitudinal measurements (9,10). While biofluid metabolite profiling of cerebrospinal fluid, plasma, and urine is more easily accessible and can be measured repeatedly, their limitations are well recognized (10,11). One of the obstacles to using biofluids to reflect metabolomic changes in nervous system pathobiology is the blood–brain barrier, which serves to limit the passage of metabolites between the brain and the rest of the body (9,10). It must be emphasized, however, that there are potentially useful biomarkers that can be detected in biofluid samples, such as those indicating brain oxidative lipid damage; these have been associated with oxidative damage in both gray and white brain matter (F4-neuroprostanes and F2-dihomo-isoprostanes, respectively) in both human and animal models (11). Despite these limitations, investigations of brain function using animal models are currently unavoidable if we are to obtain data directly on brain tissue, due to the medical and ethical implications of sampling live human brain tissue. Hence, such studies provide a valuable source of information, which can deepen our understanding of the molecular mechanisms that underpin cross-talk between the brain and the rest of the body and begin to develop the necessary medical interventions when these networks are dysregulated (9). While the use of animal models as a proxy for studying the human brain is essential, results from mouse experiments still lack the complex neuroanatomy of humans, and, therefore, caution is required in the interpretation of the findings (8,12,13).
Aside from the ethical and technical complications brought about through brain studies, there are several experimental difficulties that hound nutritional intervention studies at the point where we attempt to translate them into humans. In particular for human CR studies challenges include strict and life-long compliance with these regimens, which is a much more complex affair in humans than in mice, and also has negative side-effects such as loss of libido, a feeling of cold, and a restriction in choice (14). Recently, however, researchers have investigated other types of dietary restriction and pharmacological interventions such as “CR mimetics,” in an effort to circumvent the negative side-effects and exploit the advantages of CR for the benefit of human health during aging (1,14,15). CR mimetics mimic CR’s benefits at the molecular, cellular, and physiological levels, leading to a health-promoting effect (15). For example, a recent study demonstrated with pharmacological approaches (using NG-nitro-l-arginine ethyl ester) that nitric oxide (NO) produced through the citrulline–NO pathway promotes CR-triggered hypothermia and also that leucine enkephalin directly controls core body temperature when exogenously injected into the hypothalamus (16). Interestingly, our study on the graded impact of CR on the mouse cerebellum also found that the citrulline–NO pathway was significantly associated with the level of CR and longevity (3). Furthermore, in a previous analysis, we have suggested that reduced body temperature may be an integral causal aspect of the CR effect on energy expenditure and energy shortfall (17). There are also compounds from nature that represent an attractive source of (potential) CR mimetics—for example, polyphenolic compounds (organic compounds mostly found in fruits, vegetables, and drinks, such as coffee, tea, and wine). Specifically, resveratrol (polyphenolic phytoalexin) has been linked to neuroprotection (14). However, it has also been mentioned that although the beneficial effects of CR mimetics on life span and health have been extensively highlighted, limitations persist because it remains challenging to implement long-term diet regimens in humans (15).
Can We Use Animal Models to Predict the Human Cerebellar Response to CR?
It is worth noting that some studies have described similar results between mice and humans in the context of the brain, although broadly, they found large differences. One of these studies (8) compared the cortex in humans and mice and found that most of the cell types identified in humans had corresponding cell types in mice. Regarding the cerebellum, it has been suggested that across the life span, cerebellar development in the early period shares similarities across humans, nonhuman primates, and mice. However, differences emerge while development progresses, as revealed by cellular and molecular analyses (13). For example, the rhombic lip (one of 2 zones of neurogenesis) persists longer during cerebellar development in humans than in either the mouse or the macaque and generates a pool of neural progenitor cells (12). These studies have, therefore, highlighted that while similarities do exist, aging and development play an essential role in how we analyze and compare brain studies in humans and mice.
The cerebellum is most commonly associated with motor control and sensory functions. Moreover, research also showed markedly different responses between predictable and unexpected rewards in the cerebellum, suggesting it is engaged in more complex cognitive processing than previously believed (18). However, it also has strong links with the hypothalamus and has more recently been thought to play a role in nutritional regulation and adiposity (Figure 1, item number 2) (3). Due to this potential linkage, the cerebellum is an important brain region to consider when assessing the possible impact of CR on the brain. Figure 1 provides more detailed information about CR and the cerebellum (indicated by numbers) as well as findings in our mice (indicated by letters). In the new study by Green et al. (3), we studied the cerebellar metabolome response of male mice exposed to graded levels of CR. Restriction was started at 20 weeks of age, approximately equivalent to early human adulthood and close to the time when mice reach skeletal maturity. Using this start time avoids the potential impact of CR on development (4). It has been previously suggested that, with age, increasing oxidative stress may be a causal factor of senescence-related loss of brain function in mice (14,19,20). It has also been widely reported that tissues age incongruously to each other (21); in the case of the central nervous system, it was seen in mice that aging impacted the various anatomical structures to a different degree, as each structure has a different resistance to endogenous oxidative stress (22). In humans, the cerebellum was the most protected region in the brain with age since this region accumulates less oxidative damage than other brain regions (21). Although there is some evidence suggesting that CR relieves age-associated oxidative stress in the brains of mice (1,2,23), it was not possible to explore oxidative stress directly in the cerebellum of our mice (3). Thus, it is uncertain whether different levels of CR had a graded impact on brain oxidative stress. However, we observed profound changes in physical activity (24) and behavior (5) due to CR. Physical activity has been linked to improvements in maze tasks and increased exploratory activity in rats, but also improved cerebellar mitochondrial respiration and decreased oxidative stress and apoptotic markers, which has implications for improved mitochondrial plasticity in the cerebellum with exercise (25).
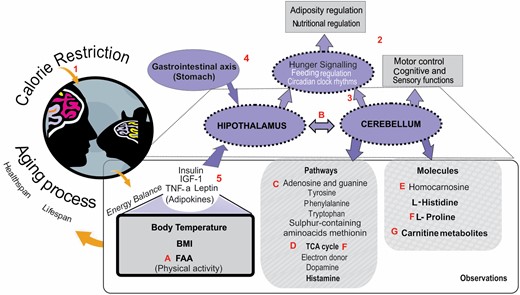
The main observations made in Green et al. (3). The numbers are information according to literature, and the letters are the results based on our data. BMI = body mass index; FAA = food anticipatory activity; IGF-1 = insulin-like growth factor-1; TCA cycle = tricarboxylic acid cycle; TNF-α = tumor necrosis factor-α. (1) Calorie restriction remains the most robust intervention to extend life span and improve healthspan. (2) The cerebellum is most commonly associated with motor control and cognitive and sensory functions, although it has strong links with the hypothalamus and is more recently thought to play a role in nutritional regulation and adiposity. (3) Cerebellum plays a role in feeding behavior, and through the circadian clock, rhythms have been shown to be centrally involved in the coordination of food anticipatory activity. (4) Cerebellar metabolism is increased by stomach expansion through the vagus nerve. (5) The hypothalamus controls energy balance through, among other signals, adipokines (adiponectin, interleukin-6, and TNF-α, leptin) and insulin, which generally circulate in the blood proportionally to body fat content; however, insulin also spikes after meals. (A) Mice under restriction show high levels of FAA for 2 h prefeeding, and this behavior was inversely correlated with factors that regulate energy balance such as leptin, insulin, TNF-α, and IGF-1. (B) Data in Green et al. suggest an essential role for the cerebellum in physiological and behavioral responses to food intake. The relationship that exists between the cerebellum and nutritional regulation may be in part due to strong direct connections with the hypothalamus, which is a major area of the brain associated with hunger signaling. (C) Pathway enrichment indicated changes in the pathways of adenosine and guanine, which are precursors of DNA production, in addition to changes in pathways of metabolism of in aromatic amino acids, tyrosine, phenylalanine, and tryptophan, and the sulfur-containing amino acid methionine. (D) They also detected increases in the TCA cycle, electron donor, and dopamine and histamine pathways. (E) In particular, changes in l-histidine and homocarnosine correlated positively with the level of caloric restriction and food anticipatory activity and negatively with insulin and body temperature. (F) Several metabolic and pathway changes acted against changes seen in age-associated neurodegenerative disorders, including increases in the TCA cycle and reduced l-proline. (G) Carnitine metabolites contributed to discrimination between CR groups.
Previous work has suggested that oxidative stress in humans is an adaptation in the aging process and is not so harmful (26). Nevertheless, if the damage accumulates throughout the entire life span, as a by-product of normal cellular processes or a consequence of inefficient repair systems, this could lead to age-associated comorbidities. For example, it has been shown that in the brains of patients with Parkinson’s disease, the tricarboxylic acid (TCA) cycle and mitochondria are dysfunctional, which is thought may contribute to the degeneration of neurons (27). In the metabolome of our mice, we saw an increase in changes in amino acid and the TCA cycle pathways, which may indicate the potential provision of nutrients (amino acids, carbohydrates, or fatty acids). It has been previously suggested that life span extension with CR is an evolutionary adaptation to reallocate limited resources during periods of food shortage (1). Although recently, an alternative idea called the “clean cupboards” hypothesis proposed that animals under CR only do things that make a contribution to save energy and maintain energy balance (28), with the aging effects being only an epiphenomenon. In that context, we support the idea that under CR, the body makes metabolomic changes to maintain the energy balance since on the cerebellum in our mice, the pathways containing metabolites with significant associations were mainly related to the production and balance energy. Furthermore, in these mice, we have previously seen that some circulating factors that regulate energy balance, such as leptin, insulin, tumor necrosis factor-α, and insulin-like growth factor-1, were also altered due to CR (Figure 1). Thus, there is a possibility that the positive effects of the CR on neurodegeneration could be a side-effect of maintaining the energy balance.
While we did not specifically measure the impact of this in our study, one theory that has been postulated to explain the impact of CR on life and healthspan is hormesis (in some cases, it is termed as “preconditioning”) (28,29). Hormesis is a dose–response phenomenon characterized by low-dose stimulation and high-dose inhibition (29). There is evidence that it may play a neuroprotective role in the brain by acting on neurons and other cell types in the nervous system. For example, mitochondrial superoxide production is believed to contribute to neuronal damage in a number of conditions ranging from chronic intermittent cerebral hypoxia to Alzheimer’s disease. However, it has been widely reported that transient exposure of neurons to low levels of superoxide converted into hydrogen peroxide can protect neurons against subsequent exposure of what would have been a lethal dose (29,30). Life-span experiments resulting in improved health parameters in the brain have been performed in both rodents and humans, using antioxidant and hormesis mimetics, such as polyphenols (as with resveratrol) and spermidine (a polyamine) (14). However, in our mice (3), there was no indication that CR affected common oxidative stress measures, and the antioxidant activity was actually lowered with CR. Therefore, we maintain the idea that our study at least follows the “clean cupboards hypothesis,” which posits that many of the benefits of CR are conferred inadvertently, as a side-effect of the short-term elimination of structures of no current value to the mouse but which can be digested to provide some energy, such as misfolded proteins and damaged organelles. While in the short term, this process exists to metabolize anything with energetic value to maintain energy balance, there may be a range of unplanned positive and negative long-term outcomes (28).
The metabolomics data in Green et al. (3) indicated that CR altered the metabolome of the cerebellum and did so in a graded fashion, after only a short period (3 months) of restriction. They found that cerebellar levels of l-proline, a neuroexcitatory amino acid, were negatively correlated with the level of CR. l-proline has been shown to destroy neural cells in the hippocampus of rats, resulting in pathologies similar to Huntington’s and Alzheimer’s diseases, suggesting that reducing l-proline may be neuroprotective (31). Moreover, Green et al. (3) have shown that several carnitine metabolites, which are a vital cofactor for the mitochondrial oxidation of fatty acids, were significantly increased with CR. Studies in humans and mice suggest that increased carnitine metabolites have a favorable role in restoring cerebral energy metabolism, which leads to a beneficial effect on major depressive disorders and Alzheimer’s disease, both of which are highly prevalent in the geriatric population (32). Even though there is still a long way to go to establish the precise metabolic mechanisms underpinning CR, previous observations in the cerebellum combined with the study of Green et al. (3) suggest amino acids and the TCA cycle as potentially key pathways in the investigation of the impact of CR on longevity and improved healthspan both in human and mice.
In addition, correlation of metabolomic cerebellar levels with previous food anticipatory behavior and hypothalamic transcriptional changes suggested that changes in the cerebellum metabolites were related to feeding regulation since we noted that previous work has suggested the cerebellum has an active role in the hypothalamo–cerebellar connections driven by hunger responses. In humans, the cerebellum has shown an activation linearly associated with increased body mass index (33). Likewise, cerebellar metabolism is increased by stomach expansion through the vagus nerve (34). In the study by Green et al. (3), it was emphasized that a relationship existing between the cerebellum and nutritional regulation may, in part, be due to direct connections with the hypothalamus. In many mammals, including primates, it has been revealed that widespread areas of the hypothalamus project into the cerebellum and connections are direct and reciprocal (35).
Further evidence has indicated that some of the direct hypothalamo–cerebellar projections involved in feeding and behavioral responses are histaminergic (36). Along with this, it has been reported in both humans and mice that histamine receptor densities decline with aging, and this imbalance may contribute to brain pathologies and dysfunction in older people (29). For instance, histamine levels are reduced by 42% in the hypothalamus of patients with Alzheimer’s disease, indicating decreased brain histamine may be associated with cognitive decline (37,38). Additionally, histidine supplementation has been shown to improve insulin sensitivity and decrease body weight in obese women (39), which is thought to occur through histamine suppression of the appetite and promotion of adipocyte lipolysis (40,41). Green et al. found that cerebellar l-histidine increased in a graded manner with increasing CR, despite being an essential amino acid derived only from the diet, and hence would have been supplied from the diet in the opposite direction. This may reflect the increased need for adipocyte lipolysis to provide energy to make up for the higher calorie deficit (41).
In summary, using mice, Green et al. (3) showed that graded CR modulated multiple metabolomic pathways in the cerebellum (mainly the TCA cycle pathway and some amino acid pathways). Here, we suggest that these changes may be potentially beneficial with regards to neurodegeneration, which has obvious implications for humans as both a long-term lifestyle change and short-term intervention. Furthermore, CR might help to stabilize changes that occur due to aging in the brain as a coincidental side-effect to maintain the energy balance both in humans and mice, that is, the “clean cupboards hypothesis.” Finally, we want to underline the need to search for an appropriate animal model, although it will not be easy, that more closely reflects physiological and molecular mechanisms observed in the human brain. At least for now, it has been shown that graded CR plays a role in modulating the cerebellar metabolome in mice and may act to defy age-associated neurodegeneration through metabolic changes.
Acknowledgments
The authors would like to thank the editor in chief Rozalyn Anderson for the opportunity to prepare this perspective and we acknowledge John Speakman for constructive criticism in reviewing the paper, which helped us to refine our ideas. LAGF was supported by a PIFI post-doctoral fellowship from CAS.
Funding
This work was supported by the UK Biotechnology and Biological Sciences Research Council BBSRC (BB/G009953/1, BB/P009875/1 and BB/J020028/1 to J.R.S.) and a grant from the National Science foundation of China also to J.R.S. A studentship supported C.L.G. from the BBSRC EastBio Doctoral Training Partnership (1438803). C.L.G. received support from the laboratory of D.E.L.P.; D.E.L.P was supported in part by National Institute of Healthgrant AGO49494. C.L.G. is also supported by the 2020 Glenn Foundation for Medical Research Postdoctoral Fellowships in Aging Research.
Conflict of Interest
None declared.
References