-
PDF
- Split View
-
Views
-
Cite
Cite
Nicholas E Scott-Samuel, Tim Caro, Samuel R Matchette, Innes C Cuthill, Dazzle: surface patterns that impede interception, Biological Journal of the Linnean Society, Volume 140, Issue 4, December 2023, Pages 485–503, https://doi.org/10.1093/biolinnean/blad075
- Share Icon Share
Abstract
‘Dazzle coloration’ describes a wide variety of high-contrast patterns allegedly providing protection against attack during motion. Previous research falls into three broad groups. First, studies using humans demonstrate that certain surface patterns can cause significant misperceptions in controlled laboratory conditions, although the effects are inconsistent in both direction and magnitude. Second, experiments on target capture or tracking also show effects that are strongly dependent upon the test paradigm. It has not been established that these laboratory findings generalize to other species, or to the real world. Third, mainly comparative studies build a case for longitudinal striping being involved in escape strategies in some squamate reptiles. We suggest that: (1) the concept of dazzle conflates a description of appearance with presumed function; (2) some effects attributed to dazzle have not been distinguished clearly from other mechanisms of protective coloration; and (3) confusion persists over the evidence necessary to attribute a dazzle function to markings. We refine the definition of dazzle to exclude appearance: dazzle is coloration that interferes with target interception, as a result of misperception of its speed, trajectory and/or range. Our review clarifies discussion of dazzle, and sets out a coherent and practical framework for future research.
INTRODUCTION
‘Dazzle coloration’, a naval term imported into biology, describes a heterogeneous collection of high-contrast patterns that, allegedly, protect moving prey from attack. Dazzle coloration might be favoured in species that live in exposed habitats or need to move rapidly between refuges, where any misdirection of attack might allow the prey to escape a strike. How, exactly, this putative defence might work remains unclear. The origins of the term lie in colour patterns applied to ships during the latter stages of World War I, as a presumed defence against submarine attack. In the section entitled ‘Historical background’, we highlight the positive and negative effects that this has had on subsequent biological thought. Under ‘The current biological understanding’, we categorize and evaluate the studies on dazzle that exist in the literature, going into some depth about the possible mechanisms underlying these effects. Next, we highlight some of the continuing confusion in this area of research, which we believe derives from three specific problems with the use of the term ‘dazzle’ in the context of defensive coloration. First, it refers to both the description of a pattern and the presumed function of that pattern. This conflation between a stimulus and its effect leads to conceptual confusion. Second, use of the word ‘dazzle’ in conjunction with other terms, such as ‘camouflage’, ‘coloration’, ‘markings’ and ‘motion’, is inconsistent and can lead to it being attributed to phenomena better described as disruptive, aposematic (warning coloration), mimetic, deimatic (startling) or neophobic. This risks making the meaning of ‘dazzle’ so broad as to be empty, and one goal of our review is to provide clear bounds to what can be described as ‘dazzle’. Third, the evidence needed to attribute a dazzle function, rather than some other function, to a colour pattern has not always been characterized clearly. In the final section, we propose a modified definition of dazzle and suggest fruitful directions for future research.
We take as our starting point the terminology of Stevens et al. (2008): ‘In nature, “motion dazzle” markings are essentially high-contrast anti-predator patterns, which may prevent predators from accurately judging the speed and trajectory of a moving prey item’ (p. 2639). Although earlier writers, such as Thayer (1909) and Cott (1940), had discussed how high-contrast patterns might serve to deceive in both nature and war, it was Stevens (2007) and, in the first experimental studies, Stevens et al. (2008) and Scott-Samuel et al. (2011) who drew a putative direct link between the mechanisms involved in some anti-predator colour patterns seen in nature and anti-attack coloration that had been invented and applied to ships in World War I. For this reason, it is important to summarize briefly how naval dazzle was thought to function, and the evidence for its effectiveness.
HISTORICAL BACKGROUND
Dazzle coloration has its roots in attempts to reduce the success of submarine attacks on merchant ships in World War I. The concept of ‘dazzle painting’ ships is linked inextricably to a British artist, Norman Wilkinson (1878–1971), who, in 1917, persuaded the British Admiralty to drop attempts at concealment and concentrate on confusion, switching from homogeneous grey to the high contrasts and geometric patterns that we associate with naval dazzle designs (Fig. 1A). Wilkinson’s goals were quite specific - the protection of merchant shipping from torpedo attack by German submarines (U-boats) - and his logic clear: you cannot conceal a ship on the open seas because its silhouette against the sky, and smoke from its funnels, will reveal it and, in any case, the engine noise will be detected by the U-boat's hydrophones. Instead, the goal was to confuse the U-boat captain as to the target's identity (both in terms of the type of ship and also whether it was a ship or another object) and its velocity (speed and/or direction). This goal was simplified by the very restrictive circumstances in which the captain had to make his judgement: through a periscope. This meant that the viewing aperture was small, and only a relatively brief observation could be made (because the periscope produced a salient wake if raised for too long).
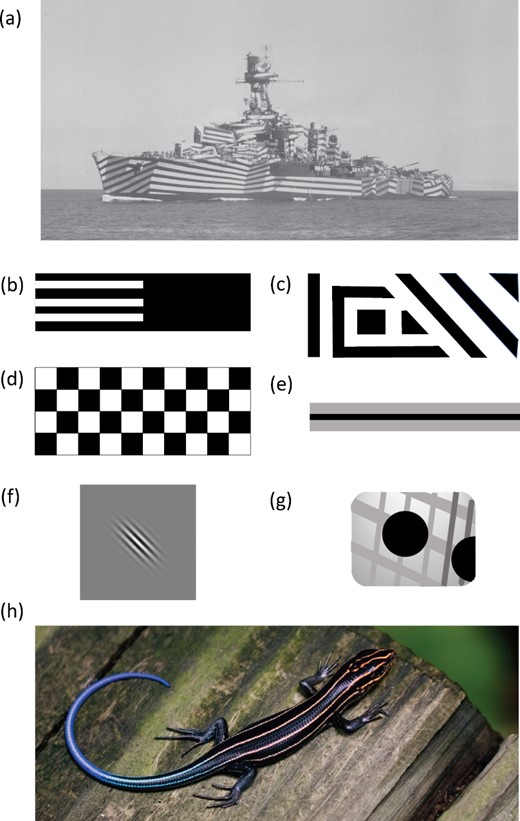
A, French cruiser Gloire (public domain photograph from the US National Archives 80-G-K-1208, National Archives Identifier 178141086). B–G, selected examples of stimuli used in computer-based experiments to show their great variability across studies, redrawn not to scale, from: B, Murali & Kodandaramaiah (2016); C, Stevens et al. (2008), Scott-Samuel et al. (2011); D, Scott-Samuel et al. (2011),; E, von Helversen et al. (2013),; F, Hughes et al. (2017),; G, (Hughes et al., 2021). H, Japanese skink (Plestiodon japonicus), one of the species in the study of stripes in lizards by Murali & Kodandaramaiah (2016). Photograph by Kris Kelley, sourced from Wikimedia Commons under CC BY-SA 3.0 licence and cropped by the authors.
In Wilkinson’s subsequent accounts (1919, 1922, 1969) he emphasized the use of colour patterns to interfere with a U-boat captain’s judgement of trajectory. And it is this specific claim that has influenced contemporary biological research on dazzle coloration: whether such coloration leads to errors in a predator’s estimation of speed or direction. Certainly, photographs of some dazzle-painted ships are impressive: it is sometimes hard to determine the ship’s bearing (the French cruiser Gloire is a striking example; see Fig. 1A). But did it work with moving targets and did it work in wartime? This has not been resolved. There was certainly contemporary research in naval research facilities, using model ships, that tested explicitly how different colour schemes affected biases in perception (Skerrett, 1919; Warner, 1919). However, post-war assessments of shipping losses with respect to coloration are inconclusive (Blodgett, 1919) because dazzle camouflage was introduced at the same time as the use of convoys, as well as during a period of constant changes in U-boat and allied shipping tactics in the remaining months of the war. The only firm conclusion was that it generated a large psychological boost for both merchant navy personnel and civilians (Behrens, 2002, 2009; Forbes, 2009; Taylor, 2016).
Although the naval use of dazzle influenced the first experiments by biologists (Stevens et al., 2008; Scott-Samuel et al., 2011), extrapolation to biological systems should be made with caution. First, naval dazzle was used in environments with no background reference points (at sea). Second, the medium between observer and target was often obscured by mist, waves and poor lighting conditions. Third, time to fix the target visually was limited. Fourth, the distance between observer and target was large. Fifth, a projectile was launched against a target. In many predator–prey interactions, these factors do not apply or only some apply. Nonetheless, this historical perspective frames the conditions in which dazzle coloration might or might not evolve.
THE CURRENT BIOLOGICAL UNDERSTANDING
We conducted a systematic review of the literature up to November 2022, following the PRISMA guideines (www.prisma-statement.org), with 43 articles meeting our inclusion criteria (see the Supporting Information). We discuss the literature in three natural groupings: studies investigating the perception of targets (N = 13); experimental studies investigating the capture or tracking of targets (N = 20); and comparative studies (N = 16). Note that the total is not 43 because some papers contained more than one type of study. The first of these groupings can establish whether the claimed underlying perceptual effects exist; the second can demonstrate whether those perceptual effects translate into reduced capture success, and hence are a proxy for increased fitness; and the third can reveal the ecological context within which dazzle patterns might be favoured. Establishing that perception distortions translate into capture success is important because, as Kodandaramaiah et al. (2020) discuss, perceptual biases might be measurable when all else is held constant, but in nature the effects might be small in comparison to other attributes of a target. Initially, however, we provide an overview of currently known psychophysical effects that might be relevant to dazzle.
Potential Underlying Mechanisms
The list of factors that might influence the perception of motion is long (for reviews, see Burr & Thompson, 2011; Nishida, 2011). In the context of dazzle, we divide the effects into three broad categories: directional ambiguity; distortion of perceived speed; and strength of motion signal. While speed and direction are independent (combining to give velocity), motion signal strength could plausibly interact with the other categories.
One cause of directional ambiguity is the aperture problem (Adelson & Movshon, 1982): the direction of motion of a moving boundary seen through an aperture can be misperceived (Fig. 2A) . Another well-studied phenomenon is the wagon wheel illusion, in which undersampling of the moving signal causes aliasing, and therefore perceived reversed motion (Fig. 2B). Some debate exists as to whether this effect can occur under natural lighting and viewing conditions (it is most often observed in movies), but the balance of evidence indicates that it probably does (Andrews & Purves, 2005); for example, it could arise as a result of eye or head movements when observing a moving stimulus. Both these potential causes of ambiguity in extracting an accurate direction signal could have obvious application to any moving striped pattern. Finally, for any richly textured non-rigid surface, there is the issue that various parts of that surface might be moving in different directions at a given instant (think of the limbs of a running quadruped; How & Zanker, 2014). The main information that a predator needs to extract for physical interception of a target is the global motion, i.e. that of the whole animal, not the various local motions of body parts. A salient surface pattern can therefore add noise to the veridical directional signal.
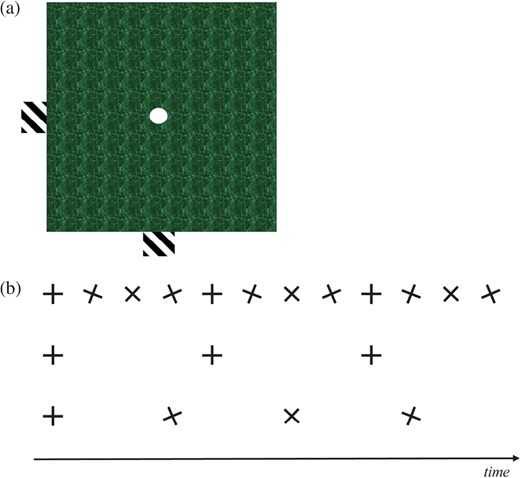
A, the aperture problem. See Video 1 (the video is playable in the HTML version). Initially, a horizontal bar with oblique stripes moves rightwards; this direction of motion is clear when seen on either side of the occluding green square, but when its stripes are viewed through the central circular aperture they appear to drift towards the top right, orthogonal to their orientation. Next, a vertical bar with similarly oriented stripes moves upwards; again, the motion is perceived correctly below and above the green square, but through the central aperture its stripes also appear to drift towards the top right. Motion viewed through an aperture may not be perceived veridically. B, the wagon wheel illusion. If a moving stimulus with regular structure is temporally sampled, the rate at which it is sampled can affect how it is perceived. Here, a clockwise rotating cross is sampled 12 times (top row, black crosses) as it rotates through 360°: the perception of motion is veridical. However, if it is sampled only every fourth frame in the same period (middle row, black crosses), it will appear not to move. And if it is sampled every third frame (bottom row, black crosses), it will appear to move anti-clockwise. A similar principle applies to repeating spatial patterns, such as stripes: when they are orthogonal to the direction of motion, the stripes could appear to move backwards; when they are parallel to the direction of motion, there is no speed information at all, and the direction is ambiguous (forwards, backwards or even stationary).
The best-known factor that distorts perceived speed is stimulus contrast (Thompson, 1982). However, the effect is variable and appears to depend on both temporal frequency (Thompson, 1982) and speed (Thompson et al., 2006). Orientation of surface pattern affects speed perception, in that horizontal stripes (relative to the direction of movement) are seen as moving more quickly than vertically aligned ones (Georges et al., 2002). Target size influences perceived speed, with smaller objects seen as moving more quickly than larger ones (Brown, 1931).
Finally, the strength of any motion signal can be influenced by a number of factors. In the broadest terms, this can be thought of in terms of signal-to-noise ratio (Merilaita et al., 2017): the signal is the veridical motion, and any non-relevant input to the visual system is the noise, which can potentially degrade the signal. Parameters such as poor sampling (a low rate at which information is updated in the visual system compared with the motion of interest; Purves et al., 1996) or fluctuation in stimulus strength (Georgeson & Scott-Samuel, 1999) lead to an increase in noise. Although a low signal-to-noise ratio does not, of itself, represent a bias in the perception of motion, the increased uncertainty could, nevertheless, affect the decision to attack or the path of interception.
Studies Investigating Effects on Perceived Speed, Trajectory and Range
Speed estimation
Only 4 of the 11 published studies yielded measures of effect size (Scott-Samuel et al., 2011; von Helversen et al., 2013; Murali & Kodandaramaiah, 2016; Kodandaramaiah et al., 2020). All involved computer-based tasks with simple geometric shapes and human participants (for sample stimuli, see Fig. 1B, D and E) . Three found that on fast-moving targets (> 15° of visual angle per second), stripes parallel to the direction of motion caused an underestimation of speed (Fig. 3A). In the studies by Murali & Kodandaramaiah (2016) and Kodandaramaiah et al. (2020), the effect was large at both high and low contrast, although diminished in the latter. In the study by Scott-Samuel et al. (2011), the effect size was consistent with those observed in the two aforementioned studies, but not significantly different from zero, and noticeably reduced for low-contrast and for slow-moving targets (3.33°/s). In contrast, von Helversen et al. (2013) found that parallel stripes caused significant overestimation of speed. Scott-Samuel et al. (2011) found significant underestimations of speed only for high-contrast, fast-moving zig-zags and checkered patterns. The other studies (Murali & Kodandaramaiah, 2016; Kodandaramaiah et al., 2020) did not test patterns more complex than simple stripes. For targets with stripes orthogonal to the direction of motion, there was a disparity in the measured effects: in Scott-Samuel et al. (2011), the underestimation of speed was not significant and similar to those for parallel stripes, whereas in Kodandaramaiah et al. (2020) and von Helversen et al. (2013) orthogonal stripes produced large overestimations of speed.

Effect sizes (Cohen’s d ± 95% confidence interval) for different pairwise contrasts from experiments on the effect of surface patterns on: A, perception of speed; B, perception of speed (but the patterns are dynamic); C, perception of trajectory; and D, capture success. The papers from which the effect size estimates come are indicated on the panels themselves, with different coloured backgrounds for each paper. Effects have been calculated such that a positive value indicates that: for A, the perceived speed of a dazzle-patterned target is higher than that of a non-dazzle control (usually unpatterned, but sometimes unoriented blobs are the control for striped patterns); for B, patterns moving in the direction of target movement increase perceived speed and patterns moving in the opposite direction reduce perceived speed; for C, the perceived trajectory is biased towards the direction in which oblique stripes point; and for D, patterns reduce capture success compared with a control (again, usually unpatterned, but sometimes unoriented blobs or background-matching camouflage). Full descriptions of the stimuli, presentation details and interpretation of effects are given in the Supporting Information (Supplementary Data File S1).
Hall et al. (2016) published a comprehensive set of experiments investigating the effects of dynamic patterns on speed estimation (Fig. 3B). Their stimuli were moving objects covered with a pattern that also moved: orthogonal stripes moving in the same direction as the direction of motion increased the perceived speed of a target, and those moving in the opposite direction reduced perceived speed. The same effects were seen with low-contrast stripes (cf, Scott-Samuel et al., 2011, with static patterns), with colours (brown and green) more typical of camouflage than conventional dazzle, and also when participants had heightened anxiety induced by inhalation of air enriched with CO2. Perceptual biases disappeared only when the moving patterns were present on just the leading edge of a target. However, while the speed perception biases measured in Hall et al.'s (2016) seven experiments appear strong and consistent, they all come from one experimental paradigm: they lack independent replication. It is clear that the other existing experiments on speed estimation have yielded very different, and sometimes contradictory, results. We return later to the possible reasons for this and other inconsistencies. However, on the available evidence, we can safely conclude that one cannot predict a priori whether a striped animal might be perceived as moving faster or slower than its true speed, or whether there will be any bias at all; the effect seems to vary with context.
Trajectory estimation
Only one study has looked at the effect of dazzle-type patterns on estimates of the direction of travel (Fig. 3C). Hughes et al. (2017) asked human observers to predict where a horizontally moving target would cross a vertical line. The target was a Gabor patch (a sinusoidal modulation of luminance convolved with a Gaussian envelope; see Fig. 1F), which had the appearance of a fuzzy-edged disc containing blurred stripes; these stripes lacked the sharp boundaries of those used in most other experiments, but they had the virtue of being composed of a single spatial frequency. Hughes et al. (2017) used a sophisticated factorial design, balancing for direction of motion and absolute stripe orientation; we reanalysed their data in terms of stripe orientation relative to the direction of motion, in order to simplify the presentation and interpretation of effect sizes. Hughes et al. (2017) found that targets with oblique stripes pointing upwards, relative to the direction of motion, were judged to pass a point above the estimated point for targets with downward-pointing stripes, when targets were moving at medium or high speeds (10 or 13.33° of visual angle per second). However, the effect was reversed at low speeds (6.67°/s). Hughes et al.'s (2017) interpretation was that, consistent with previous research, targets moving faster than the ability of the visual system to isolate spatial position leave a ‘motion streak’ (blur) that is used as a direction cue. However, the reversal (rather than disappearance) of the trajectory estimation bias was unexpected. That the effect of oriented patterns on trajectory estimation can differ markedly with target speed certainly deserves further investigation. A second manipulation investigated moving stripes: when the stripes moved within a moving Gabor patch, upwards stripe motion shifted the predicted location of the target higher relative to downwards motion. Unexpectedly, the effects were stronger for static patterns than for these dynamic patterns (cf. the strong effects of moving patterns on speed estimation found by Hall et al., 2016).
Range estimation
Although most attention has focused on the potential of dazzle to interfere with speed and trajectory estimation, successful interception of a target also requires judgement of distance, and some naval painting schemes were designed to interfere with range estimation (Forbes, 2009; Taylor, 2016). Repetitive patterns were argued to make it harder to align the two views provided by early optical, so-called ‘coincidence’, rangefinders.
While this might seem to have no relevance to biology, certain types of surface patterning can interfere with depth judgment through stereopsis (Nityananda & Read, 2017). Even for animals that judge depth through monocular cues (the majority, given that stereopsis requires forward-facing eyes with overlapping fields of view), distorting the apparent size of an object will influence judgements of its range: smaller objects, ceteris paribus, are further away (https://www.youtube.com/watch?v=vh5kZ4uIUC0). Smaller objects are also seen as faster moving (Brown, 1931), so any size distortion caused by coloration might not only interfere with range estimation, but could also indirectly influence perceived speed. However, we found no study that directly investigates an effect of surface patterning on perceived distance.
A classic illusion is that an object covered with horizontal stripes appears narrower and taller than an identically sized square composed of vertical stripes (Helmholtz, 1867). This effect has been verified for humans under controlled laboratory conditions for both two-dimensional (static female silhouettes) and three-dimensional (photographs of female mannequin torsos) horizontally and vertically striped stimuli (Thompson & Mikellidou, 2011). In a more biologically relevant experiment, Karpestam et al. (2018) demonstrated that photographs of pygmy grasshoppers (Tetrix subulata) with longitudinal stripes were judged, by human observers, to be smaller than plain grey grasshoppers. A consequence of this would be that, all other things being equal, striped prey might be judged to be further away than they really are; this has not been tested. Cott’s (1940: 93–96) suggestion that stripes that interrupt or are close to the margin of an object, irrespective of their angle, make it look larger than an object where the pattern conforms to the border has been examined once. A single observer estimated heights and girths of individuals of three free-living ungulate species on an ordinal scale, and compared them with calculated measures of size derived from photographs with known camera–object distances. Contrary to Cott’s (1940) proposal, performance on this task did not vary between striped live plains zebras (Equus burchellii) and uniformly coloured waterbuck (Kobus ellipsiprymnus) or topi (Damaliscus korrigum) (Caro, 2016).
Studies Investigating Effects on Capture (Or Tracking)
The most common type of experiment investigating dazzle coloration uses capture success or the ability to track a target. In the majority of papers from which effect size estimates can be extracted (12 of 15), this was a human clicking on a moving shape on a computer screen. Two of the non-human studies involved birds pecking at artificial targets on a computer screen (Pike, 2015) or mechanically drawn across an aviary floor (Hämäläinen et al., 2015); one involved toads attacking live (but colour-manipulated) crickets (Zlotnik et al., 2018). While the latter would seem to have the greatest ecological validity, it was not stated how or if the prey were moving at the time they were attacked, so the reported null effects of stripes are hard to interpret. The other experiments had the virtue of stimulus motion and other characteristics being tightly specified and, in the human experiments, participant viewing distance and attention also constrained.
Nonetheless, the clearest message from inspection of the effect sizes is that there is no clear pattern (Fig. 3D). All have been plotted such that a positive effect measure represents a benefit of dazzle patterns (in these studies, all various types of black and white stripes) compared with a control treatment (usually plain grey, but sometimes irregular blotches of the same colour as the stripes). However, while many show that stripes reduce capture success, many also show the opposite, sometimes within the same paper (e.g. in Stevens et al. (2011), fewer striped targets were captured than those with background-matching camouflage, but plain grey targets were significantly harder to catch than those with stripes). It is impossible to isolate the source of the variation in effect size because the differences between experiments are either too variable (e.g. across the 11 studies of capture success in Fig. 3D, stripes are compared with nine different ‘control’ treatments) or are confounded with any of the other, reported or unreported, differences between studies. For example, effect sizes appear higher in studies where the background against which targets were seen was a mean luminance grey, compared with textured backgrounds (general linear mixed model; source paper as a random effect, fixed effect of background = 1.13, likelihood ratio test = 5.46, d.f. = 1, P = 0.019). However, the effect estimates for studies with mean luminance backgrounds come from only three papers (Pike, 2015; Murali & Kodandaramaiah, 2016, 2018), and in the single study where both plain and textured backgrounds were used (Hämäläinen et al., 2015), no interaction with target coloration was detected. However, we provide the characteristics of the different studies in the Supporting Information (Supplementary Data) so that, in the future, when more studies have accumulated, more sensitive meta-analyses can be carried out.
While capture success approximates a fitness measure for the effect of the colour pattern, it comes with the important caveat that the effect might not be attributable to a misjudgement of speed or trajectory. Furthermore, the more natural the movement pattern, the harder it is to identify which aspect of interception might be suffering interference, because changes in speed, direction and predictability might covary. Hence, the results of experiments in this section do not provide direct evidence of dazzle as the causal factor, as they could be either consistent or inconsistent with a predicted effect of dazzle coloration. This is an example of the logical error of affirming the consequent (A implies B; therefore, if B then A); here, if dazzle implies that capture success is reduced, reduced capture success does not logically imply dazzle. This is also an issue for the comparative studies in the next subsection.
Within this group, four studies are somewhat distinct: three are united by their investigation of the effect of patterning on the ability to track one target among many (Hogan et al., 2016a, b, 2017a), and the fourth (Hughes et al., 2015) also uses multiple moving targets, but a different task (catch all targets). For the first three, consistent with previous research on the confusion effect (Scott-Samuel et al., 2015), target tracking became less accurate as the density of moving objects increased; the novel finding was that stripes parallel to the direction of motion enhanced this effect (Hogan et al., 2016a, b, 2017a). This effect was not, however, found for the multiple capture task (Hughes et al., 2015). While the first three studies show an interaction between dazzle and the confusion effect, this is ‘dazzle’ used as a descriptor of the pattern, rather than its effect. The effects in these three papers could indeed be a result of interference with perception of target speed, trajectory or even range, but could equally well be to do with the difficulty of individuating the targets because of their patterning. Because the latter does not interfere with perceived motion, we would not regard it as a dazzle effect under Stevens et al.'s (2008) definition.
Comparative Studies
Based on our understanding of predator–prey relationships in a variety of taxa and the ecological contexts in which dazzle might be advantageous, we predict that dazzle is more likely to be used by: (1) open-country or open-water species, because opportunities to escape into rocky crevices or into vegetation are unavailable; (2) short species, where a misdirected attack would miss the body altogether, as opposed to longer species where a poorly aimed attack might still hit a moving body part; and (3) rapidly moving species, because dazzle effects are seen (when they are detected at all) in fast-moving targets (see ‘Speed estimation’ subsection above).
Studies of squamates have addressed some of these points. They indicate that both transverse and longitudinal striping (with respect to the direction of motion) make it difficult to judge the velocity of a target, or even whether it is moving (Jackson et al., 1976; Murali & Kodandaramaiah, 2016), potentially causing attacks to be misdirected to less vital parts of the body, or for the prey to be missed altogether (Allen et al., 2013; Murali & Kodandaramaiah, 2018). Counter to our prediction, early studies suggested that transverse banding patterns were more common in slow-moving snakes (Jackson et al., 1976; Pough, 1976); however, this association disappeared in a subsequent phylogenetically controlled comparative analysis of Australian and American snakes (Allen et al., 2013).
A greater amount of comparative work has been carried out on species with longitudinal striping. Comparative phylogenetically controlled analyses indicate that this striping pattern is found principally in diurnal lizards, ground-dwelling non-arboreal lacertine and agamid lizard species (Chen et al., 2013; Murali et al., 2018), in fast moving species (Halperin et al., 2017) and in lacertine lizards that live at higher temperatures, that have colourful tails and exhibit caudal autotomy (Murali et al., 2018). In snakes, longitudinal striping is associated with living in exposed environments (Jackson et al., 1976) and in North America and Australia with species that are small and fast (Allen et al., 2013). To generalize across these taxonomic groups, longitudinal stripes are associated with species that tend to be short and slender for their clade (Allen et al., 2013; Murali & Kodandaramaiah, 2018), an intuitively satisfying finding given that predators might completely miss a short animal if their attack was slightly misdirected, but not necessarily a longer one. Interestingly, in geckos there is no significant association between longitudinal striping and diurnal activity, perhaps because they are slow moving (Allen et al., 2020). An important finding from this body of work is that longitudinal striping is associated with caudal autotomy in lizards, which is suggestive of striping leading to attacks being misdirected to the tail (Murali & Kodandaramaiah, 2016). In sum, these comparative studies build a case for longitudinal stripes evolving in rapidly moving, short, ground-dwelling reptiles. Studies in other taxa that could be interpreted as dazzle are inconclusive (e.g. Seehausen et al., 1999; Carretero et al., 2006; Rojas et al., 2014; Taylor et al., 2019; Pizzigalli et al., 2020) or focus on tails rather than bodies (e.g. Ortolani, 1999; Hawlena et al., 2006) or are only purportedly focusing on dazzle (e.g. Marshall & Gluckman, 2015).
Relevant to possible interactions between dazzle and the confusion effect (Hogan et al., 2016a, b, 2017a), research that links grouping with conspicuous markings shows that in Anseriformes, Charadriiformes, Artiodactyla and several orders of marine fishes, contrasting patterns are more prevalent in social species (Negro et al., 2020). One explanation might be confusion of predators in group-living prey species operating through dazzle, but other explanations, such as informing conspecifics of group movements, are also possible (Brooke, 1998). Moreover, the conspicuous markings recorded in the Negro et al. (2020) study are remarkably different both within and between taxa, consisting of vertical and horizontal markings, white patches of feathers on different areas of the body, multiple longitudinal body stripes and single blazes of achromatic pelage, questioning the degree to which comparative studies are the correct tool with which to explore this issue.
Some General Comments
In passing, it should be noted that some experimental environments might not deliver exactly what is required. For example, without display calibration, any reported luminance values are likely to be inaccurate; high-level programming languages (e.g. Scratch; https://scratch.mit.edu) do not offer the degree of stimulus control afforded by lower level ones (e.g. PsychoPy: Peirce et al., 2019; or Psychtoolbox: Kleiner et al., 2007), meaning that specified values for parameters such as speed might not be reproduced accurately; and using stimuli with sharp luminance boundaries can constrain the granularity of speeds that can be displayed. For the simple purposes of demonstrating a non-zero effect, these fine details are unlikely to matter because they just add experimental noise. However, for determination of the magnitudes of effects and thresholds, they do matter. Perhaps an even more important caveat is that comparability across datasets is compromised when stimulus construction and display vary between studies (see Fig. 1B–G). Furthermore, not only are there stimulus differences but also response task differences, making mean effects across studies harder to interpret. This variability across studies, and subsequent difficulty in synthesizing previous work, also holds for the comparative studies discussed in the previous section.
The variability in the effects reported in the four speed estimation studies (see subsection ‘Speed estimation’ above) is probably explained by stimulus specificity: the response varies according to the stimulus. The precise visual configuration of the targets varied from study to study, in terms of both the contrast and the form of their surface patterns; so, too, did the backgrounds against which those targets were displayed. The target speeds, in contrast, were broadly comparable across all four studies, ranging from ~11 to 20°/s (except for the slow, 3.3°/s condition in Scott-Samuel et al., 2011). In the context of a signal-to-noise framework (Merilaita et al., 2017), the relationship between the target (signal) and the background (noise) matters; therefore, if these both vary across different experiments, it is challenging to make meaningful comparisons. For example, the backgrounds used by both von Helversen et al. (2013) and Kodandaramaiah et al. (2020) were considerably more complex than those used by Scott-Samuel et al. (2011) and Murali & Kodandaramaiah (2016), which could have implications for the signal-to-noise ratio in these studies, hence the different effect of dazzle found in each pair of papers (see Fig. 3A). It is also worth noting that these experiments compared one stimulus with another, so all the data were relative; it is impossible to determine which pattern, if any, had its speed reported veridically.
The effects obtained in capture/tracking studies (see the relevant section above) are highly variable in both magnitude and direction (Fig. 3D) but, as with the speed estimation experiments, the appearance of the targets and their backgrounds, as well as the target speeds, differ so widely that it is nearly impossible to determine the causes of that heterogeneity. The problem of multiple effects of pattern beyond dazzle also applies to an innovative study involving experimental evolution of moving targets on computer screens (Hughes et al., 2021). Here, the complex surface patterns were controlled by genetic algorithms, and inter-generational selection was determined by capture success by human ‘predators’. Although there was clear evidence of evolution towards low-contrast patterns rather than the high contrasts typical of dazzle, the protocol does not allow us to separate the effects of patterns on salience and detectability (when targets appear, and low-contrast, less visible patterns would be advantageous) from those on interception (when targets move, and the effect of contrast is unclear).
As an aside, zebra stripes are a frequently quoted example of dazzle having a purported confusing effect in fleeing or even stationary animals (e.g. Stevens, 2013). That a group of zebras is termed a ‘dazzle’ adds to this misconception. These claims, made in both the scientific literature and the media, are mainly based on the repeated black and white striped coat pattern, not on their effect on predators. Yet zebra stripes are unlikely to facilitate escape from large predators, based on the latter’s poor visual acuity making stripes unresolvable except at close range (Melin et al., 2016), and two aspects of these predator–prey interactions: flight behaviour shown by zebra groups that fails to utilize any possible confusion caused by stripes, and the high success rate of lions in capturing zebras (Caro, 2016).
In contrast, over the past decade research has shown that Diptera are averse to landing on stripes. In brief, stripes reduce landings of tabanid horseflies based on experimental studies with striped artificial targets (Waage, 1981; Brady & Shereni, 1988; Gibson, 1992; Sasaki et al., 2020), horse models (Egri et al., 2012), human models (Horvath et al., 2019), painted cows (Kojima et al., 2019) and comparisons of live plains zebras (Equus burchelli) with domestic horses (Caro et al., 2019; How et al., 2020), and there is a co-occurrence of tabanid annoyance and striping in wild equids (Caro et al., 2014). Of two candidate explanations for stripes thwarting controlled landings on zebras, the barber pole and wagon wheel illusion (How & Zanker, 2014), experimental work shows that neither is likely to be operating (How et al., 2020; Caro et al., 2023). Currently, then, there is no evidence that zebra stripes function as dazzle markings in the sense used by Stevens et al. (2008) of preventing predator attack or ectoparasite attack by generating a misperception of speed or trajectory.
PROBLEMS WITH CURRENT USE OF THE TERM ‘DAZZLE’
We argue that there are three problems with the term ‘dazzle’ as it is currently used.
Dazzle as a Pattern And Dazzle as an Effect Of That Pattern
‘Dazzle coloration’ and ‘dazzle markings’ are often merely a description of the pattern and shape of colour elements that are highly contrasting on an object (e.g. Stevens et al., 2008; Murali & Kodandaramaiah, 2018). There are two problems with using ‘dazzle’ to describe a pattern in this way. First, the word itself implies an effect, immediately confounding pattern and consequence. Second, and more importantly, it is impossible to identify a pattern as dazzle based on appearance alone. While the single most diagnostic characteristic of dazzle patterns is high contrast, one can obtain apparent dazzle effects with low-contrast patterns (assuming that they are still visible). For example, moving stripes cause errors in speed estimation even when the contrast is low and when colours more typical of camouflage, such as brown and green, are used (Hall et al., 2016). Furthermore, even if high contrast is common in dazzle patterns, there are plenty of other forms of coloration that possess this feature: for example, any animal that is camouflaged against backgrounds with high-contrast elements will have high-contrast elements itself (e.g. a cuttlefish in ‘disruptive’ mode; Barbosa et al., 2008), which reduce attack by lowering the probability of being detected or recognized. High-contrast elements can be also be found on individuals exhibiting aposematic patterns, Batesian mimicry, distraction marks, deimatic displays, startle coloration, flash behaviour, pursuit-deterrence signals and, indeed, many sexually selected signals. In short, it is extremely difficult to define dazzle unambiguously in the sense of an effect-neutral pattern.
Turning to the effect of the pattern, the verb ‘to dazzle’ describes the effect that a certain pattern has on an observer. Its colloquial use covers both a purely perceptual effect (e.g. blinding by a bright light) and an emotional one (e.g. spectacular, impressive). Neither applies to the effects attributed to World War I ship painting or to animal colour patterns. Returning to the Stevens et al. (2008) definition of motion dazzle as ‘essentially high-contrast anti-predator patterns, which may prevent predators from accurately judging the speed and trajectory of a moving prey item’ (p. 2639), we propose that including ‘high contrast’ in the definition is unnecessary and reintroduces the confusion between appearance and effect. This aligns with Stevens & Merilaita's (2009) reasoning with regard to disruptive coloration: its definition should be based on its effect, not its appearance.
‘Dazzle Camouflage’
The juxtaposition of ‘dazzle’ with ‘camouflage’ is unhelpful. ‘Dazzle camouflage’ (e.g. see Scott-Samuel et al., 2011; von Helversen et al., 2013), by virtue of the second word, carries with it the baggage of crypsis: avoiding detection or recognition (Cuthill, 2019). However, the term dazzle was coined specifically to differentiate it from the many ‘low-visibility’ schemes in use at the time (Williams, 2001; Taylor, 2016: p. 29). Thus, ‘dazzle camouflage’ is an oxymoron, and we suggest that it be abandoned.
Dazzle as an Incorrect Label
Disruptive coloration and dazzle coloration share common features, notably high contrast. However, the former breaks up the outline or other distinguishing features of the target (Cott, 1940; Stevens & Merilaita, 2009) and is most effective when at least one pattern element blends in with the background and likely when the prey is stationary (Stevens & Cuthill, 2006). Conversely, for dazzle, no pattern element needs to match the background. Nonetheless, the same patterns that have a dazzle effect could also have a disruptive function when the target is stationary. For example, the paint scheme of the French cruiser Gloire (see Fig. 1A) both conceals its orientation and likely trajectory (a dazzle effect) and makes its shape and identity difficult to discern (a coincident disruptive coloration effect; Cott, 1940; Cuthill & Székely, 2009).
Deimatic displays are transient signals designed to startle or induce fear in a predator, causing it to hesitate in its attack (Umbers & Mappes, 2016; Drinkwater et al., 2022). As with disruptive coloration, it is conceivable that a dazzle pattern might also operate as a deimatic display in a different context, but we would not class deimatic displays as dazzle because the function is to cause hesitation or withdrawal, not interfere with interception.
Flash behaviour (sometimes called flash coloration) describes a normally cryptic animal exposing a conspicuous colour patch either continuously or intermittently during flight, then hiding it when it comes to a halt. This causes the observer to search subsequently for an inappropriate conspicuous object (Edmunds, 1974; Murali, 2018; Loeffler-Henry et al., 2018; Bae et al., 2019; Caro et al., 2020). This is close to dazzle in the colloquial sense (sudden appearance and disappearance of a bright stimulus in a moving object exploits a lag in sensory adaptation) and, as Murali (2018) discusses, this is probably related to the well-known flash-lag effect (e.g. Nijhawan, 1994). But flash coloration interferes with subsequent detection of the stationary object, whereas dazzle interferes with interception of an object that is already detected (and usually moving). Nevertheless, if, in flash behaviour, the conspicuous colour patch is shown intermittently and repeatedly (e.g. as seen in the conspicuous tails of some fleeing leporid species), then both flash behaviour and dazzle coloration could be operating. The former mechanism might prevent the predator from locating the prey after it had become stationary and hidden its tail, while the latter might cause the predator to fail to intercept the fleeing animal, but they are distinct processes.
Flicker fusion is the inability to resolve flashes (or moving texture), thereby obscuring an internal pattern on the target when it is moving (Umeton et al., 2017). It arises from a combination of the acuity limits of the observer's visual system and the spatial and temporal properties of what is being observed. It cannot completely conceal the outline of a moving animal, but the resulting homogeneous texture could reduce its salience (Smart et al., 2020). Indeed, it may best operate in different circumstances from dazzle: transverse striping in two species of coral snakes appears to generate flicker fusion in poor lighting conditions (Titcomb et al., 2014), whereas dazzle should operate under higher levels of illumination. Dazzle requires the pattern to be perceptible to work (more likely under bright illumination), whereas flicker fusion is the failure to resolve a pattern (more likely under dim illumination), so these are very different mechanisms. However, as with the other types of defensive coloration discussed in this section, it is theoretically possible that a pattern that enables flicker fusion at higher speeds could produce a dazzle effect when moving more slowly. Note that it is the relative speed of observer and target that matters for both flicker fusion and dazzle: motion of both the observer and the target must be factored in. Flicker fusion and dazzle are regularly conflated in the literature.
FUTURE DIRECTIONS
Based on the arguments we have outlined above, we propose a modification of the Stevens et al. (2008) definiton of dazzle:
Dazzle is coloration that interferes with the interception of a moving object, due to perceptual distortions of target speed, trajectory and/or range.
Currently, we know that certain patterns can cause perceptual biases in humans performing simple computer-based tasks, but these are not consistent in direction or magnitude and we do not know whether: (1) other animals exhibit the same perceptual errors; (2) humans routinely make similar errors in real-world situations (viz U-boats); (3) non-humans make such errors in the wild; and (4) the patterns seen on living animals have the same effects as the simple geometric designs (mainly black and white stripes) tested in the laboratory. While we know that specific patterns are associated with certain ecological situations in some wild animals, and that these associations could be predicted by dazzle, the same associations might also be predicted by other defensive functions of those patterns. Furthermore, if patterns giving rise to perceptual biases are common (either in an experiment or in the wild), then predators could adapt their behaviour to accommodate any systematic errors caused by those patterns. The corollary of this is that the effects of dazzle might be frequency dependent, a factor that has never been investigated. Finally, some patterns might induce illusory effects in observers, but unless those effects influence target interception they are irrelevant in the context of our new definition of dazzle.
To answer such questions, an integrated experimental approach is required: tightly controlled laboratory studies with artificial prey (with both humans and other species acting as predators) can constrain design choices for more ecologically valid manipulations in the field. In turn, the latter can inform which particular stimulus configurations would be of most interest for further investigation in the laboratory. Additionally, under our new assessment of dazzle coloration, comparative studies are limited in scope because they cannot unequivocally ascribe a mechanism (e.g. misperception of speed, trajectory or range) by which a colour pattern might induce a dazzle effect. With this in mind, we offer some specific and general suggestions for future research in this area.
Stripes
Stripes, either parallel or orthogonal to the direction of motion, have very varied effects on both speed perception and capture success. Some of these effects are compelling, but their direction and magnitude are inconsistent across studies. The most obvious conclusion to draw is that the observed effects are strongly paradigm dependent (notwithstanding the potential for artefacts in some experiments, where software or displays incapable of precise control of stimuli have been used). Furthermore, given that prey move in many different ways, including slow or rapid flight, direct flight or sharp or slow turns (see Brodie, 1992), and that they may be large or small relative to a predator, short or long, slender or fat, it seems incautious to assume that transverse or longitudinal striped patterning would operate against all predators in the same way. Finally, it can be difficult to separate the effect of a pattern on detection and interception when the experimental task is simply to click on a target. One way to circumvent this issue might be to use a tracking task, with continuous monitoring of either eye movements or cursor position; initially, any accuracy metric would be contaminated by both detection and interception but, as the tracking continued, it should be influenced only by the latter.
Comparative studies have the potential to uncover aspects of ecology that favour the evolution of dazzle but, generally, evidence that the observed patterns function as dazzle is lacking except, perhaps, in the study of striped lizards. The most coherent set of studies to date, by Murali, Kodandaramaiah and colleagues (Murali & Kodandaramaiah, 2016, 2018; Murali et al., 2018; Kodandaramaiah et al., 2020), combine comparative studies with estimates of perceptual bias and capture success. However, the latter two involve humans looking at abstract versions of the lizard colour patterns on a computer screen; an assessment of the effect of the real patterns on the evolutionarily relevant predators is still lacking. For example, comparative evidence indicates that longitudinal striping is characteristic of short lizards (Murali & Kodandaramaiah, 2018), although the evidence to show that natural predators direct their attack to the tail in these species is scant, so it would be useful to conduct experiments with artificial prey and natural predators to explore this relationship further, perhaps using easy-to-watch predators, such as raptors, attacking longitudinally striped, transverse-striped or variously striped artificial prey, along the lines of Powell’s (1982) classic, but largely forgotten, red-tailed hawk–weasel model experiments. The key here is to make detailed observations on whether the predator overshoots or attempts to strike behind the prey, thereby implicating misperception of speed or trajectory. In general, a good way forwards would be to allow laboratory experiments to inform those carried out in natural systems: the former can be used to constrain the parametric space for the latter, maximizing the chance of homing in on the most important aspects of stimulus and behaviour.
Trajectory Estimation
There has been only one paper on the effect of surface patterns on trajectory estimation, in two dimensions, with angled stripes causing biases in perception that vary strongly with speed of motion (Hughes et al., 2017). These effects require further investigation, using a variety of experimental models to unravel the complexity and robustness of the perceptual biases created. The ecological validity of any effects also needs to be established, because no animals with this sort of patterning have been proposed to benefit from predators misdirecting attacks (in contrast to the widespread occurrence of parallel or orthogonal stripes). Such animals may exist, but the effect needs to be linked to a plausible example in nature. Presumably, one that moves in three dimensions would be more likely, although an aperture effect (Adelson & Movshon, 1982) could work well in two dimensions (see the section above on ‘Potential underlying mechanisms’): for example, the movement of a striped snake moving under leaf litter is potentially ambiguous.
Range Estimation
There have been no experimental studies on the effect of coloration on range estimation. It is plausible that there could be an effect via size misjudgement, seen in humans, caused by stripes and other internal texture. There has been one study of human observations of striped and unstriped free-living ungulates, which failed to find any effect (Caro, 2016).
‘Motion dazzle’, as previously defined by Stevens et al. (2008), refers to the effect of coloration on perceived speed and trajectory. However, if the function of dazzle coloration is to interfere with interception, then distorting range estimation could also be important. Indeed, the effect of high-contrast repetitive patterns on optical range finders in World War I was discussed at the time (Taylor, 2016). Dazzle markings might affect the perception of target length (Jackson et al., 1976) and target area (Cott, 1940), both of which could influence estimates of range and thereby interfere with attempted interception of a moving target. Note that these effects on size perception, in addition to the use of orientation cues to predict future movement, might occur for both moving and stationary targets; the latter possibility has already been raised in the context of dazzle in plants (Lev-Yadun, 2014). Stevens et al.'s (2008) motion dazzle could be reframed as patterns that interfere with interception in a broader sense, potentially encompassing stationary targets as well as moving ones, and distortions of perceived range in addition to those of speed and trajectory. Whether that reframing is useful awaits research to show that dazzle coloration on static objects has effects on the estimation of range or future speed and trajectory.
Three-Dimensional Trajectories
The majority of experimental studies have used two-dimensional targets in plan view. While this might be relevant for avian predators attacking terrestrial prey, do the same effects occur with three-dimensional trajectories? Very few experiments have addressed this (e.g. Hämäläinen et al., 2015; Zlotnik et al., 2018) and, even in these, the predator’s viewpoint and movement at the point of attack were unknown. Some clues could be obtained using a three-dimensional gaming set-up and human predators (Hogan et al., 2017b), but this would require combination with observations of predation events in the natural world.
Background and Movement
The effect of the properties of the background is, as yet, under-investigated. Background complexity has been shown to affect detectability (Dimitrova & Merilaita, 2010, 2012; Xiao & Cuthill, 2016; Murali et al., 2021; Rowe et al., 2021), and so the environment across which artificial prey move could be manipulated in order to determine whether dazzle is more effective in some situations than others: for example flat vs. rugose surfaces, static vs. dynamic backdrops, aquatic environments, or under canopy vs. in open spaces.
Both predator and prey motion might affect the effectiveness of dazzle. Presenting moving artificial prey to predators with different styles of hunting would begin to address this (e.g. ambush vs. stalking vs. coursing predators). In addition, we currently have no idea of the relative efficacy of dazzle mechanisms in preventing successful attack in different media (air, water and land), which is unfortunate because these affect both sensory and locomotory aspects of predator–prey interaction.
Fixation and Attention
The effects of fixation and attention remain unexplored. For example, unpatterned stimuli minimize localization accuracy in peripheral vision (Smart et al., 2020): what implications does this have for the role of dazzle across the visual field? Visual acuity changes across the retinae of most predators, generally with a central area of higher resolution: this could imply that some dazzle patterns are resolved only in central vision, and therefore might never become attended to and foveated if they appear initially in the periphery. Experiments with stimuli presented both foveally and in the periphery would address this issue.
Underlying Neurophysiology
There has been no investigation into the neural underpinnings of dazzle. Santer (2013) recorded from movement-detector neurons in locusts when presented with looming patterned stimuli but, although the paper's title included the term ‘motion dazzle’, the study actually concerned target detection, not velocity estimation. In humans, most of the relevant processing presumably occurs in cortical area V5 (or its equivalent in other species). This area responds to potential motion in humans (Kourtzi & Kanwisher, 2000), which raises the intriguing prospect that dazzle could have an effect even when a target is static, so long as the possibility of movement is implied. Such an effect could potentially distort any calculation of target range for a subsequent interception attempt, especially given that the direction and magnitude of any implied motion would not necessarily be assessed accurately. Note that if such effects were to be thought of as dazzle, then deceptive patterns, such as eyespots, could also be included because they give false information about the location of the head (Kjernsmo et al., 2016), and hence the probable direction of any escape path. We do not make this claim, although we accept that a case could be made for it.
A motion signal can be recovered with a ‘delay and compare’ unit, and this is the standard structure for motion processing since it was introduced by Reichardt (1961) in the context of insect vision. The principle is that the outputs from two sensors aimed at different spatial locations, one with a delay relative to the other, can be compared to give a motion signal. The distance between the locations and the duration of the delay determine the speed tuning of the mechanism (i.e. its gradient in space-time).
Thinking in terms of dazzle, what would disrupt the processing of such a mechanism? There are no obvious routes to consistent speed distortion; while contrast can have a measurable effect on perceived speed, that effect is not consistent (see the section above on ‘Potential underlying mechanisms’) and, for some situations, simply disappears (Hall et al., 2016). In contrast, the idea that so-called ‘motion streaks’ caused by fast-moving objects could be a cue to direction of movement (Geisler, 1999) suggests that stripes might give rise to systematic distortions, and there is some behavioural evidence for this (Hogan et al., 2016a, b; Hughes et al., 2017). A final route to disruption of motion mechanisms is the addition of noise to the motion signal via dynamic information, e.g. flicker (Georgeson & Scott-Samuel, 1999) or the changing hues of iridescence (Pike, 2015), which could plausibly arise from the interplay of dappled lighting and salient surface texture. This noise can be thought of as potentially adding false correspondences over time to a delay and compare mechanism, thereby diluting the true motion signal.
Beyond Vision
Finally, we suggest that researchers consider the prospect of dazzle in the non-visual domain. Rattlesnakes use auditory signals to distort apparent range (Forsthofer et al., 2021); are there animals that make false Doppler shift sounds to make the predator think they have already passed? We see no logical reason that falsifying perception of speed, trajectory and range should be restricted to the visual domain.
CONCLUSIONS
Our key point is that only studies of perceptual biases can show whether an effect on interception is actually caused by dazzle. In addition, only studies of capture success can show whether a particular pattern's effect genuinely influences interception, and hence fitness. The need to make a link between perception and ecologically relevant outcomes also applies to experiments on humans, as it is well established that what holds for visual tasks is not necessarily true for motor tasks (Milner & Goodale, 1995). For example, some illusions distort visual judgements, but these effects disappear when observers make an immediate pointing action towards the stimulus (e.g. Bridgeman et al., 2000).
So far, the majority of experimental studies (of both perception and capture) have used humans. While they can be valuable model animals, not least because they can be given precise instructions, thereby improving experimental control, we still need to know whether other animals are affected in the same way. Furthermore, some classic visual illusions are inconsistent in their effects on other species (Feng et al., 2017). It is crucial to test predator species that have been proposed as a selective force on prey with putative dazzle patterns.
In addition, recording and analysing predator–prey interactions in the natural environment is a crucial step that will help to bridge the gap between the laboratory and the real world. The stimuli used in experimental studies have generally been fairly abstract (for some examples, see Fig. 1B–G). Those used in future research should mimic patterns seen on animals in nature in order that they can be more easily linked with the ecologically valid situations which they aim to model and illuminate.
Future experimentalists and observers of behaviour should not lose sight of the fact that contrasting coloration may have several functions acting simultaneously or sequentially in the predator–prey sequence (Valkonen et al., 2020). Dazzle should not be conflated with other anti-predator strategies, or an underlying mechanism simply assumed without rigorous tests of misjudgement of speed, trajectory or range estimation.
Research into dazzle is currently split between perception and outcome, single and multiple targets, and direct tests and ecological correlates. This implies that, a priori, it is unlikely that studies will generate the same findings or that this should be expected of them. It will be useful to frame future studies explicitly within these categories at the outset of research.
Finally, the term ‘dazzle’ should be used to describe an effect, not the external appearance of an animal.
SUPPORTING INFORMATION
Additional supporting information may be found in the online version of this article on the publisher's website:
Figure S1. PRISMA flow diagram, summarizing the processing of possible studies of dazzle coloration.
ACKNOWLEDGEMENTS
N.E.S.-S. and I.C.C. were supported by Biotechnology and Biological Sciences Research Council (BBSRC) grant BB/S00873X/1. S.R.M. was supported by a CASE studentship from the Engineering and Physical Sciences Research Council (EPSRC) and QinentiQ plc. S.R.M. performed the literature search; I.C.C. carried out the analyses; N.E.S.-S., I.C.C. and T.C. wrote the manuscript. We thank the members of CamoLab (www.camolab.com) for stimulating discussion, and Simcha Lev-Yadun and an anonymous referee for their helpful comments.
DATA AVAILABILITY
Data are available in the University of Bristol Research Data Storage Facility at: doi:10.5523/bris.1t0o5h87r5pxs2u16e3sy2ihkb
REFERENCES
Author notes
Samuel R. Matchette Current address: Department of Zoology, University of Cambridge, Cambridge, UK