-
PDF
- Split View
-
Views
-
Cite
Cite
Alex Williams, Besiana Sinanaj, Grace A Hoysted, Plant–microbe interactions through a lens: tales from the mycorrhizosphere, Annals of Botany, Volume 133, Issue 3, 1 March 2024, Pages 399–412, https://doi.org/10.1093/aob/mcad191
- Share Icon Share
Abstract
The soil microbiome plays a pivotal role in maintaining ecological balance, supporting food production, preserving water quality and safeguarding human health. Understanding the intricate dynamics within the soil microbiome necessitates unravelling complex bacterial–fungal interactions (BFIs). BFIs occur in diverse habitats, such as the phyllosphere, rhizosphere and bulk soil, where they exert substantial influence on plant–microbe associations, nutrient cycling and overall ecosystem functions. In various symbiotic associations, fungi form mycorrhizal connections with plant roots, enhancing nutrient uptake through the root and mycorrhizal pathways. Concurrently, specific soil bacteria, including mycorrhiza helper bacteria, play a pivotal role in nutrient acquisition and promoting plant growth. Chemical communication and biofilm formation further shape plant–microbial interactions, affecting plant growth, disease resistance and nutrient acquisition processes.
Promoting synergistic interactions between mycorrhizal fungi and soil microbes holds immense potential for advancing ecological knowledge and conservation. However, despite the significant progress, gaps remain in our understanding of the evolutionary significance, perception, functional traits and ecological relevance of BFIs. Here we review recent findings obtained with respect to complex microbial communities – particularly in the mycorrhizosphere – and include the latest advances in the field, outlining their profound impacts on our understanding of ecosystem dynamics and plant physiology and function.
Deepening our understanding of plant BFIs can help assess their capabilities with regard to ecological and agricultural safe-guarding, in particular buffering soil stresses, and ensuring sustainable land management practices. Preserving and enhancing soil biodiversity emerge as critical imperatives in sustaining life on Earth amidst pressures of anthropogenic climate change. A holistic approach integrates scientific knowledge on bacteria and fungi, which includes their potential to foster resilient soil ecosystems for present and future generations.
INTRODUCTION
Soil is essential for supporting life on Earth. The biodiversity of soil, however, is a threatened resource that is vulnerable to both climate change and intensive agroecosystem management (Lehmann et al., 2020; Guerra et al., 2021). Soil quality is critical to ecological balances and resource provision, so implementing measures to preserve and maintain soil is fundamental for the health of both managed and natural systems. With hundreds of thousands of taxa per gram of soil, complex microbial communities dominate soil biodiversity (Allison and Martiny, 2008). This consortia of microorganisms, also known as the soil microbiome, collectively perform key ecosystem processes including nutrient cycling and maintaining soil health.
The soil microbiome comprises bacteria, archaea, fungi, algae and protists and frequently consists of microhabitats that are linked by diverse intra- and inter-kingdom interactions (Braga et al., 2016; Berg et al., 2020). Due to their critical significance in the operation of terrestrial ecosystems, particularly plant growth, development and function, bacterial–fungal interactions (BFIs) are drawing increasing research attention. BFIs occur within complex microbial communities, existing in various plant and soil niches including the phyllosphere (the aerial part of the plant), the rhizosphere (the region surrounding the plant roots) and the bulk soil (the soil outside of the rhizosphere and not penetrated by plant roots) (Fig. 1). Under fluctuating environmental conditions, bacteria and fungi engage in complex interactions ranging from antagonism to mutualism. As a result, BFIs have significant impacts on the plant hosts and can augment growth, reproduction, transport/movement, nutrition, stress resistance and pathogenicity of the partners involved (Deveau et al., 2018). Understanding plant–microbe associations and their implications for plant and ecosystem functioning relies on a solid knowledge base regarding the dynamics between eukaryotic and prokaryotic organisms (Frey-Klett et al., 2011). It is noteworthy that, like plants, microorganisms in the soil such as mycorrhizal fungi can also recruit distinct bacteria forming a ‘mycorrhizosphere’, which is defined as the soil zone influenced by both roots and mycorrhizal fungi (Barea et al., 2002). Furthermore, another distinct suite of microbes, which provide enhanced nutrient mobilization and turnover, can be recruited directly to the ‘hyphosphere’, which is defined as the narrow region around fungal hyphae where conditions are different from the bulk soil due to hyphal exudates (Wang and Feng, 2021). In this review, we describe these BFIs with a focus on endosymbiotic fungi [specifically arbuscular mycorrhiza (AM) and Mucoromycotina ‘fine root endophytes’ (MFRE)] and collate recent findings obtained towards BFIs in the mycorrhizosphere. We include the latest advances regarding the role of interactions and mechanisms involved, the different outcomes for each partner and, ultimately, how these impact plant function. Finally we discuss knowledge gaps, emerging opportunities and applications of BFIs with respect to the mycorrhizal hyphosphere and function.
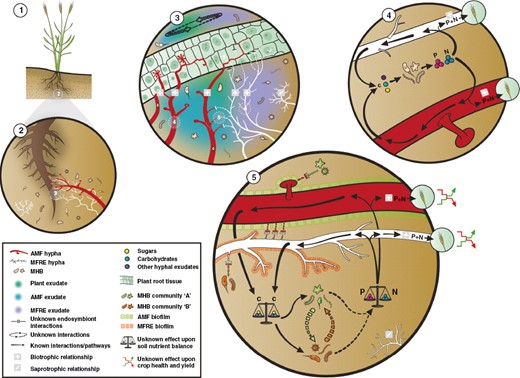
Step-wise overview of putative multipartite interactions between plant roots, mycorrhizosphere microbial communities and arbuscular mycorrhizal (AM) fungi and Mucoromycotina fine root endophyte (MFRE) fungi. 1. A sessile plant. 2. Exclusive MFRE fungi (white hypha) interactions are rare, often forming simultaneous associations alongside AM fungi (red hypha); diverse bacterial populations associate with fungi in and around the hyphae and this is termed the mycorrhizosphere. 3. Putative/unknown overview (dashed lines) of complex plant root and fungal hyphal exudate interactions. 4. Overview of potential bacterial interactions with both AM and MFRE fungi in terms of nutrient mineralization and transfer to host plant. 5. Summary panel of fundamental questions from mycorrhizal bacterial–fungal interactions (BFIs) including analysis of putative biofilms, effects of bacterial populations on nutrient mineralization and mycorrhizal function, bacterial–bacterial (B-B) interactions, fungal–fungal (F-F) interactions and BFIs.
Characterizing the mycorrhizosphere
Terrestrial plants can obtain vital nutrients through a number of pathways: directly through root uptake or through symbiotic interactions, such as mycorrhizal hyphal associations (Smith and Smith, 2011) or via N2-fixing bacteria (reviewed elsewhere, see Pii et al., 2015 and references therein). Based on their structure and function, four major mycorrhizal types have been identified: AM, ectomycorrhiza (EM), orchid mycorrhiza and ericoid mycorrhiza (van der Heijden et al., 2015). Due to their capacity to establish symbiotic interactions with ~80 % of land plant families (Tedersoo et al., 2020), AMs formed by the obligately symbiotic fungi of the phylum Glomeromycota are a crucial functional group of soil biota that can generally contribute to crop productivity and ecosystem sustainability (Smith and Read, 2008; Köhl et al., 2016). AM colonization of plant roots begins when the AM fungi sense chemical signals released by plant roots. The hyphae penetrate the root tissues and develop inter- and intra-cellularly, forming running hyphae, coils and arbuscules. The AM fungi also form vesicles that contain lipid reserves and which can be used by the fungus during times of scarcity (van Aarle and Olssen, 2003). In contrast to AM symbiosis, comparatively few plants live in symbiosis with EM fungi. EM symbiosis is established predominantly by basidiomycetes but also ascomycetes (Martin et al., 2016). The spores derived from the sporocarps of EM fungi germinate and inhabit specific types of lateral roots known as fine or short roots. These fungi’s hyphae extend into the surrounding soil, some passing through the epidermal and cortical cells and forming the Hartig net, which serves as the site for nutritional exchange between the fungal and plant cells. In addition to enhancing plant growth by facilitating the uptake of soil phosphorus (P) and other vital mineral nutrients, both AM and EM fungi possess ‘non-nutritional’ effects. These effects include stabilizing soil aggregates, preventing erosion, and mitigating plant stress caused by environmental factors such as drought and extreme temperatures (Smith and Read, 2008; Gianinazzi et al., 2010).
The acquisition and utilization of nutrients by plants, whether obtained directly through the root system or facilitated by mycorrhizal and/or other symbiotic associations, rely on the recruitment of specific groups of soil microbes. Previous research has predominantly examined the interactions between beneficial free-living soil bacteria and plants, commonly known as plant-growth-promoting rhizobacteria (PGPR). These bacteria have been the primary focus of most studies conducted so far. However, mycorrhizosphere and hyphosphere bacteria are likely to play a role in the success and outcome of plant-growth-promoting fungi. The interaction between mycorrhizal fungi and associated bacteria is complex and highly specific. The composition and function of the bacterial community can vary depending on the plant species – these compositions also differ between controlled laboratory and natural field conditions (Wang et al., 2016; Zhang et al., 2022). The relationships between AM fungi and hyphosphere microbiome members, and the outcome of their interactions on organic nutrient utilization and nutrient cycling, have only been investigated in a few studies so far, primarily using in vitro experimental setups assessing the effects of single or multiple bacterial genotypes (Jiang et al., 2021). For example, in in vitro culture, where AM fungi were manipulated, fructose secreted by hyphae served to increase bacterial phosphatase activity and shift bacterial community structure (Zhang et al., 2018a, b). To date, some well-designed mesocosm studies using root-free compartments, aiming to characterize AM fungal hyphosphere microbial communities, have been carried out. Using a range of non-sterile soils, Emmett et al. (2021) exposed root-associated AM fungi to preferentially recruit bacteria to their hyphosphere. Complementary techniques such as 16S rRNA gene amplicon sequencing, terminal fragment restriction length polymorphism (T-RFLP) analysis and 13CO2 pulse labelling have also led to the recognition of hyphal-associated phosphate-solubilizing bacteria (PSB; Wang et al., 2016). However, how these results translate to complex natural or agro-ecosystems in terms of plant growth and fitness is yet to be explored. Thus, our current knowledge of the functioning of the hyphospheric microorganisms and the underlying mechanisms of interactions between AM fungi and their associated microbiome is still largely incomplete (Jansa and Hodge, 2021; Zhang et al., 2022). In addition, little consideration has been taken regarding interaction with new emerging groups of mycorrhizal fungi such as MFRE fungi (Bidartondo et al., 2011; Field and Pressel, 2018; Hoysted et al., 2023). It is clear that BFIs play a critical role in plant host health, along with soil nutritional status, but research focus is required to identify further key players and their functional mechanisms.
Evolutionary origin of plant–fungal–bacterial interactions
In aid of continued identification of important BFIs, evolutionary insights can be gleaned from extant fungal endosymbiotic bacteria that live within hyphae. These endobacteria are widespread, occurring throughout the phyla Mucoromycota, Ascomycota and Basidiomycota, and range from obligate symbionts to facultative endofungal associates (Steffan et al., 2020; Table 1). Given that endobacteria are found in all three subphyla of the Mucoromycota, it is likely that the common ancestor of this early phylum evolved bacterial hosting abilities and thus also harboured endobacteria (Bonfante and Venice, 2020). This is supported by the Rhynie Chert, a fossil-rich sedimentary deposit from the early Devonian where putative bacterial colonies and swellings have been observed in plants which harbour AM-like fungal structures, e.g. Nothia (Taylor et al., 2003). The ability of putative bacteria and fungi to penetrate the cell walls of these Devonian plants and possibly overcome plant defences suggests it is likely that fungal endobacteria would have existed >410 million years ago. The function of early endobacteria may have been critical to fungal fitness, enough so that they have persisted to this day.
Bacterium . | Known fungal hosts . | Bacterial invasion characteristics . | Functional interaction . | References . |
---|---|---|---|---|
CaMg – Candidatus Moeniiplasma glomeromycotorum | AM Endogonales | Vertically transmitted endobacteria | – | Desirò et al., 2014; Lastovetsky et al., 2018 |
CaGg – Candidatus Glomeribacter gigasporarum | Gigasporaceae | Vertically transmitted endobacteria that expresses Type II, III and IV secretion systems and possesses vacB gene | Bacteria contain full operon for B12 vitamin synthesis | Ghignone et al., 2012; Lastovetsky et al., 2018 |
Burkholderia-related | AM | Vertically transmitted endobacteria | – | Lastovetsky et al., 2018 |
Mycoavidus sp. | Mortierella elongata | – | Bacterium relies on fungus for carbon and nitrogen | Li et al., 2017 |
Mollicute-related endobacteria | Benniella erionia | – | Bacteria provide fungus with increased resistance to Mn2+ concentrations | Lupini et al., 2022 |
Mycetohabitans rhizoxinica | Rhizopus microsporus | Vertically transmitted endobacteria with reduced genome containing few transcriptional regulators and quorum-sensing systems; and many transposons, virulence-related genes and putative effectors | Bacterium along with two Narnaviruses are needed for fungal sexual reproductive success. Bacterium produces a mycotoxin that allows fungus to be pathogenic | Partida-Martinez and Hertweck, 2005 |
Rhizobium radiobacter | Piriformspora indica | No reduced genome | Bacterium aids fungus in establishing plant–fungal symbioses | Guo et al., 2017 |
Ralstonia solanacearum | Ascomycota Mucoromycota Rhizoctonia solani | Produces lipopeptides to enter fungal cell walls of chlamydospores | – | Spraker et al., 2016 |
Bacterium . | Known fungal hosts . | Bacterial invasion characteristics . | Functional interaction . | References . |
---|---|---|---|---|
CaMg – Candidatus Moeniiplasma glomeromycotorum | AM Endogonales | Vertically transmitted endobacteria | – | Desirò et al., 2014; Lastovetsky et al., 2018 |
CaGg – Candidatus Glomeribacter gigasporarum | Gigasporaceae | Vertically transmitted endobacteria that expresses Type II, III and IV secretion systems and possesses vacB gene | Bacteria contain full operon for B12 vitamin synthesis | Ghignone et al., 2012; Lastovetsky et al., 2018 |
Burkholderia-related | AM | Vertically transmitted endobacteria | – | Lastovetsky et al., 2018 |
Mycoavidus sp. | Mortierella elongata | – | Bacterium relies on fungus for carbon and nitrogen | Li et al., 2017 |
Mollicute-related endobacteria | Benniella erionia | – | Bacteria provide fungus with increased resistance to Mn2+ concentrations | Lupini et al., 2022 |
Mycetohabitans rhizoxinica | Rhizopus microsporus | Vertically transmitted endobacteria with reduced genome containing few transcriptional regulators and quorum-sensing systems; and many transposons, virulence-related genes and putative effectors | Bacterium along with two Narnaviruses are needed for fungal sexual reproductive success. Bacterium produces a mycotoxin that allows fungus to be pathogenic | Partida-Martinez and Hertweck, 2005 |
Rhizobium radiobacter | Piriformspora indica | No reduced genome | Bacterium aids fungus in establishing plant–fungal symbioses | Guo et al., 2017 |
Ralstonia solanacearum | Ascomycota Mucoromycota Rhizoctonia solani | Produces lipopeptides to enter fungal cell walls of chlamydospores | – | Spraker et al., 2016 |
Bacterium . | Known fungal hosts . | Bacterial invasion characteristics . | Functional interaction . | References . |
---|---|---|---|---|
CaMg – Candidatus Moeniiplasma glomeromycotorum | AM Endogonales | Vertically transmitted endobacteria | – | Desirò et al., 2014; Lastovetsky et al., 2018 |
CaGg – Candidatus Glomeribacter gigasporarum | Gigasporaceae | Vertically transmitted endobacteria that expresses Type II, III and IV secretion systems and possesses vacB gene | Bacteria contain full operon for B12 vitamin synthesis | Ghignone et al., 2012; Lastovetsky et al., 2018 |
Burkholderia-related | AM | Vertically transmitted endobacteria | – | Lastovetsky et al., 2018 |
Mycoavidus sp. | Mortierella elongata | – | Bacterium relies on fungus for carbon and nitrogen | Li et al., 2017 |
Mollicute-related endobacteria | Benniella erionia | – | Bacteria provide fungus with increased resistance to Mn2+ concentrations | Lupini et al., 2022 |
Mycetohabitans rhizoxinica | Rhizopus microsporus | Vertically transmitted endobacteria with reduced genome containing few transcriptional regulators and quorum-sensing systems; and many transposons, virulence-related genes and putative effectors | Bacterium along with two Narnaviruses are needed for fungal sexual reproductive success. Bacterium produces a mycotoxin that allows fungus to be pathogenic | Partida-Martinez and Hertweck, 2005 |
Rhizobium radiobacter | Piriformspora indica | No reduced genome | Bacterium aids fungus in establishing plant–fungal symbioses | Guo et al., 2017 |
Ralstonia solanacearum | Ascomycota Mucoromycota Rhizoctonia solani | Produces lipopeptides to enter fungal cell walls of chlamydospores | – | Spraker et al., 2016 |
Bacterium . | Known fungal hosts . | Bacterial invasion characteristics . | Functional interaction . | References . |
---|---|---|---|---|
CaMg – Candidatus Moeniiplasma glomeromycotorum | AM Endogonales | Vertically transmitted endobacteria | – | Desirò et al., 2014; Lastovetsky et al., 2018 |
CaGg – Candidatus Glomeribacter gigasporarum | Gigasporaceae | Vertically transmitted endobacteria that expresses Type II, III and IV secretion systems and possesses vacB gene | Bacteria contain full operon for B12 vitamin synthesis | Ghignone et al., 2012; Lastovetsky et al., 2018 |
Burkholderia-related | AM | Vertically transmitted endobacteria | – | Lastovetsky et al., 2018 |
Mycoavidus sp. | Mortierella elongata | – | Bacterium relies on fungus for carbon and nitrogen | Li et al., 2017 |
Mollicute-related endobacteria | Benniella erionia | – | Bacteria provide fungus with increased resistance to Mn2+ concentrations | Lupini et al., 2022 |
Mycetohabitans rhizoxinica | Rhizopus microsporus | Vertically transmitted endobacteria with reduced genome containing few transcriptional regulators and quorum-sensing systems; and many transposons, virulence-related genes and putative effectors | Bacterium along with two Narnaviruses are needed for fungal sexual reproductive success. Bacterium produces a mycotoxin that allows fungus to be pathogenic | Partida-Martinez and Hertweck, 2005 |
Rhizobium radiobacter | Piriformspora indica | No reduced genome | Bacterium aids fungus in establishing plant–fungal symbioses | Guo et al., 2017 |
Ralstonia solanacearum | Ascomycota Mucoromycota Rhizoctonia solani | Produces lipopeptides to enter fungal cell walls of chlamydospores | – | Spraker et al., 2016 |
The diversity and functional significance of intimate BFIs is largely unknown. To date, three groups of endobacteria have been identified in AM fungi: CaGg (Candidatus Glomeribacter gigasporarum), which are rod-shaped Betaproteobacteria that inhabit members of the Gigasporaceae fungal family; CaMg (Candidatus Moeniiplasma glomeromycotorum), which are widespread coccoid-shaped Mollicutes-related endobacteria; and Burkholderia-related bacteria that appear phylogenetically similar to Mycoavidus cysteinexigens, an endobacterium of the soil fungus Mortierella elongata (Lastovetsky et al., 2018). Mycoavidus sp. has been shown to rely on M. elongata for carbon (C) and nitrogen (N), though it is unclear what the fungal host receives in return (Li et al., 2017). In a study coupling microfluidics with metabolomics, Burkholderia bacteria were shown to engage in metabolite exchange with M. elongata, obtaining organic acids from their fungal partners, and resulting in a higher growth rate for both organisms (Uehling et al., 2019). Similarly, the Burkholderia-related bacteria interacting with AM fungi may also rely on their hosts for nutrients.
There are several potential ways that the fungi may benefit from endobacteria. The few known functions of fungal endobacteria include the production of mycotoxins to confer fungal pathogenicity and promotion of fungal reproductive success (Table 1). Of the endobacteria that occur in mycorrhizal fungi, CaGg bacteria have the full operon for B12 vitamin synthesis (Ghignone et al., 2012), a potential currency for profiting from osmotically constant conditions and a supply of C compounds within their fungal hosts. CaGg and CaMg bacteria can coexist in the same spore (Desirò et al., 2014), which brings into question whether they provide fungi with two different benefits or whether they compete for fungal hosts.
The extent that BFI involving endobacteria in mycorrhizal fungi are necessary for downstream plant–fungal symbioses remains to be explored. Several of these endobacteria have reduced genomes containing host-colonization genes such as vacB (Ruiz-Lozano and Bonfante, 2000), which imply they have integrated closely within their fungal hosts and may have adapted to persist by promoting fungal fitness and consequently the fungi’s ability to form associations with plants. On the other hand, there are mycorrhizal endobacteria, such as Rhizobium radiobacter, which do not exhibit reduced genomes and appear to be facultative in their interactions with their host fungi. Rhizobium radiobacter has been reported to aid Piriformspora indica in establishing plant–fungal symbioses (Guo et al., 2017). The evolution of the mechanisms for these facultative bacterial symbionts is yet to be determined (Steffan et al., 2020) and understanding their functional significance in the context of plant–fungal interactions under different abiotic and biotic stresses will be important for future efforts to selectively increase the ecological fitness of mycorrhizal fungi. We now know that BFI have been occurring at least as long as plants have existed, and were central to plant evolution. These interactions are not always positive for the plant host. Predicting their function could partly be informed by studying the chemical signatures of these enigmatic systems.
Under the hood: the mechanics of plant–microbial perception
Although a strategy that benefits both plant and microbe has clear evolutionary advantages, it is by no means the only route available to microbes to gain plant-derived resources. In fact, the evolution of plant fungal pathogens and plant defences is rooted in the divergence of microbial trophic strategy and the co-evolutionary arms race (Anderson et al., 2010). Plant–microbe symbioses are ancient associations that facilitated early plant terrestrialization and have arisen multiple times through evolutionary history (Puginier et al., 2022). Plant recognition of symbionts shares molecular machinery with pathogenic responses. For example, symbiotic fungal colonization of Medicago truncatula showed that the plant has similar pre-infection nuclear repositioning responses to both the AM symbiont and fungal pathogens; these responses involve dmi3 which encodes a calcium/camodulin-dependent protein kinase (Genre et al., 2009). This finding indicates common primary responses occur in plants to interacting microbes, and are further modified depending on the nature of the interacting organism. All plant–microbial interactions are dependent on mutual recognition through effectors (e.g. fungal polysaccharides, microbe-associated molecular patterns, chitin) or via plant/hyphal exudates (Fig. 1, panel 3). Importantly, the composition of plant-exuded chemicals can be altered during plant–microbe interactions. For instance, plant volatiles are exuded very early on in the interaction between M. truncatula and Rhizophagus irregularis and contain large amounts of limonene (Dreher et al., 2019). In contrast, when infected with the pathogenic oomycete Aphanomyces euteiches, exudation of sesquiterpene predominated. Thus, exudates are highly dependent on the specific microbe present. Although the exact function of many different exuded chemical remains to be established, changing chemical ‘flavours’ is a strategy by the plant to distinguish ‘friend’ from ‘foe’. During early symbiosis, and interaction with a pathogen, the common symbiotic pathway (CSP) is activated. However, the mechanisms of interaction between plant and specific microbes are nuanced, and similarities usually hold at the top levels. For instance, in rhizobia, activation of nitrous oxide precedes transient reactive oxygen species (ROS) accumulation, activation of the auxin pathway and the suppression of pathogen-elicited phytohormone responses (such as ethylene, salicylic acid and jasmonic acid), resulting in sustained ROS production and plant defence responses (Anderson et al., 2010). The fact that nodulation (NOD) factors in rhizobia can also elicit a defence response in non-hosts (Liang et al., 2013) suggests effector and NOD factor recognition share a similar pathway with pathogen response in plants, despite their potentially beneficial function. Most endophytic and necrotrophic lifestyles are not evolutionarily stable traits, with evidence for multiple ‘switches’ between these modes within a single lineage of origin (Delaye et al., 2013). It is perhaps this shifting of trophic strategies in interacting microbes over large evolutionary timescales that has led to the development of varied and elaborate plant response mechanisms. Biotrophy (whereby pathogens can benefit nutritionally from living host cells) seems to emerge and persist, indicating it is a much more stable strategy. Although they have multiple and separate origins, biotrophs, necrotrophs and endosymbionts all share commonalities, such as host immune circumvention (Andrew et al., 2013).
The development of fungal effectors probably first appeared to ‘mask’ invading pathogens from the plant host to prevent a defence response (e.g. the Pleidies effectors in the maize pathogen Ustilago maydis; Navarette et al., 2021). The nutritional benefit to the microbe through access to plant resources could be curtailed when the host plant developed additional recognition and protection strategies (mechanical impedance, chemical disarmament) and when the hyper-sensitive response (HR) evolved to effectively kill the infected cell and pathogen. Development of cytotoxicity and self-defence within pathogens will have then provided a benefit for a necrotrophic organism, where cell destruction allows access to resources (indeed, many necrotrophic effectors have evolved specifically to induce HR and cell death; Shao et al., 2021). During a necrotrophic challenge, the plant immune system requires different strategies to defend its tissue; for instance, camalexin production in Arabidopsis thaliana is an effective defence against many necrotrophs (Khare et al., 2017). Pathogen resistance to host defence, for instance via metabolic degradation of these antimicrobial molecules, is a key facet of the ongoing adaptation and survival of these pathogens (Newman and Derbyshire, 2020). Hence, effector-led infection/defence is locked in an evolutionary cycle, where development of novel effectors leads to development of novel defences: a cat-and-mouse between pathogens masking their existence and plant detection of their presence. The occasional emergence of hemi-biotrophy, where pathogens can ‘switch’ their trophic modes, may occur to gain the upper hand and most efficient nutrient acquisition, as suggested is the case for the fungal hemi-biotroph Sclerotinia sclerotiorum (Kabbage et al., 2015). These multifaceted evolutionary events have generated the complexity we currently observe in defence-related plant metabolism, and an immune machinery that must balance interaction with multiple symbiotic/pathogenic microbes of differing origins.
The similarities between initiation of microbial symbioses and immune suppression strategies are remarkable. However, most molecular interactions are highly specialized (i.e. specific host–microbe machinery), presenting difficulty in unpicking the fundamental aspects of early symbioses. In these early scenarios, as well as in contemporary ecologies, microbes would not have interacted with plants in isolation but instead in complex, trophically competitive situations. Niche exploitation and specialization are likely to have appeared rapidly where many competing microbes are vying for an edge over access to plant-derived resources. In certain circumstances partnerships between two or more microbes would have provided more successful niche exploitation. For example, it has been suggested that Oxalobacteraceae species, which are found living abundantly within AM hyphae, provide the fungi with greater colonization ability, probably through production of unique metabolites (Scheublin et al., 2010). In contrast, pathogens can also utilize symbioses to help infect hosts. Strains of the plant beneficial bacteria Pseudomonas fluorescens appear to be susceptible to colonization by pathogens such as Escherichia coli and Staphylococcus aureus (Zarei et al., 2022). Considering certain opportunistic pathogenic bacteria (e.g. S. aureus) have the ability to hijack the mammalian immune response via inoculation of avirulent bacteria (Boldock et al., 2018), it is not unreasonable to suggest a similar hijacking approach can also occur in plants. Likewise, when multiple beneficial organisms interact with the same host, selective niche specialization allows a competitive edge to the individual but where niche overlap occurs, nutritional cross-feeding has been shown to promote coexistence (Jacoby and Kopriva 2019). For example, in nodulating plants, such as clover, both the plant and rhizobia stand to gain a nutritional advantage when a third-party AM symbiosis also forms (Ossler et al., 2015). These benefits are dependent on the developmental stage of the symbiosis, where initial growth penalties are succeeded by much greater benefits when the tripartite interaction is fully established (Mortimer et al., 2008). However, specific host genetics and abiotic environments will also determine the extent of collegiality between these symbiotic partners (Ossler et al., 2015). During their evolution, plant-associated microbes have diversified to exploit a range of interaction strategies. While complex, the outcome tends to be positive or negative for the plant host. It is due to this fact that plant recognition mechanisms contain so much overlap and redundancy, albeit characterizing the extent to which these mechanisms differ among plant groups, and with specific interacting microbes, is an ongoing task.
Microbial consortia are the functional workforce of the rhizosphere
Understanding the roles of individual rhizosphere microbes within an ecological consortium is much more difficult than for one or two competing root-colonizers. The rhizosphere, much like the gut, is a melting pot of functionally active microbes, which can act to alter host physiology in various ways (Selosse et al., 2014). Modern cataloguing approaches (meta-genomics) can identify the microbe species present, or the types of functional genes that exist in the system, but cannot directly infer function (Hornung et al., 2019). This is a particular problem considering that many species with assigned ‘functions’ (e.g. pathogen, symbiont, saprotroph, wood-decayer) are only studied in certain contexts, and their functional adaptability is not recorded. For example, Pseudomonas simiae generally acts as a beneficial and growth-promoting bacterium in A. thaliana; yet when CO2 is elevated and nutrient availability is low, A. thaliana root development becomes repressed under P. simiae colonization (Williams et al., 2018). Considering these points, the traditional reductionist experimental systems we use to study interaction (i.e. 1 plant, 1 microbe, 1 set of conditions) could be inadequate to describe the biological function of the organisms in question (see Spanu and Panstruga, 2017). Considering entire microbial assemblages of a system, such as a rhizosphere, as a tool to predict functional traits is equally problematic, largely due to the complexity of these interactions (Munoz-Ucros et al., 2021). More recent use of synthetic communities coupled with high-resolution -omics technologies permits unprecedented insight into the molecular foundations of niche partitioning across different species. This approach was recently taken in A. thaliana (Mataigne et al., 2022) where use of genome-scale metabolic models indicated that metabolic asymmetry of the rhizosphere (driven by nutrient availability and root exudation) predetermines microbial niche partitioning and overlap.
Most microorganisms operate as part of larger multi-species consortia, including within specialized multicellular complexes such as biofilms (Yang et al., 2011). These communities cohabit a specialized slurry consisting of extracellular polymeric substances (EPS). They are present throughout nature in most conceivable habitats (such as water pipes, bones and prosthetics after trauma or surgery; Bar-On and Milo 2019), anywhere a suitable surface and microbes are present. Pertinently, biofilms develop within the rhizosphere, particularly along the root surface (Fig. 1, panel 5). The biochemistry of biofilms is seldom described, principally due to their mechanical sensitivity and the difficulty extracting a root system from soil without interrupting them. It has been demonstrated that Bacillus subtilis biofilms on plant roots are reliant on plant-derived polysaccharides that are used as both a cue and an energy source to produce the EPS of the biofilm (Beauregard et al., 2013). Biofilms are chemically rich environments and, along with the mucilage of the roots, may coexist as an interface between plant and microbes for both communication and nutrient exchange via exuded metabolites. Further, it has been postulated that the evolution and exudation of C-rich metabolites that constitute the mucilage act as a de facto phytogenic scaffold, not dissimilar in properties to the EPS, which rhizosphere microbes can make use of to rapidly develop their biofilms (Nazari et al., 2022). Microbe to microbe communication occurs within biofilm environments too, and it is likely that mutually beneficial interactions occur here such as between AMs and PGPR bacteria, such as P. simiae (Pandit et al., 2020). Biofilms also act as a potentially attractive cache of C-rich resources for invading organisms. Fascinatingly, these rich communities exhibit elements of self-preservation, as well as providing a genetic reservoir with high potential for adaptation, for example through horizontal gene transfer between bacteria (Lyons and Kolter, 2015; Berthold et al., 2016). It is remarkable then that their study is still so limited, and there is much that their formation, function and metabolism can tell us about early life and microbial [bacteria–fungi (B–F), bacteria–bacteria (B–B), fungi–fungi (F–F)] interactions.
Bacterial trafficking via hyphal highways
In addition to harbouring genetic and chemical diversity, microbial biofilms act as a medium through which microbes can move within the rhizosphere. In soil, bacteria are heterogeneously dispersed and are mobile through swimming, swarming, twitching, sliding or gliding, all of which are more efficient in the presence of a liquid (Henrichsen, 1972; Dechesne et al., 2010). Although pores filled with water are present between soil particles, the rugged landscape of substrates can mean bacterial movement is limited, even in the presence of chemotaxis (Zhang et al., 2020). In fungal networks, the thin water film which surrounds hyphae is used like a ‘highway’, allowing particular bacteria to translocate along it with ease (Jansa and Hodge, 2021). For example, the saprotrophic fungus Lyophyllum sp. carries bacteria, which travel distances as great as 1 cm per day (Warmink and Van Elsas, 2009). Bacterial movement via hyphae has also been demonstrated in vitro for AM fungi and the phosphorus-mineralizing bacterium Rahnella aquatilis (Jiang et al., 2021). These authors present a model for the recruitment and transport of bacteria by mycorrhizal fungi lacking the genetic machinery to mineralize nutrients in complex organic material. The implications of this model for the function of BFIs have real ecological significance, as they amplify the influence of fungi in the rhizosphere; that is, they not only shape the composition of the microbial community around them, but also the spatial distribution of bacteria. It is likely that there is some level of selectivity to the types of bacteria that are translocated on the hyphae, for example through size exclusion, the release of deterrent or attractant compounds, and through microbe–microbe communication.
Microbial hub-bub: the molecular language of the rhizosphere
Microbes possess an innate ability to secrete and recognize different chemical cues in their environment. These semiochemicals are either ‘self’ specific, an example being small-peptide pheromonic signals (Calcagnile et al., 2019), or shared between different species, examples including allelochemicals such as coumarins, flavonoids and organic acids. Self and neighbour recognition allows microbes to form mixed communities as well as secure nutrient resources by limiting competition, for instance production of siderophores with both iron-sequestering and growth-inhibitory functions (Gu et al., 2020). While self-recognition cues are more straightforward to identify in controlled conditions, it is only recently that the vast suite of secondary metabolite signals microbes use have started to be unravelled in mixed communities (Chevrette et al., 2022). This is largely due to development of high-throughput, high-resolution mass spectrometry and imaging techniques such as DESI-ESI and MS-MS networking (Stasulli and Shank, 2016). Similarly, monocultured microbes seldom produce signals in the absence of other microorganisms (Liu and Kakeya, 2020) limiting the knowledge we can garner from traditional culturing approaches. More intricate, tri- or multi-partite interactions can provide us with much more relevant information, for instance the components and activity of microbial allelochemical exudation. A captivating illustration of competitive allelopathy can be found in the fungal endophyte of Japanese plum-yew, Paraconiothyrium variabile, which can negatively modulate the production of the mycotoxin beauvericin by phytopathogenic Fusarium oxysporum; this occurs through elevated secretion of P. variabile secondary metabolites including 13-oco-9, 11-octadecdienoic acid (Combès et al., 2012). In this example resource guarding by the endophyte is tailored towards host protection – if the host is damaged C allocation to the endophyte is reduced.
The examples above assume cell-to-cell communication between individual microbial species, but if considering a microbial consortium, it is logical to discuss whole community chemical communication. Quorum sensing (QS) is the process by which exuded signals can synchronize a microbial community response to a trigger, a classic example being population density (Darch et al., 2012). QS relies on autoinducers that are produced and recognized between cells. This phenomenon is generally studied within species (e.g. B. subtilis, which utilize a multi-protein complex, ComQXPA, to achieve QS; Kalamara et al., 2018), but sensing and response between species also occurs (Whitehead et al., 2001), for instance in a biofilm where the production of both granular structures and EPS are determined by the QS metabolite N-acyl-homoserine-lactone (AHL; Tan et al., 2014). Although multi-species QS mechanisms are less well understood, combined modelling and genetic cataloguing approaches suggest that both long-range and species-specific signals can move in this way (Silva et al., 2017; Abisado et al., 2018). More recently the use of similar modelling techniques and knowledge of AHL-directed QS have been postulated as a method to engineer QS-like behaviours across industrially valuable microbes, such as biofuel-producing cyanobacteria (Kokarakis et al., 2023). Although these synchronizing behaviours are incredibly useful in the rhizosphere context, a dearth of technology directed toward their study means this is still an emerging field of research. QS certainly has a role in many microbes, including rhizobia (for nodulation/N fixation; Gosai et al., 2020), PGPR (Hartmann, 2020), and plant-growth-promoting fungi (rhizobia within AM spores; Palla et al., 2018). Importantly, plant hosts have evolved both to recognize and to respond to some of these QS signals, allowing them a greater selective control over microbial partners (such as QS quenching to disrupt activity of various pathogens; Rodríguez et al., 2020), highlighting the complexity of chemical communication among mixed plant-interacting microbial communities in the rhizosphere. Hence, the interface through which BFI and plant communication takes place is important for recognition and function. It is by identifying and characterizing this chemical language that we can start to develop a toolbox for a much more in-depth understanding of rhizosphere communication at large.
Mycorrhiza helper bacteria: the fungal facilitators
Mycorrhiza helper bacteria (MHB) are the suite of microbes associated with mycorrhizal fungi and which serve as the third crucial participant in plant–BFI associations (Sangwan and Prasanna, 2022). MHB actively contribute to, and promote, various aspects within the mycorrhizal symbiosis including the functioning of AM symbiosis, stimulation of hyphal growth and spore germination, root colonization, and the improvement of metabolic fitness of AM fungi. MHB are not specifically associated with a particular type of mycorrhizal symbiosis, or taxonomic classification of bacterial strain. MHB were first discovered when Douglas fir was co-inoculated with the EM fungus Laccaria laccata and P. fluorescens BBc6 (Duponnois and Garbaye, 1991). Since their discovery, MHB have been classified into two distinct groups based on their mode of action: mycorrhiza helper bacteria influence the functioning of an existing AM fungal symbiosis; and mycorrhization helper bacteria actively stimulate the formation of the symbiotic association between AM fungi and the roots of their host plants (Frey-Klett et al., 2007). MHB have been isolated from diverse environments: the mycorrhizosphere, hyphosphere, sporocarps and fruiting bodies of EM fungi.
To date, many bacterial strains have been reported to be able to promote either AM or EM symbioses (Table 2; Garbaye, 1994; Barea et al., 2002; Johansson et al., 2004; Artursson et al., 2006; Duponnois, 2006) with the first MHB being described in association with the AM fungus Rhizophagus (Glomus; Mosse, 1962). Being inhabitants of the mycorrhizosphere, MHB are not governed directly by the plant host, but they exhibit a gradient of specificity in relation to the fungus (Hameeda et al., 2007). However, it is important to note that the composition and function of the bacterial community can vary depending on the plant species and prevailing environmental conditions. The MHB strains that have been identified to date belong to many bacterial groups and genera (Table 2), such as gram-negative Proteobacteria, gram-positive Firmicutes and gram-positive Actinomycetes. The co-evolutionary history between MHB and their fungal partners has probably influenced the specificity of their interactions, enabling finely tuned cooperation in nutrient acquisition and other essential functions. However, there are additional groups of MHB that exhibit more generalized effects on plant growth promotion, highlighted in numerous studies (Deveau and Labbe, 2017). The effects encompass the production of phytohormones, such as auxins, cytokinins and gibberellins, as well as play pivotal roles in regulating various aspects of plant growth, including root development, shoot growth and flowering. Therefore, beneficial activities of MHB are not solely limited to their role in mycorrhizal formation and function.
Bacteria known to be involved in bacterial–fungal interactions (BFIs) on the exterior of fungal structures.
Bacterium . | Known fungal hosts . | Functional interaction . | References . |
---|---|---|---|
Paenibacillus validus | Glomus intraradices | Fungus grows and sporulates when in co-culture with bacterium. Fungus uses raffinose as a carbon source | Hildebrandt et al., 2002, 2006 |
Pseudomonas putida | Glomus fistulosum | Fungal colonization of roots and hyphal growth in substrate is higher when bacterium is present | Vosátka and Gryndler, 1999 |
Paenibacillus sp. strain B2 | Funneliformis mosseae | Bacterium stimulates colonization of sorghum roots by the fungi. Bacterium suppresses soil-borne pathogens | Budi et al., 1999 |
Bradyrhizobium japonicum | Bacterium produces nodulation (Nod) factors that stimulate production of flavonoids by soybean and subsequently promote mycorrhiza formation | Xie et al., 1995 | |
Bacillus pabuli | Glomus clarum | Bacterium enhances fungal spore germination and fungal colonization of pea plants. Bacterium also enhances shoot growth, shoot N content and P use efficiency in plants | Xavier and Germida, 2003 |
Pseudomonad sp. | Rhizopogon luteolus | Bacterium promotes establishment of mycorrhiza with Pinus radiata | Garbaye, 1994 |
Streptomyces sp. | Glomus mosseae | Bacterium promotes fungal spore germination | Tylka et al., 1991 |
Bacterial sp. | Glomus mosseae | Bacterium reduces heavy metal damage to hyphae and increases mycelial growth and mycorrhiza formation | Vivas et al., 2005 |
Bacterium . | Known fungal hosts . | Functional interaction . | References . |
---|---|---|---|
Paenibacillus validus | Glomus intraradices | Fungus grows and sporulates when in co-culture with bacterium. Fungus uses raffinose as a carbon source | Hildebrandt et al., 2002, 2006 |
Pseudomonas putida | Glomus fistulosum | Fungal colonization of roots and hyphal growth in substrate is higher when bacterium is present | Vosátka and Gryndler, 1999 |
Paenibacillus sp. strain B2 | Funneliformis mosseae | Bacterium stimulates colonization of sorghum roots by the fungi. Bacterium suppresses soil-borne pathogens | Budi et al., 1999 |
Bradyrhizobium japonicum | Bacterium produces nodulation (Nod) factors that stimulate production of flavonoids by soybean and subsequently promote mycorrhiza formation | Xie et al., 1995 | |
Bacillus pabuli | Glomus clarum | Bacterium enhances fungal spore germination and fungal colonization of pea plants. Bacterium also enhances shoot growth, shoot N content and P use efficiency in plants | Xavier and Germida, 2003 |
Pseudomonad sp. | Rhizopogon luteolus | Bacterium promotes establishment of mycorrhiza with Pinus radiata | Garbaye, 1994 |
Streptomyces sp. | Glomus mosseae | Bacterium promotes fungal spore germination | Tylka et al., 1991 |
Bacterial sp. | Glomus mosseae | Bacterium reduces heavy metal damage to hyphae and increases mycelial growth and mycorrhiza formation | Vivas et al., 2005 |
Bacteria known to be involved in bacterial–fungal interactions (BFIs) on the exterior of fungal structures.
Bacterium . | Known fungal hosts . | Functional interaction . | References . |
---|---|---|---|
Paenibacillus validus | Glomus intraradices | Fungus grows and sporulates when in co-culture with bacterium. Fungus uses raffinose as a carbon source | Hildebrandt et al., 2002, 2006 |
Pseudomonas putida | Glomus fistulosum | Fungal colonization of roots and hyphal growth in substrate is higher when bacterium is present | Vosátka and Gryndler, 1999 |
Paenibacillus sp. strain B2 | Funneliformis mosseae | Bacterium stimulates colonization of sorghum roots by the fungi. Bacterium suppresses soil-borne pathogens | Budi et al., 1999 |
Bradyrhizobium japonicum | Bacterium produces nodulation (Nod) factors that stimulate production of flavonoids by soybean and subsequently promote mycorrhiza formation | Xie et al., 1995 | |
Bacillus pabuli | Glomus clarum | Bacterium enhances fungal spore germination and fungal colonization of pea plants. Bacterium also enhances shoot growth, shoot N content and P use efficiency in plants | Xavier and Germida, 2003 |
Pseudomonad sp. | Rhizopogon luteolus | Bacterium promotes establishment of mycorrhiza with Pinus radiata | Garbaye, 1994 |
Streptomyces sp. | Glomus mosseae | Bacterium promotes fungal spore germination | Tylka et al., 1991 |
Bacterial sp. | Glomus mosseae | Bacterium reduces heavy metal damage to hyphae and increases mycelial growth and mycorrhiza formation | Vivas et al., 2005 |
Bacterium . | Known fungal hosts . | Functional interaction . | References . |
---|---|---|---|
Paenibacillus validus | Glomus intraradices | Fungus grows and sporulates when in co-culture with bacterium. Fungus uses raffinose as a carbon source | Hildebrandt et al., 2002, 2006 |
Pseudomonas putida | Glomus fistulosum | Fungal colonization of roots and hyphal growth in substrate is higher when bacterium is present | Vosátka and Gryndler, 1999 |
Paenibacillus sp. strain B2 | Funneliformis mosseae | Bacterium stimulates colonization of sorghum roots by the fungi. Bacterium suppresses soil-borne pathogens | Budi et al., 1999 |
Bradyrhizobium japonicum | Bacterium produces nodulation (Nod) factors that stimulate production of flavonoids by soybean and subsequently promote mycorrhiza formation | Xie et al., 1995 | |
Bacillus pabuli | Glomus clarum | Bacterium enhances fungal spore germination and fungal colonization of pea plants. Bacterium also enhances shoot growth, shoot N content and P use efficiency in plants | Xavier and Germida, 2003 |
Pseudomonad sp. | Rhizopogon luteolus | Bacterium promotes establishment of mycorrhiza with Pinus radiata | Garbaye, 1994 |
Streptomyces sp. | Glomus mosseae | Bacterium promotes fungal spore germination | Tylka et al., 1991 |
Bacterial sp. | Glomus mosseae | Bacterium reduces heavy metal damage to hyphae and increases mycelial growth and mycorrhiza formation | Vivas et al., 2005 |
MHB: engineers of mycorrhizal development and function
MHB have been reported to facilitate spore germination and promote mycelial growth in mycorrhizal fungi. The stimulatory effect of MHB and their culture filtrates on spore germination was first documented in the endomycorrhizal fungi, Glomus mossae (Mosse, 1962). Subsequently, the effects of MHB associated with Glomus clarum, where direct contact between the spores and bacteria was necessary for the induction of spores, were observed (Xavier and Germida, 2003), indicating a ligand–receptor interaction between the two microbes. However, the interaction was suggested to be much more complex as these spore germination stimulatory bacteria were accompanied by other bacterial isolates on the G. clarum spore surface, producing antagonistic volatiles, which regulated spore germination. In contrast, volatile compounds produced by different species of Streptomyces were shown to promote the germination of G. mossae spores (Tylka et al., 1991). Similarly, it was shown that the otherwise obligately symbiotic Glomus intraradices could grow and sporulate without a plant in fungal–bacterium co-cultures with Paenibacillus validus (Hildebrandt et al., 2002), with raffinose being detected as the specific C source in the cultures supporting mycelial growth (Hildebrandt et al., 2006). An increase in mycelial biomass and promotion of mycorrhiza establishment has also been shown in the presence of MHB (Garbaye and Bowen, 1989; Gryndler and Vosátka, 1996; Founoune et al., 2002). The colonization of roots with Glomus fistulosum and the growth rate of the hyphae in the soil substrate were significantly higher when the fungus was co-inoculated with Pseudomonas putida or with the low-molecular-weight fraction of the bacterial culture supernatant (Vosátka and Gryndler, 1999), indicating that the effective substances were in this fraction. MHB may contribute to AM fungal hyphal growth through their anti-pathogenic efficacy (Lioussanne et al., 2006). The MHB Paenibacillus sp. strain B2 was found to stimulate colonization of Funneliformis mossae in sorghum, while simultaneously suppressing soil-borne pathogens such as Phythophora parasitica, Fusarium oxysporum and Rhizoctonia solani (Budi et al., 1999). MHB have also been implicated in having a strong positive impact on spore germination and on presymbiotic fungal growth under toxic concentrations of heavy metals; bacterial inoculation not only reduced damage to G. mossae hyphae but even resulted in increased mycelial growth and mycorrhiza formation (Vivas et al., 2005). MHB may also play a vital role in promoting the proliferation of plant root systems. Morphological changes in roots during mycorrhiza formation have been attributed to phytohormones, including auxins and ethylene (Kaska et al., 1999; Horii and Ishii, 2006). These include the formation of lateral roots and dichotomous branching of short roots (Barker and Tagu, 2000), which leads to an increase in potential points at which plant and fungus can interact. MHB have also been indirectly implicated in facilitating root colonization and directing mycelial growth of both AM and EM fungi towards fine roots through the release of chemotropic signals such as flavonoids from plants (Lagrange et al., 2001; Akiyama et al., 2002). Xie et al. (1995) demonstrated that the NOD factors produced by an MHB Bradyrhizobium japonicum strain stimulated the production of flavonoids by soybean (Glycine max) seedlings and subsequently promoted mycorrhiza formation.
MHB interacting with AM fungi also have the ability to enhance the functionality of already established mycorrhizal symbioses, by contributing to mineral weathering and their ability to solubilize phosphorus (Viveganandan and Jauhri, 2000). Within the soil and rhizosphere, various microbes, including fungi and bacteria, actively participate in mineral weathering by secreting protons and complexing agents such as low-molecular-weight organic anions or siderophores. The collective influence of the entire mycorrhizal complex, comprising the root, the symbiotic fungus and other associated microorganisms, on mineral weathering ultimately leads to better plant growth (Hameeda et al., 2007). In an onion crop, the MHB strains Enterobacter sp. and B. subtilis expedited the uptake of phosphorus from rock phosphate when inoculated with R. irregularis (Toro et al., 1997). This characteristic of MHB was also demonstrated by Jayasinghearachchi and Seneviratne (2005), who found that mixed biofilms of phosphate-solubilizing fungi and Bradyrhizobium elkanii accelerated the process of rock phosphate solubilization. Hence, MHB probably play a central functional role within BFI and plant interactions that we are only just beginning to uncover.
A MYCORRHIZAL PARADIGM SHIFT: ABUNDANT, UNDEREXPLORED AND ECOLOGICALLY RELEVANT SYMBIOTIC RELATIONSHIPS
The unfolding awareness of emerging groups of mycorrhizal fungi has had major ramifications for terrestrial mycorrhizal research (Bidartondo et al., 2011; Field et al., 2015, 2016, 2019; Field and Pressel, 2018; Hoysted et al., 2019, 2023). Mucoromycotina fine root endophyte (MFRE) fungi are a facultatively biotrophic and saprotrophic lineage (Fig. 1, panels 2–5; Bidartondo et al., 2011; Field et al., 2015, 2016), opening up a realm of undiscovered ecosystem functions alongside the strictly biotrophic Glomeromycota AM fungi, with which MFRE fungi were classified together until recently (Stürmer, 2012, Orchard et al., 2017a). Exclusive plant–MFRE symbioses seem to be rare, with the majority of land plants forming simultaneous associations alongside AM fungi (Rimington et al., 2015), with recent research highlighting a potential functional complementarity between the two groups (Field et al., 2019). Traditionally, MFRE fungi have been functionally associated with the facilitation of plant P uptake and occur commonly in soils with low P availability (Smith et al., 2015; Albornoz et al., 2016; Orchard et al., 2017b). However, recent exploration of MFRE function in wild-collected plant-based systems showed that MFRE symbionts transferred significantly more 15N tracer compared to 33P tracer to an early-diverging vascular plant than to Haplomitropsida liverworts in comparable experiments (Field et al., 2016; Hoysted et al., 2019). Furthermore, using analysis of natural abundance 15N signatures, it was shown that MFRE-associated Lycopodiella inundata and Juncus bulbosus were 15N enriched compared to co-occurring reference plants with different mycorrhizal partners, supporting a hypothesis that plants hosting MFRE symbionts benefit from fungally acquired N (Hoysted et al., 2019). While it has been shown that some Glomeromycota AM fungi can transfer N to their associated host (Ames et al., 1983; Hodge et al., 2001, Leigh et al., 2009), the ecological relevance has been widely debated (Smith and Smith, 2011). Coupling this with emerging evidence that MFRE fungi may transfer more N to host liverworts from organic sources compared to Glomeromycota AM fungi (Field et al., 2019) further highlights their saprotrophic capabilities. Nonetheless, it is crucial to highlight that the majority of MFRE research has been carried out using unpasteurized soil culture-based experimental systems (Orchard et al., 2017b; Albornoz et al., 2020) or wild-collected plants (Field et al., 2015, 2016, 2019; Hoysted et al., 2019, 2021a, b), and so probably included other soil microorganisms such as bacteria, alongside MFRE fungi. Recently, an experimental system has been developed to overcome this challenge and can test the function of MFRE fungi in the absence of other microorganisms (Hoysted et al., 2023).
Using a novel and tractable in vitro experimental system designed to manipulate axenic MFRE cultures (Hoysted et al., 2023) we can begin to address fundamental questions regarding the mycorrhizosphere and its involvement in MFRE lifestyles (i.e. facultative biotrophy and/or saprotrophy and function). These fundamental questions give rise to exciting possibilities and questions particularly regarding the different ecosystems in which we find diverse lineages of mycorrhizal fungi and their associated bacteria. Microbe complementarity compared to associations with only one type of fungus may be hugely beneficial for the host plant (Field et al., 2019) as there may be differences in the ability of plants to utilize soil nutrients when associating with different groups of biotrophic and/or saprotrophic fungi, and/or their bacterial associates. With several environmental factors potentially impacting the activity of saprotrophic microbes (both bacteria and fungi), including temperature, pH, substrate additions and soil moisture (Rousk and Bååth, 2011), it is imperative to study such tripartite interactions further including fungus, B-B interactions and BFIs.
Perspectives on plant–bacterial–fungal legacies
The relationship between mycorrhizal fungi and the mycorrhizosphere carries significant ecological implications. Despite plants and mycorrhizal fungi interacting for >450 million years, the functional significance of mycorrhizosphere and hyphosphere bacterial partners are only starting to be appreciated. Such BFIs play pivotal roles in shaping the functioning of ecosystems and influencing plant community dynamics. The soil bacterial community can adversely affect mycorrhizal fungal activities in soil by competing for different nutrient sources, reducing plant growth (i.e. pathogens), interacting with other soil microbes and producing unfavourable chemicals (Nehl et al., 1997; Glick, 2005). However, soil microbial communities can also exert positive effects on mycorrhizal fungi through: the production of plant hormones, solubilization of soil nutrients, improvement of soil structure, control of plant pathogens and stimulation of plant growth (Glick, 2005; Rillig and Mummey, 2006).
Among the most important implications of microbial interactions in ecosystem conservation is the alleviation of different soil stresses including salinity, drought, acidity, compaction and heavy metals. Microbes are constantly interacting in the soil, and therefore an understanding of how mycorrhizal fungi and other soil bacteria can alleviate the unfavourable effects of heavy metals on plant growth or how the specific manipulation and engineering of microbes can be used for the remediation of polluted soils (Berg, 2009; Joner and Leyval, 2009) is highly desirable. AM fungi are able to alleviate stress by enhancing plant growth, storing heavy metals in the vacuoles of their vesicles and binding them by the production of the glomalin complex (Khan, 2005; Holátko et al., 2021). Byproducts of glomalin interactions are able to bind soil particles and form soil aggregates, resulting in improved soil structure (Andrade et al., 1998; Barea et al., 2005). Consequently, the advantages of engineering the mycorrhizosphere with a view to enhancing soil structural properties and fertility are attractive. Further research is needed to investigate how stress tolerance can be improved when using AM fungi in combination with other soil microbes, including new emerging groups of mycorrhizal fungi, such as MFRE fungi (Bidartondo et al., 2011; Field and Pressel, 2018; Hoysted et al., 2023).
Engineering of the mycorrhizosphere with respect to enhancing crop plant growth and yield without the use of chemical fertilizers has been highlighted in the literature (Giovanni et al., 2020; Santos and Olivares, 2021). However, BFIs are highly complex and include a wide range of mechanisms where diverse molecules are involved. In agriculture, BFIs are often explored in the frame of the development of biocontrol strains, yet the response, often carried out in glasshouses, is dependent on many factors including the species of each organism involved in the tripartite interaction as well as the prevailing environmental conditions. While AM fungi have the potential to contribute to cereal nutrient assimilation (Thirkell et al., 2019), limitations surrounding the need for high colonization and a diverse fungal community to maximize crop yield, and failure to apply rigorous agronomic methodology, have been raised (Ryan and Graham, 2018). Such caveats include the small number of agricultural field studies and the ability to manage AM fungi likely to be compromised by rudimentary knowledge underlying mycorrhizal symbiosis. Therefore, there is a call for further field-scale experimentation of what role AM fungi may be playing in crop growth (Rillig et al., 2019; Thirkell et al., 2019). This is further complicated by the complexity of interactions between mycorrhizal fungi and other soil microbes including bacterial communities, which we have extensively highlighted in this review. Across 11 host species from three different habitats, AM fungi were reported to benefit most plants similarly, but the response of the plant differed when soil filtrates from habitats in which they did not occur were applied (Pizano et al., 2017). This implies that certain environments accumulate species-specific soil pathogens, whereas other habitats accumulate soil microbes that support native plant species (Pizano et al., 2017). Further, a meta-analysis, incorporating datasets from both laboratory and field studies, found that the beneficial impact of AM fungi on grain yields was less pronounced in ‘field-inoculation’ conditions, displaying an overall effect of 16 %. The findings exhibited variability, crop-specificity, were lower for new cultivars introduced after 1950 and were additionally influenced by soil pH (Zhang et al., 2018). Currently, the application of bacterial–fungal bioinoculants to agricultural systems is limited by a variety of factors, including unculturability, low survival rate and low colonization ability of introduced concoctions, as well as lowered expression of key antagonistic traits under field conditions. Nevertheless, their full potential will probably be captured during future work, with the use of comprehensive multi-omics approaches. Therefore, understanding of detailed dynamic inter-kingdom relationships, whether synergistic or antagonistic, will allow scientific exploration and, potentially, agricultural exploitation of these BFIs.
Within the soil microbiome, BFIs play a crucial role in ecosystem functioning and plant health. Here, we emphasize the complexity of BFIs, which can vary depending on plant species, mycorrhizal type and environmental conditions. Overall, we call for critical insights into the modulation of microbiome assembly of emerging groups of mycorrhizal fungi. Filling knowledge gaps and exploring the functional details of microbial interactions in the soil microbiome will advance our holistic interpretation of the true complexity of tripartite interactions in natural and agricultural settings. Using cytological, molecular, proteomic and metabolomic comparisons between inoculated host plants (singly or co-colonized, with both MFRE and AM fungi, in addition to mycorrhizosphere bacteria) we can begin to tease out the finer functional details, in terms of nutrient transfer. Furthermore, investigating how mycorrhizosphere microbial communities accelerate mineralization of essential nutrients will provide invaluable insight into their ecological role within plant–fungal life-systems.
Continued research into the functional capacity of soil microbes and the comparison of sterile and non-sterile experimental systems will contribute to our understanding of the complex interplay between plants, fungi and bacteria in the soil ecosystem. Recognizing the glaring caveats discussed above, this research may also provide opportunities for translation and exploitation into vulnerable agricultural systems. The ecological significance of plant–fungal–bacterial interactions highlights the potential for improving synergistic interactions between mycorrhizal fungi and soil microbes to contribute towards a sustainable future, and therefore we suggest increased efforts to disentangle positive, negative or neutral outcomes in tri-/multi-partite interactions in both laboratory and field settings. The use of soil microbes, including MFRE fungi, stands as a potential solution for alleviating soil stresses and improving soil structure and fertility, such as with AM fungi. Fundamentally, soil biodiversity plays a critical role, particularly interactions within the soil microbiome, in sustaining life on Earth. The vulnerability of soil biodiversity to climate change underlines the urgency of maintaining and improving soil quality for the health of managed and natural ecosystems.
FUNDING STATEMENT
The authors received no financial support for the preparation, authorship and/or publication of this article.
CONFLICT OF INTEREST
The authors report no known conflicts of interest that impact the validity of this work.
ACKNOWLEDGEMENTS
We thank Dr Zoë Popper for inviting G.A.H. to contribute the present review to Annals of Botany. We thank Dr Nicholas Brereton and the anonymous reviewers for their constructive comments that helped to develop our manuscript. A.W. and B.S. were supported by an ERC CoG “MYCOREV” (865225). We also thank Research Figures, Ireland, for the scientific illustration.
AUTHOR CONTRIBUTIONS
G.A.H., A.W. and B.S. conceived and designed the outline and contributed equally to the review article. G.A.H., A.W. and B.S. contributed to the first draft of the manuscript. G.A.H., A.W. and B.S. led the development and editing of the manuscript.
LITERATURE CITED
Author notes
Alex Williams, Besiana Sinanaj and Grace A Hoysted contributed equally to the manuscript