-
PDF
- Split View
-
Views
-
Cite
Cite
Mario Vallejo-Marin, Avery L Russell, Harvesting pollen with vibrations: towards an integrative understanding of the proximate and ultimate reasons for buzz pollination, Annals of Botany, Volume 133, Issue 3, 1 March 2024, Pages 379–398, https://doi.org/10.1093/aob/mcad189
- Share Icon Share
Abstract
Buzz pollination, a type of interaction in which bees use vibrations to extract pollen from certain kinds of flowers, captures a close relationship between thousands of bee and plant species. In the last 120 years, studies of buzz pollination have contributed to our understanding of the natural history of buzz pollination, and basic properties of the vibrations produced by bees and applied to flowers in model systems. Yet, much remains to be done to establish its adaptive significance and the ecological and evolutionary dynamics of buzz pollination across diverse plant and bee systems. Here, we review for bees and plants the proximate (mechanism and ontogeny) and ultimate (adaptive significance and evolution) explanations for buzz pollination, focusing especially on integrating across these levels to synthesize and identify prominent gaps in our knowledge. Throughout, we highlight new technical and modelling approaches and the importance of considering morphology, biomechanics and behaviour in shaping our understanding of the adaptive significance of buzz pollination. We end by discussing the ecological context of buzz pollination and how a multilevel perspective can contribute to explain the proximate and evolutionary reasons for this ancient bee–plant interaction.
INTRODUCTION
Bees, which depend on pollen to feed their larvae, have shaped the evolutionary history of the flowers they pollinate, sometimes giving rise to elaborate floral forms (Darwin, 1862; Barrett, 2010; Stephens et al., 2023). Some pollen-rewarding flowers have morphologies that physically conceal pollen from floral visitors, requiring bees that use vibrations to extract the protein-rich pollen grains. This interaction has given rise to the phenomenon of buzz pollination. During buzz pollination, bees apply vibrations produced with their thoracic muscles to shake pollen out of tubular floral structures, most often anthers that open through small apical pores (Fig. 1). Buzz pollination involves the interaction between potentially more than half of all bee species (Cardinal et al., 2018) and perhaps 20 000 species of plants across dozens of plant families, including economically important crops such as tomato (Solanum lycopersicum, Solanaceae), kiwifruit (Actinidia deliciosa, Actinidiaceae) and blueberries (Vaccinium spp., Ericaceae) (Buchmann, 1983; De Luca and Vallejo-Marin, 2013).
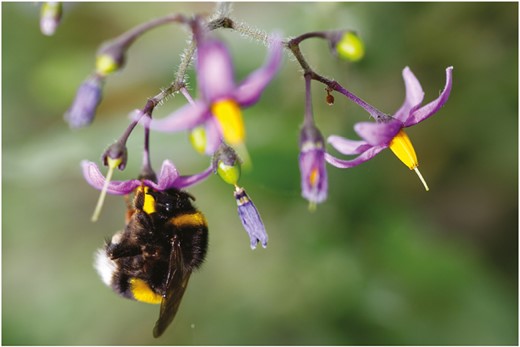
A bumblebee (Bombus terrestris, Apidae) in the stereotypical posture taken when vibrating a buzz-pollinated flower (Solanum dulcamara, Solanaceae). The bee is grabbing the base of the yellow anther cone with its mandibles, while curling its body into a C-shape around the anther cone. The bee uses the indirect flight muscles in its mesosoma to generate vibrations that are transmitted through the body of the bee, including its head, to the anthers where the vibrations result in pollen grains being ejected through small apical pores and onto the bee.
Although buzz pollination is common and geographically widespread, the use of vibrations to remove pollen from certain flowers went unnoticed by early pollination biologists. Observations on the pollination biology of buzz-pollinated flowers date back to Sprengel (1793), yet there appears to have been some confusion about how bees were removing pollen from them (reviewed in Teppner, 2017). The first unequivocal evidence linking pollen release with the production of vibrations by visiting bees is probably Swedish botanist C. A. M. Lindman’s (1902) work describing the pollination of Brazilian species of Senna alata (Fabaceae) (Teppner, 2017). Early work in buzz pollination established that vibration-producing bees were important, if not essential, pollinators of plants in which the pollen remains inside tubular anthers that open through small apical pores (i.e. poricidal anthers) (Osorno-Mesa, 1947; Rick, 1950; Michener, 1962; Linsley and Cazier, 1963; Macior, 1964; Buchmann, 1974; Bowers, 1975; Thorp and Estes, 1975; Buchmann et al., 1977), and recognized these vibrations as a major mechanism by which bees gather pollen from flowers (Buchmann and Hurley, 1978; Thorp, 1979). Vogel (1978) placed buzz pollination into the wider evolutionary context of transitions from nectar-producing to nectarless, pollen-only flowers, defining the Solanum-type flower and linking their evolution to a close physical interaction with bees. A series of studies by Buchmann and colleagues in the 1970s to late 1980s kickstarted the modern study of buzz pollination by bringing together the description of a widely evolutionary convergent set of floral traits thought to be associated with poricidal anthers (Buchmann, 1983), detailed analysis of bee’s vibratory behaviour (Buchmann et al., 1977; Buchmann, 1985; Buchmann and Cane, 1989), pollination ecology (Buchmann, 1974; Buchmann and Buchmann, 1981) and the first biophysical model of buzz pollination (Buchmann and Hurley, 1978). The study of buzz pollination has recently seen renewed interest from a wide range of researchers and, in the last decade, the number of studies tackling buzz pollination through a variety of approaches including biomechanical experiments (e.g. Arroyo-Correa et al., 2019; Bochorny et al., 2021; Jankauski et al., 2022a), behavioural ecology (Russell et al., 2017; Switzer and Combes, 2017; Wilkins et al., 2022), pollination and community ecology studies (Anderson et al., 2015; Solís-Montero et al., 2015; Mesquita-Neto et al., 2018; Kemp et al., 2022; Delgado et al., 2023), chemical ecology (Russell et al., 2016; Vega-Polanco et al., 2023a), biophysical modelling (Hansen et al., 2021; Jankauski et al., 2022b), phylogenetic and evolutionary analyses (Cardinal et al., 2018; Dellinger et al., 2019a, 2023; Melo et al., 2021; Hilgenhof et al., 2023), and the dissection of the economic and conservation implications of buzz pollination (Switzer and Combes, 2016; Rego et al., 2018; Cooley and Vallejo-Marin, 2021; Vega-Polanco et al., 2023b) has greatly increased.
In this review, we address the proximate and ultimate explanations for buzz pollination using Tinbergen’s levels of analysis framework (Bateson and Laland, 2013). We first discuss the proximate mechanism and ontogeny of buzz pollination in both bees and flowers. We then focus on the fitness consequences and evolutionary implications of buzz pollination for both partners. We also attempt to show how integrating across levels of analysis can be a powerful tool to identify and fill in major gaps in our knowledge. Finally, we discuss the ecological context of buzz pollination, particularly from the perspective of how community composition may affect buzz pollination interactions. Our goal is to contribute to a more multilevel understanding of this fascinating interaction between flowering plants and bees.
PROXIMATE EXPLANATION: THE MECHANISMS AND ONTOGENY OF BUZZ POLLINATION
Bees – mechanisms
Bees use powerful muscles in their thorax to produce vibrations in a variety of behavioural contexts, including during flight, communication, defence (also called alarm, hissing or escape buzzes), excavation of nests and pollen collection (Hrncir et al., 2008; Vallejo-Marin, 2022). These vibrations are powered by contractions of large muscles that occupy the majority of the bee’s thorax, called the indirect flight muscles (Dudley, 2000) (Fig. 2). In both bees and flies, these muscles power the flapping of the wings during flight via an indirect mechanism, in which muscle contraction causes deformation of the thorax, and indirect actuation of the wing via a complex hinge mechanism (Pringle, 1949; Dickinson and Tu, 1997). Importantly, indirect flight muscle contraction and wing flapping can be decoupled, allowing bees and flies to vibrate their thorax without deploying their wings (Vallejo-Marin, 2022). The vibrations of the thorax are caused by two sets of muscles acting antagonistically. The first set (dorsoventral muscles, DVM) is attached to dorsal and ventral plates of the bee exoskeleton, and contraction of these muscles causes the thorax (or, more properly, the mesosoma) to compress in the dorsal–ventral axis. The second set (dorsal-longitudinal muscles, DLM) run along the metasoma and their contraction causes lateral compression of the thorax (Fig. 2). The DVM and DLM contract in alternation, and thorax deformation causes one pair of muscles to contract (e.g. the DVM) when the other stretches (e.g. the DLM), and vice versa. The indirect flight muscles are of a special type called stretch activated (Deora et al., 2017). Stretch-activated muscles have the property of being able to spontaneously contract (i.e. without additional neuron input) in response to being stretched (Josephson et al., 2000). The combination of being stretch activated with the alternate deformation of the bee’s thorax means that the indirect flight muscles can produce rhythmic contractions at frequencies higher than the rate at which motor neurons stimulate them (Josephson et al., 2000). The physiological properties of the bee’s muscles coupled with the elastic properties of their thorax give bees the capacity to produce thoracic vibrations at relatively high frequencies and decouple them from wing flapping.
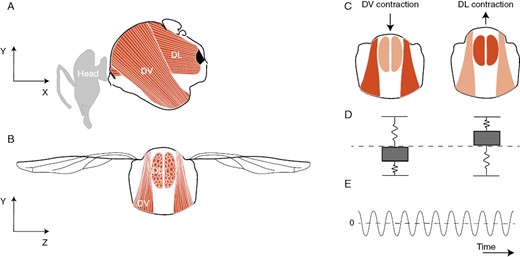
Vibrations produced during buzz pollination are powered by the stretch-activated indirect flight muscles located in the mesosoma of a bee. (A, B) A pair of dorsoventral muscles (DVM) run from the tergum (dorsal) to the sternum (ventral) sides of the mesosoma. A pair of dorsal longitudinal muscles (DLM) runs longitudinally along the mesosoma. (C) When the DVM contract (shown here with a darker colour), the tergum moves down and deforms the thorax longitudinally causing the DLM to stretch. The stretch-activation response of these muscles then causes the DLM to contract (shown with a darker colour), elevating the tergum, deforming the thorax dorsoventrally and causing the DVM to stretch. The stretch-activation response then causes the DVM to contract again, restarting the cycle. The stretch-activation response results in cycles of alternating muscle contraction in response to each electrical input from a motor neuron, allowing bees to produce vibrations of higher frequency than the rate of firing of their motor neurons. (D) Mechanical representation of the power muscles as a simplified mass-spring system. The two springs in parallel correspond to the DLM and DVM. (E) The rhythmic contraction of the power muscles results in oscillatory behaviour, visualized here as relative displacement of the surface of the thorax over time.
The oscillation of the bee’s thorax can be mechanically represented as a mass–spring system with two springs, with each spring capturing the contraction or stretching of the DVM and DLM (Fig. 2C). The alternate contraction of the DVM and DLM cause rhythmic oscillation of the thorax in three dimensions (X, Y, Z). The oscillatory displacement of the thorax surface around its resting point is actuated by the force produced by the DVM and DLM and mediated by the mass and stiffness of the thorax (Lynch et al., 2021; Casey et al., 2023). Recent experimental work with carpenter bees (Xylocopa californica) has shown that although thoracic vibrations are produced in three dimensions, the force amplitude of the vibrations is not the same in all directions (Jankauski et al., 2022a). Jankauski and colleagues showed that the force of vibrations along the dorsoventral axis (Y axis in Fig. 2A, B) are on average 250 % and 150 % as large as the force of vibrations along the two other axes (X and Z axes, respectively; Fig. 2A, B). These mechanical considerations of the vibrations produced by bees are important for understanding and interpreting empirical measurement of bee vibrations, and to help develop hypotheses of how bee traits might be related to the characteristics of the vibrations that they can produce.
The characteristics of non-flight bee vibrations have been traditionally analysed through the sound they produce. Both flight and non-flight vibrations produce sound when the wings and/or exoskeleton interact with the surrounding air. However, sound is an incidental by-product of these mechanical oscillations and does not appear to be functional in mating (Conrad and Ayasse, 2015), flight or pollen extraction (King and Buchmann, 1996), although it could still be functionally important in defence buzzes where sound could help startle or warn predators (Moore and Hassall, 2016), and perhaps in some other communication contexts (Hrncir et al., 2005). During buzz pollination, the mechanical properties of the vibration determine patterns of pollen release from flowers (De Luca et al., 2013), and thus it is desirable to characterize these mechanical properties in bees. At least some sound properties can adequately capture the mechanical characteristics of bee buzzes. For example, the temporal dynamics (e.g. the duration and pattern of buzz production) and the vibration frequency (number of cycles per second, Hz) can be accurately estimated from sound recordings (De Luca et al., 2018). However, the mechanical amplitude of bee vibrations is poorly captured by its sound amplitude (De Luca et al., 2018), and often sound recordings are made on relative scales that make comparison among studies difficult or impossible. Therefore, fully characterizing the mechanical properties of bee buzzes requires the use of sensors that allow acquisition of the duration, frequency, and absolute amplitude components of vibrations, such as accelerometers, force and displacement transducers, and laser vibrometers (reviewed in De Luca and Vallejo-Marin, 2022). This full characterization of bees’ vibrations is important for determining how different vibration properties affect their functional consequences, such as the amount of pollen released during buzz pollination.
The capacity to produce these vibrations is expected to be influenced by bee size, mass, morphology and physiology. Yet, we know relatively little about how the enormous variation in these traits and the degree to which they scale with one another (e.g. Danforth, 1989; Kendall et al., 2019) affect the type of vibrations bees can produce. Theoretical models of insect flight predict that insects with smaller wings require higher frequency wingbeats to achieve enough lift during flight (Pringle, 1957; Dudley, 2000; Deora et al., 2017). When body and wing size scale allometrically, we can predict that wingbeat frequency should be negatively correlated with body size (Deora et al., 2017). Indeed, there is a strong negative association between bee body size and wingbeat frequency during flight (Burkart et al., 2011; De Luca et al., 2019). In contrast, it is less clear how the frequency of non-flight vibrations should covary with body size and mass (which often scale allometrically, Kendall et al., 2019). Considering the vibrating thorax as a mass–spring mechanical system (Lynch et al., 2021; Casey et al., 2023) (Fig. 2), we expect that an increase in body mass would reduce the natural frequency of the thorax (San Ha et al., 2013). If non-flight vibrations are produced at frequencies near the natural frequency of the thorax, as is hypothesized to occur during flight (Jankauski, 2020), we would predict that body size and buzz frequency should also be negatively correlated. This prediction does not appear to hold as the frequency of pollination buzzes appears to be weakly and variably related to bee size in analyses both within (Pritchard and Vallejo-Marin, 2020) and between species (Burkart et al., 2011; De Luca et al., 2014, 2019; Arroyo-Correa et al., 2019; Rosi-Denadai et al., 2020), and the relationship changes if multiple measurements of body size are included (e.g. wet body mass and intertegular distance) (Corbet and Huang, 2014; Switzer and Combes, 2017). Although more data and a standard approach to body mass are needed, the observed weak and variable association between body size and frequency of non-flight vibrations raises the possibility that non-flight vibrations are driven beyond the resonance properties of the thorax (Hrncir et al., 2008), at least as predicted by changes in body mass.
The relationship between body size and amplitude has been explored less often, in part due to the difficulty in obtaining amplitude measurements as described above. All else being equally, we would expect that body size should be positively related to vibration amplitude. This is because larger bees should have proportionally larger indirect flight muscles. Because muscle power output is proportional to the cross-sectional area of the muscle (Ellington, 1985; Klowden, 2013), we would predict that vibration amplitude changes as a power function of body size (Clemente and Dick, 2023). Intriguingly, studies measuring the amplitude of pollination and defence buzzes using laser vibrometry or accelerometers on captive bumblebees (Bombus terrestris and B. ignitus) found no association between body size (intertegular distance) and vibration amplitude (Arroyo-Correa et al., 2019; Pritchard and Vallejo-Marin, 2020). In contrast, a multi-species comparison of defence buzzes across 22 bee taxa from Scotland found the expected strong positive association between thorax width and acceleration amplitude measured with a miniature accelerometer, showing that larger species produce higher amplitude buzzes (Vallejo-Marín and Vallejo, 2020). Additional empirical and theoretical developments on the relationship between bee traits and vibration properties are urgently needed to determine the functional consequences of variation in bee form.
Bees – behaviour and ontogeny
Although the basic mechanism by which non-flight buzzes are produced appears to be conserved across behavioural contexts (Vallejo-Marin, 2022), their characteristics and coordination with other motor components of a given behaviour can differ dramatically. While buzzing in some behavioural contexts appears nearly stereotyped within species (i.e. a repetitive behaviour, largely unmodified with experience), in other behavioural contexts the vibration characteristics are highly labile. For example, buzzes recorded during the courtship of many bee species are typically stereotyped in terms of duration of individual buzzes, fundamental frequency, peak amplitude and pattern, often having a narrowly constrained fundamental frequency, duration and peak amplitude, and a regimented sequence of buzzes (Alcock et al., 1978; Alcock and Buchmann, 1985; Alcock, 2013; Conrad et al., 2018; Russell et al., 2018a). Likewise, the very short warning buzzes used by honeybees during the waggle dance are similarly stereotyped (Pastor and Seeley, 2005). Buzzes used by queen and worker honeybees in inter-group communication (i.e. piping, quaking and tooting, for swarming, emerging and mating processes, respectively) are more labile, but each type is distinguishable in frequency, amplitude, and duration of buzz types, and the order and pattern of buzz types, indicating substantial stereotypy nonetheless (Michelsen et al., 1986; Pratt et al., 1996; Thom et al., 2003; Schlegel et al., 2012).
By comparison, buzzes produced in alarm, aggressive interactions, and in pollen extraction appear to be much more labile, and considering each behavioural context separately, bees produce buzzes that can vary substantially in fundamental frequency, peak amplitude and the duration of individual buzzes (e.g. King and Buchmann, 1996; Kirchner and Röschard, 1999; Cane, 2014; De Luca et al., 2014; Pritchard and Vallejo-Marin, 2020). Yet while buzzes are comparably labile across these behaviours, it appears that there are discernible differences in buzz qualities among behavioural contexts. For instance, direct comparisons of buzzes produced in alarm and in pollen collection demonstrate that both peak amplitude and fundamental frequency are substantially higher and greater, respectively, for alarm versus pollen extraction buzzes (De Luca et al., 2014; Pritchard and Vallejo-Marin, 2020).
Behaviour is rarely fully effective at first expression and thus often requires modification by experience (Tierney, 1986; Papaj and Prokopy, 1989; Papaj et al., 2008). Buzzing to extract pollen is a complex behaviour that involves multiple components, including (1) vibrational characteristics of the buzz and (2) the motor routines to transmit the vibrations to the flower and to interact with flower structures (‘flower handling’) (Russell et al., 2016). All or some of these components could require experience to become fully effective (Russell et al., 2016). While learning is a ubiquitous mechanism by which changes in behaviour with experience occur (Kamil and Roitblat, 1985; Papaj and Prokopy, 1989; Barron et al., 2015; Hollis and Guillette, 2015), whether components of buzzing behaviour are learned is still poorly understood, and mostly based on studies using generalist bumblebees (Bombus spp.), thus focusing on just one of more than 40 independent evolutionary origins of this buzzing behaviour among bees (Cardinal et al., 2018).
Although, in principle, bees could also make their buzzes more effective at extracting pollen by modifying the vibrational characteristics of their buzzes with experience (e.g. amplitude, frequency and duration), evidence that vibrational characteristics of buzzes are modified with experience is weak. To date, only a single study has directly tested whether bees can learn to modify a vibrational characteristic (fundamental frequency) to acquire a pollen reward (Switzer et al., 2019). In their study, the authors used artificial flowers that automatically rewarded bees with pollen when they buzzed the flowers at the correct frequency ranges but found that bees did not learn to match the given fundamental frequencies. Observational studies have documented that bees visiting different species of plants produce vibrations with different properties, but this could be partly explained by bees with different vibrational characteristics assorting themselves among suitable plant types rather than by a behavioural adjustment of the vibrations produced by a single individual bee (Corbet and Huang, 2014). Adjustment of buzz duration and frequency of buzzes produced by individual bees has been shown in experimental visits of Bombus impatiens to different Solanum species (Switzer and Combes, 2017), but no adjustment on buzz frequency was observed in B. terrestris in other Solanum taxa (Pereira Nunes et al., 2021). In experiments following the same individual (B. impatiens and B. terrestris) over consecutive bouts of visitation, the length of individual buzzes, their fundamental frequency and/or their peak amplitude are observed to increase or decrease as bumblebees gain experience (Morgan et al., 2016; Russell et al., 2016; Whitehorn et al., 2017). However, changes in behaviour over time are not necessarily a result of experience and may be a result of ageing or simple changes in motivational state (e.g. a shift in physiology that results in the organism activating or modifying behaviour; e.g. hunger activating behaviours to obtain food) (Papaj and Lewis, 1993). Future work that directly quantifies the functional consequences of these behavioural changes in terms of pollen extraction will be required to disentangle which mechanisms are responsible for changes in vibrational characteristics of buzzes.
Effective transfer of energy from bee vibrations to the flower and successful pollen removal should depend on the motor routines used to extract pollen from the flower. Buzzing to extract pollen is frequently described as being a stereotyped behaviour that is tightly coordinated in time and space, with grasping by the mandibles and legs occurring prior to each buzz (De Luca and Vallejo-Marin, 2013). In bumblebees, the motor routine to transmit vibrations is spontaneously performed and functional at first expression on different kinds of flowers (Russell et al., 2016, 2017) (Fig. 3). This suggests that the ordering of these elements is key to the transfer of energy from the vibrations and to elicit pollen release (Russell et al., 2016). However, coordination of anther grasping and buzzing may not be as stereotyped in all bee taxa, suggesting that alternative means to transfer energy from vibrations to the flower and elicit pollen release may be just as effective. For example, in Amegilla murrayensis thoracic vibrations can occur before and after grasping the anther with the mandibles, which often results in the head rapidly tapping the anther (‘head banging’) (Switzer et al., 2016). Likewise, Protandrena bees have been observed to initially grasp the proximal part (near the filament) of the anther but slide toward the proximal end of the anther as they continue buzzing (‘buzz-milking’) (Cane and Buchmann, 1989). Furthermore, even if the buzzing motor routine is generally spontaneously performed and stereotyped, given that bees can assess pollen returns while buzzing (Buchmann and Cane, 1989; Russell et al., 2017), the possibility remains that bees may learn to modify when they use the buzzing motor routine in association with floral cues (Fig. 3).
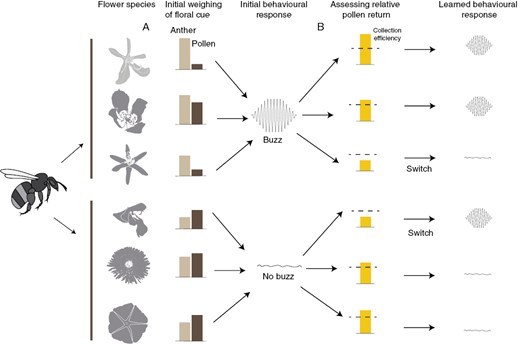
Floral cues that elicit buzzing to extract pollen probably involve (A) innate and (B) learned components. (A) Bees initially weigh two kinds of cues, anther chemical cues and pollen tactile cues (particle size) and attempt to extract pollen via buzzing when anther cues surpass some relative threshold of pollen cues (the proximate cause of buzzing). In contrast, if pollen cues outweigh anther cues, bees attempt to extract pollen without buzzing. These cues are common across angiosperms but are likely to vary in their absolute and relative strength among plant species, illustrated here as different types of flowers. After an initial attempt to extract pollen via a given motor routine, bees (B) assess the efficiency of pollen extraction (the functional consequence of their behaviour, e.g. rate of pollen collection) and hypothetically modify the pollen extraction motor routine accordingly. If efficiency is low using one pollen extraction motor routine, bees should learn to switch to the alternative motor routine and/or modify its components. Furthermore, bees might even learn to associate the appropriate motor routine with a given flower type, which would further optimize their foraging efficiency. Credit for floral profiles: A. Schmidt-Lebuhn, M. O. O. Pellegrini, M. McNair and M. Vallejo-Marin (available at: www.phylopic.org).
Diversity in floral form is often hypothesized to affect the effectiveness of flower handling behaviour (Laverty, 1980, 1994). Although poricidal flowers are at their simplest just a tubular structure containing pollen grains, the arrangement, shape and size of the stamens, as well as the morphology of the rest of the flower are highly diverse (Buchmann, 1983; Dellinger et al., 2019a) (see ‘The form of buzz-pollinated flowers’ below). For example, to intercept pollen expelled from anthers that are curved, a bee should position its body underneath the anther pores before buzzing, whereas a bee encountering multiple spread-out anthers should potentially attempt to grasp them all at once to maximize simultaneous pollen collection (Fig. 4). Therefore, to collect pollen more effectively, bees should respond to differences in floral form by modifying their flower handling behaviour with experience. Accordingly, when some anthers but not others are experimentally blocked, bees flexibly adjust to forage on unblocked anthers (Papaj et al., 2017). Furthermore, naïve bees use flower handling motor routines unique to each buzz-pollinated plant species and modify these handling routines substantially with experience (Laverty, 1980; A. L. Russell et al., in prep.). Taken together, there appears to be strong emerging evidence that buzzing behaviour involves both stereotyped and flexible components, including instrumental learning. Although floral cues associated with buzz-pollinated flowers are poorly understood, their floral displays appear to share many features with other animal-pollinated plants. For instance, floral displays of buzz-pollinated species are in part composed of generalized visual and olfactory cues that function in combination to influence pollinator behaviour (Connolly and Anderson, 2003; Russell et al., 2018b; Brito et al., 2021b). Different cues may also be functionally specialized to an extent. For instance, visual features of the corolla serve primarily to attract bees from a distance (Connolly and Anderson, 2003; Russell et al., 2018b; Brito et al., 2021b; Kemp et al., 2022). However, relative to other plant taxa, the androecium of buzz-pollinated plants – and perhaps pollen-rewarding plants generally (Dobson, 1987; Lunau, 2000; Nicholls and Hempel de Ibarra, 2017) – appear to play a much larger role in manipulating pollinator behaviour. Poricidal anthers often take on an attractive function and are presented prominently to floral visitors (Vogel, 1978; Connolly and Anderson, 2003; Russell et al., 2018b). They are commonly bright yellow regardless of the amount of pollen remaining in the anther, which is thought to allow them to display similar visual cues to floral visitors even when empty (Vogel, 1978; Buchmann and Cane, 1989). In some buzz-pollinated species, the stamens change colour substantially with age, which is thought to serve as a cue that bees can use to find unvisited flowers (Weiss, 1995; Larson and Barrett, 1999b). Both visual and olfactory cues associated with the stamens elicit landing by bees and stamen olfactory cues furthermore direct bees to the pollen-concealing anthers (Connolly and Anderson, 2003; Russell et al., 2018b; Anderson et al., 2023). Behavioural evidence for cues associated with the pollen reward of buzz-pollinated plants is scant (Burkart et al., 2014; Russell et al., 2018b; Solis-Montero et al., 2018; Vega-Polanco et al., 2023a). If removal of concealed pollen alters androecial scent and these changes can be perceived by bees, they could serve as a cue used by bees to assess the presence of the pollen reward (Burkart et al., 2014). On the other hand, buzz-pollinated plants presumably benefit by restricting the ability of bees to use pollen scent as a cue (Russell et al., 2018b). Bees that must land on the flower and physically contact the plant reproductive organs to assess pollen presence may transfer or pick up pollen in the process and thus contribute to pollination.
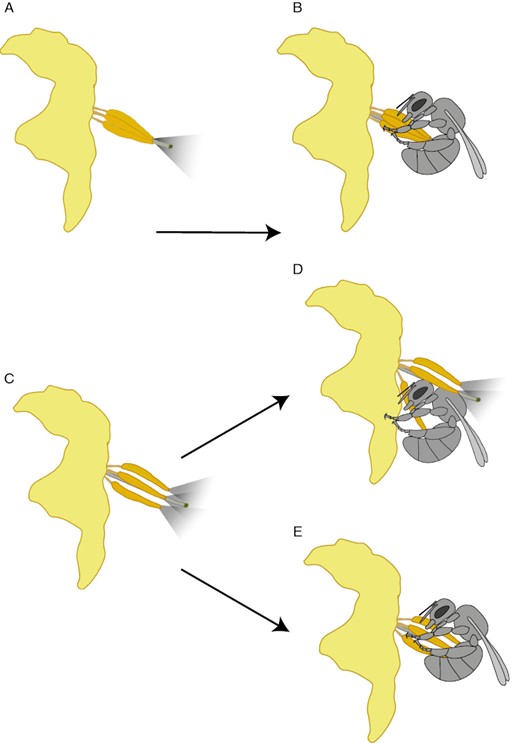
The functional consequences of different floral morphologies in buzz-pollinated plants are strongly mediated by pollinator behaviour. (A) Fused anther cones, where anthers are more or less rigidly joined into a conical structure, have evolved repeatedly in buzz-pollinated flowers. Fused anther cones transmit vibrations from bees more effectively to all anthers, releasing more pollen over a more concentrated area (A, B) compared to species with free anther architectures, where the anthers are not rigidly attached to each other (C). A free anther architecture may waste pollen if a bee does not contact the terminal pores of non-focal anthers (D). However, bees managing to bundle together multiple free anthers during buzzing effectively transform the flower into a functional cone reducing pollen wastage and increasing the precision of pollen placement on the bee’s body (E). Whether bees can hold multiple anthers together while buzzing or not should depend, in part, on the size of the bee relative to the flower.
Once bees arrive at the anthers, what floral cues enable bees that are capable of buzzing to determine whether to buzz? Given that buzzing is widely used by bees to extract pollen from flowers with diverse anther morphologies, including those that do not conceal their pollen within poricidal anthers (Buchmann, 1985; Russell et al., 2017) (Fig. 3), floral cues associated with buzzing behaviour are also probably not unique to buzz-pollinated plant species. Indeed, bees have been recorded buzzing the flowers of at least 89 species across 59 genera and 30 families that display their pollen on the surface of the anthers (Russell et al., 2017; A. L. Russell, online database). For bumblebees minimally, the mechanism that initially controls buzzing appears to involve a weighing of two types of common floral cues. Chemical cues associated with the anthers are, on their own, sufficient to elicit a buzzing response, whereas a pollen tactile cue (particle size) on the anthers will suppress a buzzing response, depending on the relative strength of each cue type (Russell et al., 2017; A. L. Russell et al., unpubl. res.). Consequently, on flowers that display their pollen on the surface of the anthers, bees initially extract pollen without buzzing, but begin to buzz with more regularity as pollen is progressively depleted (Raine and Chittka, 2007; Russell et al., 2017, 2021). Buzz-pollinated flowers, which do not have any pollen on the surface of their anthers, are thus immediately buzzed. Although anther chemical cues that elicit buzzing are chemotactile or of very low volatility and are found among diverse plant taxa, the identity of these compounds and whether they are shared across angiosperms remains elusive (Russell et al., 2017, 2018b). Furthermore, while the proposed mechanism controlling the initial expression of buzzing is simple and does not invoke massive convergent evolution or specialized floral signals, future work will be required to determine whether this same mechanism also controls the initial buzzing behaviour of a breadth of bee taxa.
Flowers – mechanisms
The form of buzz-pollinated flowers.
Flowers of buzz-pollinated plants typically conceal pollen from floral visitors in tube-like structures in which pollen can only be released through small apertures. These tube-like flowers can be formed in different ways. The canonical buzz-pollinated flower is nectarless and possesses anthers that at maturity open and release pollen only through small openings (pores or short slits), usually at the tip of anthers (Harris, 1906; Macior, 1970; Buchmann et al., 1977). These narrow-opening, tubular anthers are called poricidal anthers and represent an evolutionarily derived morphology compared to the anthers found in the majority of angiosperms in which mature anthers open through extensive longitudinal sutures and passively release pollen grains (Buchmann, 1983; Vallejo-Marin et al., 2010). The anthers of buzz-pollinated flowers tend to be relatively stiff (Todd, 1882), although formal measures of material properties of poricidal anthers, and particularly compared to their non-poricidal relatives, are lacking. Not all plants with poricidal anthers are buzz pollinated, and poricidal dehiscence combined with specialized slimy pollen characterizes fly-pollinated species in the families Rafflessiaceae, Hydnoraceae, Araceae and Viscaceae (D’Arcy et al., 1996; Endress, 1996).
Floral structures analogous to poricidal anthers have also evolved in buzz-pollinated flowers to form or extend tubes that conceal pollen and open only through small apertures. For example, some species of Senna and Chamaecrista (Fabaceae) have modified petals that surround poricidal anthers forming an extended tube from which pollen is released by vibrations (Amorim et al., 2019). In these species, vibrations cause pollen to be ejected from the anther pore into the tube formed by petal tissue from where it is channelled to the body of buzz pollinators. The ricochet mechanism by which pollen bounces on the surface of these petal tubes is not well understood, but these extended tubes might allow flowers to dispense pollen onto areas of the pollinator’s body where it is less likely to be groomed and more likely to contact the stigma of another flower (Wolfe and Estes, 1992; Westerkamp, 2004; Amorim et al., 2017). In other cases, such as in tomato (Solanum lycopersicum, Solanaceae) and some of its wild relatives, poricidal anthers may secondarily evolve wider or longer openings that extend along the longitudinal axis of the anther (Carrizo García et al., 2008). In this situation, a poricidal flower is reconstituted by the fusion of the anthers into a conical structure that opens through a narrow gap at the tip of the joined anther cone (Endress, 1996; Glover et al., 2004; Vallejo-Marin et al., 2022). In several species of the family Ochnaceae, in which most species have poricidal anthers, some taxa have secondarily evolved laminar tissue (staminodia) that forms a conical structure with a narrow apical aperture that surrounds the anthers (Kubitzki and Amaral, 1991). The anthers of these species can have pores or open through more elongated dehiscence, but the pollen is dispensed to buzz-pollinating visitors through the narrow apical gap of the conical structure formed by the staminodia (Kubitzki and Amaral, 1991).
A buzz-pollinated, poricidal flower can also evolve in species with regular longitudinally dehiscent anthers by other modifications of the corolla. For example, Amazonian species of Ternstroemia (Pentaphylaceae) have evolved a buzz-pollinated poricidal structure formed by petals surrounding longitudinally dehiscent anthers (Bittrich et al., 1993). In Ternstroemia dentata and T. laevigata, five out of the ten petals bend over the anthers, forming a conical structure that surrounds the anther and style and releases pollen via an apical pore (Bittrich et al., 1993; Amorim et al., 2019). Similarly, many species of alpine Pedicularis (Orobanchaceae) have evolved a narrow corolla that opens through a small apical aperture surrounding the longitudinally dehiscent anthers and forming a tubular structure that is buzz pollinated by bumblebees (Corbet and Huang, 2014). Other narrow-opening corollas that exclude large pollinators from directly touching the anthers have also evolved in some species of Vaccinium (Ericaceae) (Moquet et al., 2017). In these Vaccinium species, large floral visitors that are unable to directly contact the anthers apply vibrations to remove pollen (Cane and Payne, 1988).
Buzz-pollinated flowers are thus morphologically diverse and it is unclear whether they combine a distinctive set of traits to form a buzz pollination syndrome. In addition to pollen released from poricidal morphology by vibrations, buzz-pollinated plants are speculated to be associated with other floral traits, although few comparative studies exist. For instance, buzz-pollinated flowers produce large numbers of smooth-surfaced, small, relatively dry pollen grains (Vogel, 1978; Buchmann, 1983), though these traits are also shared with non-poricidal plant taxa that offer pollen rewards to bees (Roberts and Vallespir, 1978; Cruden, 2000; Roulston et al., 2000; Götzenberger et al., 2007). Plants with poricidal morphology may also converge to similar floral architectures, such as the Solanum-like or solanoid flower, in which anthers are presented in a conical structure at the centre of the flower (Vogel, 1978; Faegri, 1986). This Solanum-like morphology has convergently evolved across many angiosperm genera in disparate families (Harder and Barclay, 1994; De Luca and Vallejo-Marin, 2013; Vallejo-Marin et al., 2022), but flowers with poricidal morphology display an enormous diversity of floral architectures, as exemplified in the families Ericaceae, Fabaceae, Melastomataceae and Ochnaceae to name a few (Marazzi et al., 2007; Brito et al., 2016; Moquet et al., 2017; Dellinger et al., 2023). Furthermore, although most buzz-pollinated flowers are nectarless and offer pollen as the main reward to floral visitors, some buzz-pollinated flowers can also produce nectar and attract non-buzzing nectar foragers, such as in the Ericaceae (e.g. Vaccinium myrtillus), Boraginaceae (e.g. Trichodesma) and Melastomataceae (e.g. some species in the tribe Myconiae), increasing the range of floral visitors to these plants (Dukas and Dafni, 1990; Brito et al., 2017; Moquet et al., 2017). The functional consequences of offering nectar in buzz-pollinated flowers are little understood. However, when nectar is experimentally added to otherwise nectarless buzz-pollinated plants, visiting bumblebees (Bombus spp.) adopt a different posture when visiting the flower, therefore changing the place of contact of anthers and stigmas on the floral visitor (Tong et al., 2019). In summary, buzz-pollinated flowers are associated with a diversity of floral traits beyond the canonical nectarless flowers with poricidal anthers (Buchmann, 1983; Russell et al., 2016; Amorim et al., 2019), but a unifying feature appears to be the morphological restriction of pollen access to small openings, i.e. the evolution of poricidal flowers.
Biomechanical properties of buzz-pollinated flowers.
The general importance of the biomechanical properties of flowers for pollination is well recognized (Reith et al., 2006; Whitney and Federle, 2013; Timerman and Barrett, 2021), and buzz pollination is probably one of the clearest examples of why. The interaction between buzz-pollinated flowers and bees that use vibrations to collect pollen occurs through direct mechanical contact between bee and flower. Anther and flower traits such as their geometry, architecture and material properties should determine how floral structures respond to being vibrated (Vallejo-Marin, 2019a; Brito et al., 2020; Jankauski et al., 2022b). A potentially important characteristic of buzz-pollinated flowers is their natural frequency, defined as the frequency at which an object vibrates when a temporary force is applied to it. When a time-varying force (e.g. a bee’s buzz) is applied to an object (e.g. a stamen) with a frequency corresponding to the natural frequency of the system, the amplitude of the vibration will be maximized due to resonance. Bee vibrations ‘tuned’ to the natural frequency of flowers should enhance pollen collection (King and Lengoc, 1993; Harder and Barclay, 1994; Jankauski et al., 2022b) as higher amplitude vibrations result in higher rates of pollen release from buzz-pollinated flowers (De Luca et al., 2013). Indeed, pioneering analysis of the dynamic properties of the tomato anther cone (not individual anthers) showed that sound excitation at frequencies around the modelled natural frequency of the cone resulted in higher pollen release (DeTar et al., 1968). However, the predicted natural frequencies of the anther cone occur at high frequency ranges (4–21 kHz) and are thus beyond the fundamental frequencies of bee buzzes. Similarly, finite element analysis (FEA) modelling of individual stamens in kiwi fruit (Actinidia deliciosa, Actinidiaceae) suggests that the lowest natural frequency that could eject pollen occurs above 6 kHz and suggesting that bee-induced resonance does not play a role in pollen ejection in this species (King and Lengoc, 1993). However, empirical analyses of individual stamens of several species of Solanum (Pereira Nunes et al., 2021; Jankauski et al., 2022b) suggest lower natural frequencies that could overlap the range of values produced by bees. For example, in Solanum section Androceras, the first (lowest) natural frequencies of individual stamens range from 20 to 400 Hz (Pereira Nunes et al., 2021), and in S. elaeagnifolium FEA modelling reveals a first fundamental frequency of about 57–67 Hz (Jankauski et al., 2022b). The lowest of these stamen natural frequencies are probably below the range of bee buzzes (which for non-flight vibrations are ~80–500 Hz, M. Vallejo-Marin et al., unpubl. res.), but at least some of these overlap with the frequencies that bees can produce. Moreover, new FEA models incorporating the added mass of the bee to the vibrating flower show that the bee mass brings down upper natural frequencies to values (135–565 Hz) that are within the range of bee buzzes (Jankauski et al., 2022b).
Pollen release.
In principle, the duration, amplitude and frequency of the vibrations applied to the flower should influence how much pollen is released, but only a few studies have developed theoretical models to investigate patterns of pollen release in buzz-pollinated systems. Inspired by empirical measurements of pollen released per buzz (Buchmann et al., 1977). Buchmann and Hurley (1978) developed the first biophysical model of pollen release during buzz pollination. Their model assumed that pollen release from poricidal anthers is a function of the gain in kinetic energy of pollen grains as they elastically bounce against the internal wall of the anther (locule). In their model, an increase in the velocity of the vibrations applied to the anther translates to more pollen release from flowers. Recently, Hansen et al. (2021) developed a 2D anther model in which individual pollen grains interact with the anther walls as a ball on a billiard table and exit the rectangular anther through an apical pore. Numerical simulations of their model showed that pollen release was strongly related to the velocity amplitude of the applied vibrations. The duration of the vibrations should be a strong determinant of pollen release, but as the anther empties of available pollen, continuing to apply vibrations to the same anther should result in diminishing pollen returns (King and Buchmann, 1996; De Luca et al., 2013; Kemp and Vallejo-Marín, 2021; Tayal and Kariyat, 2021). The gradual release of pollen when vibrations are applied to a poricidal anther can be simply described by an exponential decay function (Kemp and Vallejo-Marín, 2021).
The first empirical study to systematically explore how vibration properties affect pollen release is probably King and Lengoc (1993). Studying the poricidal anthers of Actinidia deliciosa (kiwifruit), they measured pollen release using artificial vibrations of different acceleration and frequency magnitudes. Although acceleration amplitude was positively related to the amount of pollen released, frequency had no effect. King and Lengoc suggested vibrations could help break adhesive liquid bridges between pollen grains and allow them to be propelled out of the anther pore. Since then, multiple other studies using several plant taxa have demonstrated that the effects of variation in vibration frequency, within the range produced by bees, on pollen release are modest at best and can depend on whether amplitude characteristics are included as co-variates (e.g. Harder and Barclay, 1994; De Luca et al., 2013; Rosi-Denadai et al., 2020). In contrast, there is a strong positive relationship between vibration amplitude characteristics (including displacement, velocity or acceleration) and pollen release (e.g. Harder and Barclay, 1994; King and Buchmann, 1996; De Luca et al., 2013; Rosi-Denadai et al., 2020; Kemp and Vallejo-Marín, 2021), suggesting this is probably a general phenomenon, and thus making vibration amplitude a key parameter for buzz pollination.
The importance of flower morphology, including the internal anatomy of flowers, in converting floral vibrations into pollen release has been suspected since the early days of modern buzz pollination studies (Buchmann et al., 1977; Buchmann and Hurley, 1978). However, our current knowledge about the link between flower form and pollen release is very limited. A useful system to investigate the relationship between floral form and pollen release is species of Solanum that have undergone replicated evolutionary transition from large- to small-flowered taxa. Using artificial vibrations, Kemp and Vallejo-Marin (2021) showed that smaller flowers have higher rates of pollen release than their large-flowered ancestors, suggesting that transitions in size (and shape) have functional consequences for the dispensing schedule of pollen grains. In their study, anther wall area, but not pore size, was positively related to the rate of pollen release. This functional link between the internal properties of the anther locule and pollen release during buzz pollination suggests that changes in both external characteristics and internal anatomy are connected to the function of buzz-pollinated flowers. In species of Melastomataceae that produce morphologically distinct sets of anthers in the same flower with putative different functions (i.e. heterantherous), small anthers release pollen grains at a slower rate than large anthers following artificial vibrations (Dellinger et al., 2019b; Brito et al., 2021a). In addition to variation in anther shape and size, some buzz-pollinated species have stamens in which the connective tissue takes on elaborate morphologies of unknown function. Bochorny et al. (2021) showed that experimental removal of the twisting connective appendages of Huberia bradeana (Melastomataceae) is associated with reduced pollen removal but does not affect bee visitation. Given the widespread and puzzling diversity of stamen architectures in Melastomataceae and many other taxa, similar experiments have the potential to illuminate the functional consequences of floral morphology in buzz pollination. Development of theoretical models of pollen release in buzz-pollinated flowers may further help guide future tests of how floral morphology is expected to affect pollen release patterns.
Flowers – ontogeny: development and pollinator interactions
Pollen release in buzz-pollinated species is not only determined by morphological characteristics of the flower but also partly determined by dynamic processes regulating pollen availability in the flower and anther following anthesis. Anther dehiscence in buzz-pollinated species usually occurs simultaneously across all anthers within a flower (Dellinger et al., 2019b). However, several studies have shown that pollen release depends on flower age (time from anthesis). In Primula conjugens, older, virgin flowers release more pollen per buzz than recently opened ones (Harder and Barclay, 1994). The proportion of pollen released per buzz also increases with floral age in two species of Pleoroma (Melastomataceae) (Brito et al., 2021a), in Solanum douglasii and Solanum xantii (Solanaceae) (Buchmann et al., 1977), and in Senna reticulata (Fabaceae), but not in Adelobotrys adscendens or in Conostegia subcrustulata (Mealstomataceae) (Dellinger et al., 2019b). The mechanism explaining this age-dependent effect on pollen release could be associated with the gradual dehydration of the tapetum inside the anther and the release of pollen grains into the anther locule where they can then be removed by vibrations (King and Ferguson, 1994). King and Ferguson (1994) showed that young anthers of Actinidia deliciosa in which the pollen is covered by tapetal fluid do not allow pollen to be ejected as a result of vibrations. Adhesive interactions among pollen grains and between pollen and anther, as mediated by tapetal fluid, are thus good candidates for the mechanisms responsible for the age-dependent pattern of pollen release in at least some buzz-pollinated species.
Flower function (e.g. patterns of pollen release and placement of pollen on the pollinator’s body) is probably mediated by the behaviour of the pollinator while on the buzz-pollinated flower (Wilkins et al., 2022). How a bee handles the flower will determine the position of the bee’s body when the vibrations are applied, and thus the location, amount and frequency of pollen deposition and stigma contact. For example, in buzz-pollinated species in which anthers are loosely arranged, some pollinators may be able to manipulate the flower to gather multiple stamens simultaneously to effectively vibrate them as a unit (Fig. 4). To date, little work has been conducted to quantitatively evaluate how pollinator behaviour affects the functional consequences of different floral morphologies of buzz-pollinated plants.
ULTIMATE CONSEQUENCES: THE FITNESS CONSEQUENCES AND EVOLUTION OF BUZZ POLLINATION
An evolutionary explanation of buzz pollination requires investigating how proximate mechanisms translate into fitness differences (Arnold, 1983) and how they shape the evolutionary history of traits associated with buzz pollination. In this section we discuss the fitness consequences of deploying vibrations to extract pollen, including the energetic costs of deploying floral vibrations. We then discuss why the use of vibrations to collect pollen evolved, and why some bees have gained and lost the capacity to perform this behaviour on flowers. We next discuss the fitness consequences incurred by buzz-pollinated flowers for both male and female components of plant fitness. We present the evolutionary context in which buzz-pollinated flowers evolve and discuss hypotheses to explain why they evolve. Our focus in this section is on the ultimate consequences and explanations for buzz pollination.
Bees
Despite substantial attention to characterizing the proximate causes of buzzing behaviour, the functional consequences for bees have been barely explored. Part of this certainly lies with the difficulty of measuring fitness consequences for bees. Most bee species are solitary and may require unique conditions to fly, nest and mate, not well suited to captivity. Many bumblebee species are easier to rear in captivity and thus have served as a model system for nearly all experimental studies on buzzing behaviour. However, because bumblebees are eusocial, fitness can only be measured at the colony level, which requires assessing the cumulative benefit of all workers in terms of numbers of successfully reproducing queens and drones (Plowright and Laverty, 1984; Alaux et al., 2005; Raine and Chittka, 2008). Thus, for most behavioural experiments, even the simplest proxy for fitness – number of new queens and drones produced – is prohibitive in terms of time and funds.
Nonetheless, there are tantalizing clues that buzzing to extract pollen has costs and benefits for bees that could affect their fitness. In terms of benefits, most obviously, buzzing enables bees to access concealed pollen from poricidal angiosperms, which is largely inaccessible to other pollinators, as well as to collect pollen more efficiently from many other non-poricidal plant species (Russell et al., 2017; Cardinal et al., 2018). In terms of costs, buzzing is thought to be energetically expensive relative to other types of pollen collection behaviour (Buchmann, 1983; Russell et al., 2017; Kemp et al., 2022). Although the energetic costs of buzzing to extract pollen have yet to be measured, they are probably at least as high as flight costs given their mode of production and the type of vibrations produced (Russell et al., 2017; Kemp et al., 2022; Vallejo-Marin, 2022). Furthermore, buzzing may carry costs or benefits that depend on the thermal environment, given that prolonged use of the indirect flight muscles causes a rapid increase in thoracic body temperature (Heinrich, 1975, 1993). Accordingly, in hot environments, bees that use buzzing to extract pollen may be at risk of exceeding their thermal maxima, whereas in cold environments, buzzing might enable bees to thermoregulate more effectively when not flying. Addressing these hypotheses will not only require biophysiological experiments, but also studies that examine the drivers of buzzing and non-buzzing bee biogeography.
Finally, although not all behaviour must necessarily be adaptive, a full understanding of why behaviour changes requires integrating proximate and ultimate perspectives (Losey, 1993; Alcock and Sherman, 1994; Bergman and Beehner, 2022). Changes in foraging behaviour are frequently predicted to increase the collection rate and total rewards collected from flowers (Laverty, 1980; Raine and Chittka, 2008), yet there is still little direct evidence of these functional consequences for buzz pollination mutualisms. Despite substantial focus in the literature, changes in vibrational characteristics of buzzing have yet to be convincingly linked to changes in pollen collection by bees. Nonetheless, when pollen is rendered inaccessible by experimentally gluing poricidal anthers shut, bees consistently increase the peak amplitude of their buzzes, suggesting a functional role for this behavioural change (Russell et al., 2016; Switzer et al., 2019). On the other hand, while the motor routines involved in handling buzz-pollinated flowers have received very little attention, instrumental learning of flower handling motor routines in other plant–bee mutualisms has been linked to changes in resource acquisition (e.g. Raine and Chittka, 2007, 2008) and probably also affects pollen collection in buzz pollination mutualisms.
Fitness effects have the potential to drive broader evolutionary patterns and bees have evolved many features as a response to evolutionary pressures imposed by plants, including morphological, behavioural and physiological adaptations that enable them to collect, store and digest pollen (Thorp, 2000; Danforth et al., 2019). Ancestral trait reconstruction by Cardinal et al. (2018) showed that the ability to apply vibrations to flowers was probably already present in the earliest bees in the early Cretaceous 100–145 Mya, prior to the evolution of any poricidal plant lineages (A. L. Russell et al., unpubl. res.). This evidence suggests that the capacity to use vibrations to remove pollen from flowers evolved before the earliest poricidal flowers (Endress, 1996; Russell et al., 2017).
The capacity to buzz flowers has been proposed to be a major driver of bee diversification, given that buzzing bees can collect pollen more efficiently and from a greater variety of plant species relative to bees incapable of buzzing (Cardinal et al., 2018). Unfortunately, pollen collection behaviour including buzzing to extract pollen is only rarely reported, even for taxa that are likely to be composed entirely of buzzing species, and thus high-resolution phylogenetic inference is correspondingly limited. Much more work is especially needed in documenting the occurrence or absence of buzzing outside of North American and European bee species. Similarly, while coevolution between buzzing bees and buzz-pollinated flowers has been frequently posited (Macior, 1964; Buchmann, 1983; Proenca, 1992), evidence for any adaptations that buzzing bees may have evolved in response to buzz-pollinated plants has yet to be described.
Buzzing is also conspicuously absent in many bee taxa and is estimated to have been lost on average 66 times across the Apoidea (Cardinal et al., 2018). Multiple hypotheses have been given to describe losses or absences of buzzing, including purported physiological costs of buzzing, ineffectiveness of buzzing by small bees, specialization on plant species that do not require buzzing, competitive exclusion by more effective buzzing bees and the evolution of alternative comparably efficient pollen extracting behaviours (Cardinal et al., 2018). However, none of these various hypotheses have been tested (for a related discussion of hypotheses of why pollen-feeding hoverflies do not buzz pollinate see Vallejo-Marín and Vallejo, 2020), and unfortunately pollen foraging behaviours are still poorly documented in most bee taxa (Thorp 1979, 2000; Portman et al., 2019), precluding comprehensive phylogenetic analyses.
Flowers
Field measurements of plant fitness and variation in floral traits linked to buzz pollination are essential to evaluate their adaptive and evolutionary significance. Yet, a major gap in the study of buzz pollination is the scarcity of studies that have linked traits associated with buzz pollination to direct measurements of plant fitness (Appel and Cocroft, 2023). Many studies of the functional consequences of flower traits associated with buzz pollination use proxies of fitness such as pollinator visitation (Brito et al., 2016; Russell et al., 2016), pollen release (Buchmann et al., 1977; Harder and Barclay, 1994; Larson and Barrett, 1999a; De Luca et al., 2013; Dellinger et al., 2019b; Kemp and Vallejo-Marín, 2021), pollen placement on the bee’s body (Vallejo-Marin et al., 2009; Dellinger et al., 2019b; Tong et al., 2019; Russell et al., 2021) and pollen receipt on stigmas of experimental plants (Russell et al., 2021; Wilkins et al., 2022). Although these fitness correlates are theoretically expected to influence the pathway to fitness (Minnaar et al., 2019), the extent to which these indirect measurements scale to variation in fitness in wild populations of buzz-pollinated plants is mostly unknown.
Potential consequences of buzz pollination for components of plant fitness.
As discussed above, the evolution of buzz-pollinated flowers is closely related to the evolution of poricidal flowers. The small openings of poricidal anthers from which pollen can be released suggests that buzz-pollinated flowers could function as a pollen dispensing mechanism (Harder and Barclay, 1994; Larson and Barrett, 1999a). Pollen dispensing mechanisms influence the proportion of available pollen removed by pollinators (Harder and Barclay, 1994). When pollinators are abundant, increased pollen removal from bee-pollinated flowers is expected to yield diminishing fitness returns. This is because increased pollen removal from a flower induces pollinator grooming, thus removing pollen from the pollination process, as well as because the surface of pollinators can become saturated with pollen and additional pollen grains suffer from either falling off the pollinator or being buried under successive layers of deposited pollen (Harder and Wilson, 1994; Minnaar et al., 2019; Moir and Anderson, 2023). Thus, it can be hypothesized that when visits by potential pollinators are not limiting, mechanisms restricting pollen removed per visit should be favoured by natural selection. To date, no studies with buzz-pollinated plants have investigated the extent to which pollen dispensing mechanisms such as anther pore size or age-dependent pollen availability vary within species. Inter-species comparisons of plants with and without poricidal flowers in different plant families suggest that at least at this level, poricidal anthers are associated with more gradual pollen release (as a proportion of total pollen in the flower) than in plants with longitudinal anthers (Pereira Nunes and Vallejo-Marin, 2022). Between buzz-pollinated species, Solanum taxa with larger anther pores are associated with higher rates of pollen release upon artificial vibrations (Kemp and Vallejo-Marín, 2021).
The dispensing mechanism of poricidal anthers may be costly for female fitness in situations where pollinator visitation is low. When pollinator visits are rare, restricting the amount of available pollen removed by a visitor may negatively impact the availability of pollen transported by the bee for fertilizing other flowers. Larson and Barrett (1999a) showed that higher pollen removal in populations of the buzz-pollinated herb Rhexia virginica (Melastomataceae) reduced the level of pollen limitation (female fitness pathway) in the population. Their study revealed that at the end of anthesis, about half of the pollen produced remained inside the anthers, suggesting that in situations of low pollinator visitation, pollen dispensing may be costly for both male and female components of fitness.
Although vibrations are required to release significant amounts of pollen in buzz-pollinated flowers, the extent to which vibrations are necessary to receive pollen on stigmas and fertilize ovules is less clear. Some species of buzz-pollinated Cassia, Senna and Chamaecrista have stigmas inside a chamber or cavity in the style covered by trichomes. In these species, vibrations might be required for successful pollination (Dulberger et al., 1994; Marazzi et al., 2007). In a seminal experimental study of Chamecrista chamaecristoides which has a stigma cavity covered by trichomes, Arceo-Gomez et al. (2011) showed that when pollen is applied to the style without vibrations the flower does not set fruit. In contrast, application of pollen in combination with artificial vibrations to the style resulted in 30 % seed set. Vibrations do not seem to be required for fruit set in buzz-pollinated species with more glabrous stigmas, such as Solanum, but clearly more studies investigating the relationship between vibrations and female fitness in buzz-pollinated species are needed.
How and why do buzz-pollinated flowers evolve?
Addressing why buzz pollination evolves in plants is closely associated with understanding the evolution of poricidal anthers (Buchmann, 1983). In general, poricidal anthers are evolutionarily derived from longitudinally dehiscent anthers, presumably by failure of the opening of the stomium, which results in dehiscence being restricted to apical regions (Fig. 5A). Evolution of poricidal dehiscence from longitudinally dehiscent ancestors characterize entire genera (e.g. Solanum, 1250 species; Hilgenhof et al., 2023; Senna, 350 species; Marazzi et al., 2006) or entire families (e.g. Melastomataceae with about 5150 species; Melo et al., 2021), but transitions to poricidal morphologies are much harder to observe within genera or species. For example, in the genus Myrcia s.l. (Myrtaceae), some species have longitudinal dehiscence while others open only through apical pores (Proenca, 1992), and given the widespread occurrence of longitudinal anthers in this genus, it is likely that these represent evolutionarily derived instances of the transition from longitudinal to poricidal anthers. Within-species variation in poricidal morphology would be a prime opportunity to investigate the fitness consequences of transitions from longitudinal to poricidal anthers.
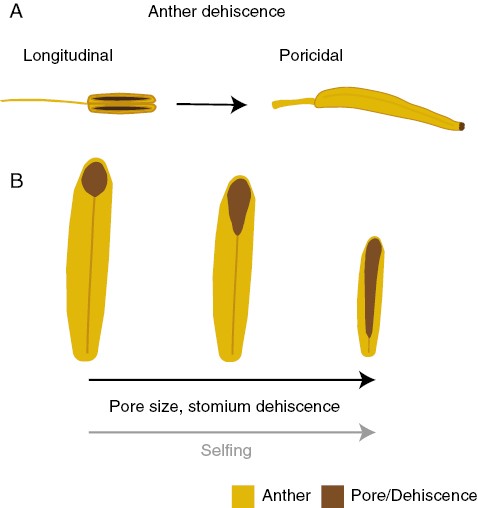
(A) The evolutionary transition to poricidal stamens involves failure of the longitudinal dehiscence of the anther, resulting in dehiscence being restricted to small apical regions of the anther. These apical dehiscence regions can form pores or small terminal slits, from which pollen grains can leave the anther. Often, this transition is accompanied by shortening of the filament, enlargement and stiffening of the anther, and a large increase in pollen production. (B) In contrast, the evolutionary breakdown of poricidal stamens could be associated with shifts in mating system from outcrossing to selfing. In some Solanum species, the transition to self-pollination appears to be associated with a reduction in the size of stamens and, importantly, an increase in relative dehiscence area, which could facilitate pollen release and autonomous selfing even in the absence of buzz pollinators.
A potentially useful system to investigate the evolutionary consequences of variation in anther dehiscence involves the breakdown of poricidal anther morphologies, where anther pores yield increasingly longitudinal dehiscence (Fig. 5B). This breakdown of poricidal anther morphologies appears to be common within lineages of poricidal flowers. For example, in Solanum, multiple evolutionary transitions from large flowers with narrow pores to smaller flowers with elongated pores have been documented in closely related species in the section Androceras. Similarly, in wild and cultivated tomatoes (Solanum section Lycopersicon), the anthers open through elongated anther pores or slits, which regain a poricidal function because the individual anthers are joined together with trichomes to form a cone that becomes the poricidal structure (Glover et al., 2004; Carrizo García et al., 2008). In Solanum, the widening of anther pores could be related to reduced reliance on vibrations to release pollen grains, and presumably an increased capacity to self-pollinate in the absence of buzz-pollinating bees, but this has not been formally tested. Overcoming the limitation of pollen release in the absence of vibrations is an additional challenge faced during the transition from outcross to self-pollination in buzz-pollinated plants, where it might not be sufficient to bring stamens and stigmas close together as in other plants where pollen is passively released as the anther matures. Where the transition to completely rely on buzz pollination occurs along this continuum of anther dehiscence variation, and the degree to which buzzing behaviour is expressed, is unknown and further work in this area would provide insights into how and why buzz pollination evolves.
Several hypotheses have been proposed to explain why buzz pollination evolves. These non-mutually exclusive hypotheses can be grouped into whether they explain buzz pollination as an evolutionary response to: (1) protect flowers from overexploitation by pollen-seeking floral visitors, (2) control pollen dispensing, (3) localize the deposition of pollen grains on the pollinator’s body or (4) protect against abiotic stress (Fig. 6). First, the protection set of hypotheses posit that tubular anthers with narrow openings can effectively limit the type of floral visitors that can extract pollen rewards (Fig. 6A). For example, pollen removal from poricidal anthers using legs or mouthparts is probably slow and inefficient as the animal (usually an insect) must probe the small pair (one per theca) of microscopic openings in each anther (Thorp, 1979). Even destructive harvesting through chewing the anther as done by beetles and some bees (e.g. Trigona) is a time-consuming process (Renner, 1983). Thus, poricidal anthers may work as a filtering mechanism restricting the type of floral visitors that can efficiently extract pollen from them, effectively rendering as a specialized pollination system for bees using vibrations to harvest pollen and discouraging visits by other types of visitors (butterflies, flies, birds, bats, non-buzzing bees) (Sun and Rychtář, 2015; van der Kooi et al., 2021). However, poricidal anthers alone do not seem to be an effective filter against vibration-foraging pollen thieves. Wild populations of buzz-pollinated plants can have high incidences of pollen thieves, removing large numbers of pollen grains using vibrations without delivering pollen to the stigma (Solís-Montero et al., 2015; Mesquita-Neto et al., 2021). Second, poricidal anthers may evolve as dosing mechanisms controlling pollen removal by pollen-collecting bees (Harder and Barclay, 1994) (Fig. 6B). Most buzz-pollinated plants are nectarless, and bees are notoriously effective at harvesting pollen from flowers (Hargreaves et al., 2009). Poricidal anthers may enable flowers to reduce the amount of available pollen removed in each visit, as discussed above (‘Potential consequences of buzz pollination for components of plant fitness’).
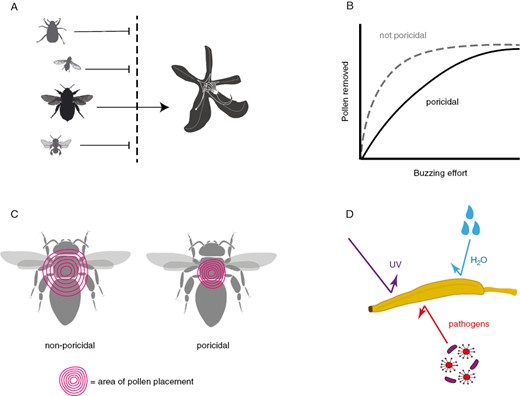
Conceptual diagram of four types of hypotheses for why poricidal flowers evolve (see text for detailed explanation and references). (A) In response to overexploitation by pollen-seeking floral visitors, poricidal flowers evolve as a filtering mechanism to restrict the type of floral visitors that can easily access pollen rewards, leaving bees capable of harvesting pollen with vibrations as the main pollinators. Note that some visitors could still remove pollen without vibrations (e.g. by chewing through the anther or gathering pollen from the pore), but this is inefficient. (B) Poricidal flowers evolve as pollen-dispensing mechanisms. Poricidal flowers might function to reduce the rate of pollen removed by pollen-seeking floral visitors compared to species with longitudinally dehiscent anthers and non-poricidal flowers. (C) Poricidal flowers evolve to increase the precision of pollen placement on the body of floral visitors. (D) Poricidal flowers evolve as a mechanism to protect pollen grains against abiotic (ultraviolet radiation, rain) and/or biotic agents (pathogens). Credit for bee profiles: M. Broussard, E. Price, A. Wilson (available at: www.phylopic.org) and M. Vallejo-Marin.
A third hypothesis suggests that the evolution of poricidal flowers with a solanoid floral architecture enables the localization of pollen placement on the pollinator (i.e. the precision of pollen placement, Armbruster et al., 2009), and reduces pollen loss during transport (Harder and Barclay, 1994) (Fig. 6C). Although Harder and Barclay focused their discussion on the solanoid flower rather than on poricidal anthers or buzz pollination, the evolution of poricidal anthers might facilitate further changes in floral architecture including the evolution of the solanoid flower. Artificial vibrations applied to an individual anther or set of anthers result in a broadly spread-out cloud of pollen (Dellinger et al., 2019b), potentially challenging this precision hypothesis (Larson and Barrett, 1999a). However, during buzz pollination, bees usually press their body closely to the anther pore (Osorno-Mesa, 1947), and at this short distance more precise pollen placement is possible. Consistent pollen placement and pick up in buzz-pollinated flowers can be facilitated by bilaterally symmetric flowers (or asymmetric ones in the case of enantiostylous flowers with reciprocally deflected anthers and style), which can induce pollinators to alight in a consistent position among visits, potentially also increasing the accuracy of pollen transfer (Endress, 1999; Armbruster, 2022; Nevard and Vallejo-Marin, 2022). Monosymmetry and asymmetry commonly evolve in buzz-pollinated flowers (Endress, 2012). In fact, the evolution of heteranthery, where different sets of anthers within the same flower specialize on feeding and pollinating functions, relies on relatively accurate and consistent pollen placement on the body of the pollinator (Luo et al., 2008; Vallejo-Marin et al., 2009; Saab et al., 2021). Heteranthery and enatiostyly are often associated with nectarless, buzz-pollinated flowers (Vallejo-Marin et al., 2010; Barrett, 2021), lending support to the idea that accurate and precise pollen placement is possible in buzz-pollinated flowers. Moreover, the morphology of the anther tip and shape and size of the anther pore (or the tip and pore of the poricidal structure in other types of poricidal flowers) might dictate the spread and direction of the ejected pollen grains (Amorim et al., 2019) and could thus be under selection under this hypothesis. Some species of Senna (e.g. S. acururensis, S. aversiflora and S. tonduzzi) have long and elaborated anther tips that could function to fine-tune the deposition of pollen on the pollinator’s body (Marazzi et al., 2007). These long, apical anther tubes have been documented in a handful of buzz-pollinated species in Fabaceae, Melastomataceae, Solanaceae, Ericaceae and Elaeocarpaceae (Marazzi et al., 2007; Hilgenhof et al., 2023).
Finally, an unexplored hypothesis is that keeping pollen grains inside a tubular structure confers them protection against abiotic stressors such as ultraviolet (UV) radiation (Koski and Ashman, 2015), rain and desiccation (Fig. 6D). The anther wall, or the corolla tube that covers anthers in other poricidal flowers, is a physical barrier protecting pollen grains. In many buzz-pollinated flowers, the anthers contain UV-absorbing pigments, which may help reduce harmful direct UV irradiation to pollen grains. If so, we might even expect the concealed pollen of buzz-pollinated flowers to lose adaptations to UV radiation (Lunau, 1995). A variation of this hypothesis is that the tubular structure of anthers may also be a physical barrier against microbial pathogens that might otherwise come into direct contact with pollen, for example during floral visits. To our knowledge, stress–pathogen protection hypotheses have never been tested in buzz-pollinated flowers.
THE ECOLOGICAL CONTEXT OF BUZZ POLLINATION
The ecological interaction between buzz-pollinated plants and buzz-pollinating bees is functionally specialized but taxonomically diffuse. Buzz pollination systems are functionally specialized as bees need to deploy a specific behaviour (buzzing flowers for pollen) to efficiently remove pollen, and poricidal flowers probably require vibrations for their reproduction. However, there seems to be a very weak to no requirement for specific taxa of bees or flowers to successfully engage in buzz pollination. Wild populations of buzz-pollinated flowers are often visited by diverse buzz-pollinating bees that vary widely in size and taxonomic composition (e.g. Larson and Barrett, 1999b; Houston and Ladd, 2002; Brito and Sazima, 2012; Huang and Shi, 2013; Mesquita-Neto et al., 2018, 2021; Rosi-Denadai et al., 2020; Dellinger et al., 2021; González-Vanegas et al., 2021). For example, across its native range, Solanum rostratum is buzz pollinated by bees ranging from very small (Augochlora sp., Halictidae) to large (Bombus, Apidae) to enormous (Xylocopa sp., Apidae) (Todd, 1882; Bowers, 1975; Solís-Montero et al., 2015). In its introduced range in China, S. rostratum is effectively cross-pollinated by a completely different taxonomic set of buzz-pollinating bees that are still functionally similar in size and buzzing behaviour to those in the native range, and which yield a similar outcrossing rate (Vallejo-Marin et al., 2013; Zhang et al., 2017). From a bee perspective, there also seems to be limited taxonomic specificity on which buzz-pollinated plant species can be exploited, beyond the usual limitations imposed by what kind of pollen different types of bees can digest (Danforth et al., 2019). Native bees can and do visit non-native buzz-pollinated plants, and in some cases new ecological associations form in the introduced range of both taxa as has occurred in southern Florida during the invasion of the orchid bee Euglossa viridissima which buzz pollinates the invasive weed Solanum torvum (Liu and Pemberton, 2009). Buzz pollination thus is a functionally specialized interaction where taxonomic correspondence between bees and plants takes a secondary role.
Rather than taxonomic identity, a more important determinant of the ecological interaction between bees and buzz-pollinated flowers appears to be the relative sizes of bees and flowers and their behaviour on the flower. In a review of the visitors of buzz-pollinated plants, Delgado et al. (2023) showed that large-flowered species are visited by more species of bees than small-flowered taxa (see also Valadão-Mendes et al., 2022). Large-flowered plants are visited by both buzzing and non-buzzing bees of relatively larger size and, surprisingly, small-flowered species tend to be visited mostly by small buzz-pollinating bees. Non-buzzing bees visiting large flowers appear to act mostly as antagonists, removing pollen resources while failing to pollinate (Solís-Montero et al., 2015; Delgado et al., 2023). The reasons why large and non-buzz pollinating bees seem to avoid small flowers are unknown but they may involve increased difficulty of handling or buzzing small flowers, and energetic costs that exceed the reward returns (Pyke and Starr, 2021). From the perspective of the plant, bee size relative to the flower can affect the likelihood of pollen transfer to the stigmas. In S. rostratum, the relative fit between bee and flower size (i.e. the difference between thorax width and anther–stigma distance) affects the number of pollen grains deposited during buzz pollination (Solis-Montero and Vallejo-Marin, 2017). Bees that are the same size or larger than the separation between anthers and stigma transfer more pollen to the stigma than relatively smaller bees. The same pattern occurs in buzz-pollinated Chamaecrista ramosa where bees below a certain size fail to contact stigmas and pollinate flowers (minimum size threshold; Mesquita-Neto et al., 2021). This flower–bee size relationship means that vibration-foraging bees that are legitimate pollinators in one plant species can become pollen thieves in another one (Mesquita-Neto et al., 2018).
The diffuse interaction between buzz-pollinated plants and their vibration-foraging visitors is also sensitive to temporal and spatial variation in plant communities. In the South African buzz-pollinated plant and nectarless Cyanella hyacinthoides (Tecophilaceae), the number of pollinator species is independent of the composition of the plant community (Kemp et al., 2022). However, the rate of visitation to C. hyacinthoides is reduced when other plant species with more accessible resources are abundant. In this case, buzzing bees might be avoiding nectarless buzz-pollinated flowers due to the high energetic costs associated with producing vibrations to extract pollen from poricidal flowers, when they can acquire both pollen and nectar (needed to fuel continued foraging) at less energetic cost from nearby plant species. To entice pollinators, buzz-pollinated plant species may thus particularly benefit: (1) by flowering synchronously, (2) by offering higher quality rewards relative to synchronously flowering non-poricidal taxa and (3) by flowering before or after other non-poricidal taxa with more accessible resources. Thus, it is possible that for buzz-pollinated plants, the effect of the surrounding community on visitation is strongly influenced by both the type of rewards their competitors offer and how those rewards are offered to floral visitors.
CONCLUSIONS
Buzz pollination captures an intimate and ancient interaction between bees and flowers. Bees have evolved many features in response to the selective pressures imposed by foraging on floral resources, including adaptations to collect, transport and digest pollen grains (Thorp, 2000; Cardinal and Danforth, 2013; Danforth et al., 2019). However, the capacity to produce non-flight vibrations and deploy them on flowers is probably as old as bees themselves (Cardinal et al., 2018) and precedes the evolution of poricidal flowers. Why only some bee species buzz-pollinate flowers while others have never been observed using vibrations to harvest pollen is unknown and one of the big open questions in buzz pollination research. Buzz pollination belies a high degree of functional specialization where only bees capable of using vibrations can efficiently harvest pollen from flowers with poricidal morphologies, and where most poricidal flowers rely on vibration-producing bees to reproduce. However, this functional specialization contrasts with the ecologically diffuse and taxonomically generalist interaction between bees and poricidal flowers where the capacity to vibrate, the handling behaviour, and the relative size of flower and bee are more important than their taxonomic identity. Perhaps for this reason, to date, no convincing examples of one-to-one species co-evolution in buzz-pollinated systems have been documented.
A general evolutionary trajectory of buzz-pollinated flowers appears to be one in which the reliance of pollen as the main or only reward to floral visitors triggers the evolution of floral morphologies that limit pollen removal. Restricted pollen access then causes bees to deploy energy-demanding, complex motor routines that allow them to rapidly harvest pollen grains using vibrations further selecting for floral modifications that control pollen removal patterns. Poricidal flowers appear to be an evolutionary innovation that is associated with higher plant diversification rates (A. L. Russell et al., unpubl. res.). Moreover, buzz-pollinated plants are not adaptive dead-ends as previously thought, and evolutionary tinkering can yield shifts in pollinator type without losing poricidal anthers (Vallejo-Marin, 2019b). Such a change in pollinator type can be seen in the evolutionary shifts to a bellows-mechanism in flowers of perfume-rewarding species of some Solanum pollinated by male orchid bees (Sazima et al., 1993), and in the sugar-body rewarding species of the tribe Merianeae (Melastomataceae) pollinated by birds (Dellinger et al., 2014).
A more detailed understanding of buzz pollination will require a closer and explicit look at the interaction between buzzing bees and poricidal flowers rather than studying each partner separately. Achieving this will require an integrative approach where diverse fields come together including behaviour, biomechanics, physiology, phylogenetics, ecology and evolution. Studies of buzz pollination might be particularly timely given the variety of global stressors currently threatening bee pollinators, such as the potential effects of pesticide exposure on buzz pollination behaviour (Switzer and Combes, 2016; Whitehorn et al., 2017), effects of changing temperatures on bee activity (Woodard, 2017; Sepúlveda and Goulson, 2023) and effects of elevated carbon dioxide on pollen nutrient quality (Ziska et al., 2016). After more than a century capturing the curiosity of researchers, buzz pollination continues to be an exciting field with many opportunities to view fundamental biological questions through an interdisciplinary lens.
ACKNOWLEDGEMENTS
We would like to dedicate this manuscript to the memory of Paul A. De Luca. We thank Gitte Petersen for the opportunity to write this review, and the Associate Editor Rachel Spigler, Shuan-Quan Huang, Ze-Yu Tong and an anonymous reviewer for insightful comments on a previous version.
FUNDING
M.V.M. was partially supported by a Human Frontier Science Program research grant (RGP0043/2022).