-
PDF
- Split View
-
Views
-
Cite
Cite
W Sawangproh, L Hedenäs, A S Lang, B Hansson, N Cronberg, Gene transfer across species boundaries in bryophytes: evidence from major life cycle stages in Homalothecium lutescens and H. sericeum, Annals of Botany, Volume 125, Issue 4, 13 March 2020, Pages 565–579, https://doi.org/10.1093/aob/mcz209
- Share Icon Share
Abstract
The mosses Homalothecium lutescens and H. sericeum are genetically, morphologically and ecologically differentiated; mixed populations sometimes occur. In sympatric populations, intermediate character states among gametophytes and sporophytes have been observed, suggesting hybridization and introgression in such populations.
We determined genotypes using bi-allelic co-dominant single nucleotide polymorphism (SNP) markers, specific to either H. lutescens or H. sericeum, to estimate the degree of genetic mixing in 449 moss samples collected from seven sympatric and five allopatric populations on the island of Öland, south Sweden. The samples represented three generations: haploid maternal gametophytes; diploid sporophytes; and haploid sporelings.
Admixture analyses of SNP genotypes identified a majority as pure H. lutescens or H. sericeum, but 76 samples were identified as mildly admixed (17 %) and 17 samples (3.8 %) as strongly admixed. Admixed samples were represented in all three generations in several populations. Hybridization and introgression were bidirectional.
Our results demonstrate that admixed genomes are transferred between the generations, so that the populations behave as true hybrid zones. Earlier studies of sympatric bryophyte populations with admixed individuals have not been able to show that admixed alleles are transferred beyond the first generation. The presence of true hybrid zones has strong evolutionary implications because genetic material transferred across species boundaries can be directly exposed to selection in the long-lived haploid generation of the bryophyte life cycle, and contribute to local adaptation, long-term survival and speciation.
Introduction
Interspecific hybridization is a form of extreme outcrossing that is widespread in plants and fungi, as well as in some animals (Rieseberg, 1995; Rieseberg and Carney, 1998; Burke and Arnold, 2001; Schumer et al., 2004; Gross and Rieseberg, 2005; Baack and Rieseberg, 2007; Mallet, 2007; Soltis and Soltis, 2009). Many plant genera contain sister lineages, representing both outcrossing and inbreeding mating systems, that are known to hybridize (Jordan et al., 2018). The evolutionary significance of interspecific hybridization depends on the degree of gene exchange between genomes, as well as the ecological and reproductive characteristics of hybrid individuals and their offspring (Shaw, 1998; Harrison and Larson, 2014). Hybrid zones may serve as arenas for the transfer of genes from one taxon to another (via introgression) so that adaptive alleles potentially are moved into new genomic backgrounds (Natcheva and Cronberg, 2004; Paoletti et al., 2006; Whitney et al., 2010; Harrison and Larson, 2014). Extensive hybridization may either initiate breakdown, or reinforcement, of reproductive barriers between closely related species (Petit et al., 1999; Tubaro and Lijtmaer, 2002; Schumer et al., 2017). It is estimated that >50 % of the vascular plant species (Stebbins, 1950), and about 50–70 % of flowering plant species, have homoploid or allopolyploid hybrid origin (Stace, 1987; Ellstrand et al., 1996; Rieseberg, 1997).
Most studies of hybrid zones concern organisms with a dominant diploid generation in their life cycles. Plants commonly referred to as ‘bryophytes’ belong to three major lineages of non-vascular plants (the liverworts, hornworts and mosses), which are unique among land plants in having a dominant haploid generation and short-lived sporophyte generation. As a consequence of their life cycle, bryophytes are sometimes expected to have severely limited evolutionary potential (Anderson, 1963; Crum, 1972; Longton, 1976). Interspecific hybridization is rarely considered as an important evolutionary phenomenon in bryophytes; early observations (e.g. Philibert, 1873) have made bryologists believe that hybridization leads to aborted sporophytes or non-viable spores. In most genera of mosses, closely related species are distinguished by gametophytic rather than sporophytic characters (Shaw, 1994). Due to few distinguishing morphological characters and extensive phenotypic plasticity, identification of hybrids is usually intractable without genetic markers. Homoploid hybridization has been detected by molecular methods in a few genera (Shaw, 1994, 1998; Cronberg, 1996a, 1997; Cronberg and Natcheva, 2002; Natcheva and Cronberg, 2007; Ricca and Shaw, 2009) involving closely related species growing in close vicinity in mixed populations (Cronberg, 1996b; Shaw, 1998). In some cases, viable recombinant gametophytes were shown to occur in sympatric populations, identified with the help of genetic markers (Cronberg, 1996b; Shaw, 1998; Draper et al., 2007; Hernández-Maqueda et al., 2008; Hedenäs, 2011). The evolutionary implications of hybridization are impossible to evaluate without population-level studies regarding frequencies of hybrid sporophytes and recombinant gametophytes, their degrees of genomic admixture as well as their ability to contribute to future generations, i.e. to investigate if plants from putative hybrid zones are able to successfully complete their whole life cycle, including cross-fertilization between recombinants or backcrossing with parental species and subsequent production of viable spores. These kinds of studies are currently sorely missing, which is a major drawback since theories developed for the special case of life cycles with a dominant diploid generation do not necessarily fit well with organisms such as bryophytes, having a dominant haploid life cycle.
We considered two native species of the genus Homalothecium (Brachytheciaceae) to be a suitable study system because gametophytes that deviated from typical morphotypes of either of the two species, assumed to be of hybrid origin, had been found in mixed populations on the Baltic island of Öland (Lars Hedenäs and Frida Rosengren, pers. commun.).
The genus Homalothecium comprises nine morphological species (Hofmann, 1998) which have been confirmed by phylogenetic studies (Huttunen et al., 2008). The species in the genus typically have triangular leaves with small alar cells, and a glossy appearance (Huttunen et al., 2018). Recently, two additional species with regional distribution in Europe have been recognized within H. sericeum sensu lato (s.l.) by a combination of morphological and genetic studies (Hedenäs et al., 2009, 2014). Homalothecium sericeum and H. lutescens are perennial and dioicous medium-sized pleurocarpous mosses (Hofmann, 1998; Smith, 2004) commonly found in Europe and the only two known from Sweden (Nyholm, 1965; Hofmann, 1998; Huttunen et al., 2008; Lieske, 2010; GBIF Secretariat, 2018a, b). The estimated divergence between the two sister species is 3.39 million years (Huttunen et al., 2008). The two species are normally ecologically separated – H. sericeum grows epiphytically on tree trunks of broad-leaved trees or epilithically on calcareous stone or mortar in forests or parks, whereas H. lutescens grows on calcareous soil, usually in more open habitats such as pastures – but occasionally the two species grow intermixed in sympatric populations. The species also show a number of distinguishing characters in both the gametophyte and sporophyte generations (Watson, 1981; Hofmann, 1998; Smith, 2004; Atherton et al., 2010). A morphometric analysis (W. Sawangproh et al., unpubl. res.) confirmed the traditionally used distinguishing characters when the species were growing in their typical habitats, but revealed intermediate character states among gametophytes and sporophytes collected from seven populations in which the two species grow in close proximity (= sympatric populations) on Öland. Analysis of single nucleotide polymorphism (SNP) markers from the same study revealed genomic admixture between the two species in sympatric populations, although morphological intermediacy was not strongly associated with genomic admixture, suggesting introgression rather than complete genomic mixing in the populations.
Here, we present a more detailed genetic analysis of Homalothecium plants from seven sympatric populations together with five single-species reference populations (= allopatric populations). We analyse patterns of allelic SNP variation among samples in three generations: haploid (female) gametophytes (parental generation); diploid sporophyte and setae (F1 generation); and sporelings/recombinants (F2 generation). Our goals are: (1) to investigate the degree of interspecific mixing based on data from SNP markers in Homalothecium plants from sympatric populations compared with those from allopatric populations; (2) to test if admixture is symmetrical between the two parental species in recombinants and if completely admixed samples occur; and (3) to check if admixed genes are transmitted between gametophytes and sporophytes and between sporophytes and progeny.
MATERIALS AND METHODS
Study species and study site
Allopatric and sympatric populations of H. lutescens and H. sericeum were collected from different localities in the Baltic Island of Öland, Sweden (Fig. 1; Supplementary data Table S1). Populations from Öland were situated at the Great Alvar, a steppe-like and open area with a mosaic of base-rich and weakly acidic soils overlying the limestone bedrock (Köningsson, 1968; Bengtsson, et al., 1988; Runyeon-Lager and Prentice, 2000) or at the adjacent area with deciduous forest called Mittlandsskogen. In this study, we collected gametophytes in November, i.e. when sporophytes were ripe or nearly ripe (i.e. capsules with dark green to brownish colour) with undispersed spores (Fig. 1; Supplementary data Table S1). Gametophytes (small patches with/without capsules) were sampled from seven putatively hybridizing sympatric populations (HB1–HB7), three allopatric populations of H. sericeum (HS1–HS3) and two allopatric populations of H. lutescens (HL1 and HL2) at fixed points located every 2 m along limestone walls (as a line transect at each site; Fig. 2). This first sample set was used for DNA extraction of the gametophytes and, when present, separate extraction of associated sporophytes (seta + spore capsule). Additional sporulating gametophyte colonies with aberrant growth form (irregular and asymmetric branching pattern, different from both the parental species) were collected separately adjacent to the transect lines from the sympatric populations (HB1–HB7; Fig. 2). No such aberrant forms were found in the allopatric populations. This second sample set was used for DNA extraction of sporophytes (only seta = the same data set as in W. Sawangproh et al., unpubl. res.). The spores from the capsules were germinated and cultured for subsequent DNA extraction (see below).
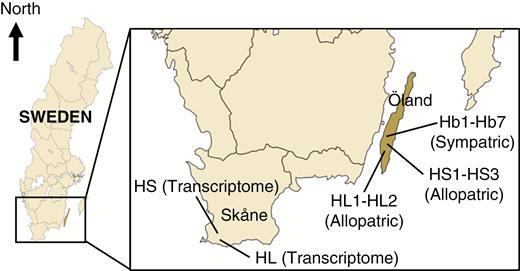
Simplified map (not to scale) showing locations of allopatric populations of H. lutescens (HL1 and HL2), allopatric populations of H. sericeum (HS1–HS3), sympatric populations (HB1–HB7) and allopatric populations for transcriptome data.
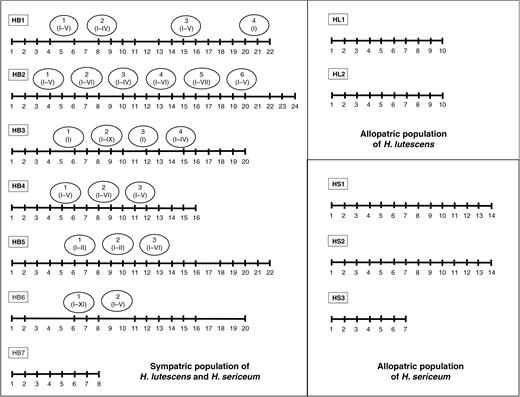
Gametophyte shoots were collected along line transects from allopatric populations of H. lutescens (HL1 and HL2) and H. sericeum (HS1–HS3) and from sympatric populations (HB1–HB7). Sampling points were separated by 2 m. Some sampling points are missing because of no plant cover (sampling points without vertical lines along the line transects). Additional sporulating gametophyte colonies with aberrant growth form along the transect lines were collected separately (marked by circles). Numbers of putative hybrid sporophytes collected from such colonies are indicated by Roman numerals (see more details in Supplementary data Table S1).
Development of species-specific SNP markers
In this study, transcriptomes were used for SNP discovery in Homalothecium plants for several reasons: (1) transcriptomes have been widely used for discovering SNPs in non-model organisms (reviewed in Ekblom and Galindo, 2011); (2) the species-specific SNP markers allow direct identification of hybrid genotypes by heterozygous diagnostic allele combinations; (3) the usually dense SNP abundance throughout the genome allows a high number of diagnostic markers to be developed (Clarke et al., 2014; Rusek et al., 2015); and (4) SNP markers have low mutation rates (Vignal et al., 2002; Batley et al., 2003). We used a similar approach for sample preparation and SNP selection from pooled samples to that described in Rosengren et al. (2015) to pick out SNPs that were species specific and expressed in both the gametophyte and sporophyte phases. Young shoots of H. lutescens and H. sericeum from allopatric populations were collected in different localities in Skåne (southern Sweden) (Fig. 1; Supplementary data Table S2). Before RNA extraction, samples were acclimatized by placing individual shoots onto a moist sandy substrate in a plastic box (himension: height 18 × width 33 × depth 40 cm) for 3 d in a culture room with full light illumination and constant temperature of approx. 12 °C. The boxes were aerated. Shoots with young green capsules were selected for extraction. Total RNA were extracted separately from the upper 25 mm of gametophyte apices and associated young green sporophytes of each specimen. Total RNA was extracted using the RNeasy Plant Mini Kit (Qiagen) with liquid nitrogen following the protocol of Rosengren (2015), followed by quality and quantity controls of extracts. Five gametophyte and five sporophyte RNA extracts from each of four localities (Bjärsjölagård, Klagshamn, Käglinge and Arrie; Supplementary data Table S2) were separately pooled for gametophytes (pool 1) and sporophytes (pool 2) for later construction of cDNA libraries for H. lutescens. Likewise, RNA extracts for H. sericeum were separately pooled from 20 gametophytes (pool 3) and 20 sporophytes (pool 4) from Dalby and 20 gametophytes (pool 5) and 20 sporophytes (pool 6) from Övedskloster. Six paired-end libraries were sequenced on an Illumina MiSeq Platform at the Sequencing Facility at the Department of Biology (Lund University). In total, 34.5 Mb of raw sequence data (17.2 Mb for gametophytes and 17.3 Mb for sporophytes) were obtained and analysed on the computational infrastructure UPPMAX (http://www.uppmax.uu.se) according to the scripts provided in Supplementary data Appendix .I. We used the program Trinity (launched 17 July 2014) for de novo reconstruction of transcriptomes from the RNA sequencing (RNA-seq) data of the gametophytes of H. sericeum to create a reference sequence (total assembled bases: 51 692 526). After reference construction, we mapped the sequence reads from both H. lutescens and H. sericeum to the reference assembly by using Bowtie (version 1.1.2) and exported output alignments in SAM format for SNP calling in SAMtools (version 0.1.19). A final number of 79 314 contiguous sequences (=contigs) (total contigs created: 324 101) representing 42 481 248 reads (H. lutescens, 10 289 896; H. sericeum, 32 191 352) remained to be analysed from the mapping to the reference sequence. The SNP calling was performed by a custom-made Perl program (available upon request). In total, 4631 putative SNPs with read coverage at the SNP position of least 20 within 100 bp upstream or downstream of the targeted SNP positions were extracted by SAMtools. The 133 highest quality SNPs, which displayed species-specific variants (alleles), were chosen for SNP genotyping.
DNA analyses from allopatric and sympatric populations
Samples from allopatric populations (= reference populations) and sympatric populations (= putatively hybridizing populations) of H. lutescens and H. sericeum (Supplementary data Table S1) were brought to the laboratory and cultured in a growth chamber at a constant temperature of approx. 12 °C and a photoperiod of 16 h:8 h (light:dark). Before DNA extraction, shoots were sorted individually into mature gametophytes (= P generation), immature sporophytes (= F1 generation) and ripe sporophytes. The material sampled from fixed points (first sample set, see above) and the additional material with ‘aberrant’ morphology (second sample set) were treated somewhat differently. (1) Gametophytes from all fixed sample points were extracted separately. If sporophytes were present on these gametophytes, one or two of the attached entire sporophytes were selected and extracted separately. (2) From gametophytes with ‘aberrant’ morphology, the sporophytes were cut into two pieces, the seta and the spore capsule. Spores were harvested from one or two spore capsules from each gametophyte and sown out on agar plates in sterilized conditions (see the spore germination method in Supplementary data Appendix II) to obtain sporelings (= F2 generation) for growth and later extraction. The setae from these sporophytes were immediately separately extracted. The remaining part of each sample was air-dried and kept in paper bags as voucher specimens at the Botanical Museum at Lund University. DNA was extracted from mature gametophytes, immature sporophytes and sporelings using a standard DNA extraction kit (Qiagen DNeasy Plant MiniKit). We decided to combine sporelings germinated from a single sporophyte with high germination success (>30 % of spore germination) by randomly picking 25 sporelings from each agar plate for extraction in order to gain enough DNA. In the case of low germination success (≤30% spore germination), we combined all available sporelings in the extractions. Samples were extracted with the same standard DNA extraction kit. In total, 464 samples were extracted: 173 haploid female gametophyte, 210 diploid sporophytes and 81 (combined) haploid sporeling samples. All DNA samples were sent for SNP genotyping performed at SciLifeLab Uppsala with the multiplexed primer extension (SBE) chemistry of the iPLEX assay.
Data analyses: examination of genetic structure
The 111 SNP markers were subjected to Bayesian analyses using methods executed in the program STRUCTURE version 2.3.4 (Pritchard et al., 2000). The ‘admixture model’ of STRUCTURE assumes that each individual has ancestry from one or more of K assumed populations or genetic groups. The admixture coefficient (q) describes the proportion of an individual genotype that originates from each of the K categories. In the present study, we set K = 2 corresponding to the assumed two species contributing to the gene pool of the sample. No prior species information was used in any of the analyses. The SNP data were treated as being diploid (ploidy = 2) and thus coding the haploids as being homozygotes. We used an admixture model of ancestry, with correlated allele frequencies. We used a burn-in of 20 000 steps and 50 000 replications. In assigning each sample to a class, we used q = 0.90 as the threshold (Burgarella et al., 2009; Melville et al., 2016): samples with q ≥ 0.90 were assigned to the purebred category and samples with q < 0.90 were assigned to the admixed category (Hert et al., 2011). However, the STRUCTURE results were treated with caution because the assumption of Hardy–Weinberg equilibrium is not met due to the different ploidy level in gametophytes (=haploid), the sporophytes/setae (=diploid) and sporelings (=haploid). For improved visualization, resulting graphs from STRUCTURE were edited in Adobe Photoshop.
Multivariate analysis
We performed principal co-ordinate analysis (PCoA) with the Excel plugin GenAlEx version 6.5 (Peakall and Smouse, 2012), using the co-dominant genetic distance matrix generated from the 111 SNPs (with data standardization) to elucidate the genetic relationships between the samples of mature female gametophytes, sporophytes and sporelings. To improve the visualization of the genetic differentiation between the parental species and the putative hybrids, resulting graphs were edited in Adobe Photoshop.
Hybrid index analysis
To evaluate the degree of interspecific mixing of genotypes, all samples were assigned a ‘hybrid index’ based on the presence of alleles characteristic of H. lutescens or H. sericeum in 85 alternatively fixed nuclear SNPs. At a particular locus, 0 denoted homozygosity for the H. lutescens allele, 1 homozygosity for the H. sericeum allele and 0.5 heterozygosity (one allele from each of the species). The hybrid index for each genotype was the mean across the values for the 85 SNP loci, therefore ranging from 0 (pure H. sericeum) to 1.0 (pure H. lutescens), with all values in between indicating admixed genotypes; 0.5 indicated completely balanced representation of the two parental genomes.
Paternity analysis
The data set involving maternal gametophytes (G) and their attached sporophytes (S) was used to determine what degree of genomic admixture occurred in sporophytes and to calculate the genetic distance between parents. As the diploid sporophyte genotype is a result of fusion between the haploid paternal and maternal gametophyte genotypes (= F1 generation), the paternal haplotype for each sporophyte was derived by subtracting the maternal gametophyte haplotype from the diploid sporophyte genotype. The total data set of 114 gametophytes included 72 maternal shoots with sporophytes, from which one to three sporophytes were analysed per maternal shoot.
Progeny analysis
The data set involving sporophytes (setae) and sporelings was used to check if SNP alleles present in the sporophyte were also transmitted to the sporelings (R = recombinants; representing the F2 generation). This data set included sporelings from 81 sporophytes (out of 115 sporophytes from the second sample set; see Supplementary data Table S1) that successfully germinated. The sporelings from each of the 81 sporophytes were analysed together, as described above, to give a composite representation of the parental genomes in the SNP typing, i.e. with an (artificial) diploid expression. This strategy was adopted to reduce the number of extractions, but also because the sporelings grew intermingled and had small amounts of DNA, which made it difficult to extract individual sporelings. Consequently, we could not ascertain the genotypes of individual sporelings or determine their individual degrees of admixture.
RESULTS
SNP genotyping
Of the 133 SNP markers in the iPLEX assay, 111 resulted in genotyping and were informative (i.e. produced allelic data and were polymorphic). We blasted the contigs where each SNP marker was located to predict their cellular compartmentalization (nuclear, mitochondrial or chloroplastic). Our initial intention was to use plastid and mitochondrial SNPs as indicators of the maternal parent species. A few contigs were matched against sequences of Physomitrella patens (the most studied moss genome model organism) that suggested plastid or mitochondrial origins, but the inheritance patterns of these revealed that all of these were nuclear, so in the end we treated all SNPs as nuclear SNP markers. Of the 111 SNP markers, 85 were alternatively fixed markers for either H. lutescens or H. sericeum (i.e. species-specific markers), and 26 were polymorphic in one or both parental species (non-species-specific markers) in the allopatric reference populations (Supplementary data Table S3). The frequency of samples with heterozygous genotypes in the species-specific and non-species-specific SNPs are shown in Supplementary data Fig. S1. The total proportion of missing data at the 111 SNPs (failed genotyping) was 20.14 %. Out of 466 individuals, 17 individuals (i.e. ten setae, five gametophytes and two aggregate sporelings) completely failed in the SNP genotyping. Most of these were omitted from the study except the six setae (= NA in Supplementary data Table S4) for which SNP genotyping failed but where spores germinated to a sufficient extent for successful SNP genotyping of aggregated sporelings.
Structure analysis
For the STRUCTURE analysis, the most likely number of clusters was chosen at K = 2 (Fig. 3), which equates to samples being assigned either as H. lutescens or as H. sericeum. The results from the STRUCTURE analysis showed that the majority of samples were ‘pure’ species with a probability exceeding the threshold value for q (the admixture coefficient) of 0.9. However, we observed evidence of hybridization, with samples classified as admixed between H. lutescens and H. sericeum when 0.90 > q > 0.10. In five of the seven sympatric populations, admixed samples were found, and in total 17 samples of the 449 (3.8%) from the sympatric populations were identified as being admixed. Of these 17 admixed samples, six were haploid gametophytes, six diploid sporophytes and five haploid recombinants (Fig. 3). Five admixed samples (samples 112, 119, 120, 122 and 124) had q values between 45 and 55 %, i.e. a balanced representation of both the parental genomes, whereas the other 12 samples had q values >55 % for one cluster (and thus <45 % for the other cluster), indicating skewed admixture towards either of the Homalothecium species (nine samples: 34, 123, 189, 321, 322, 327, 329, 330 and 368, towards H. sericeum; three samples: 374, 375 and 376, towards H. lutescens).
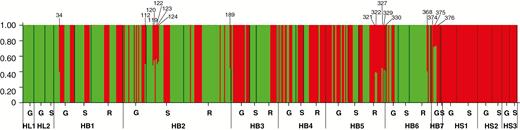
Clustering results for all samples obtained for K = 2, using the program STRUCTURE. Each sample is represented by a vertical bar partitioned into K coloured segments that correspond to the estimated membership proportions (q, admixure coefficients) for each parental species (H. lutescens = green colour and H. sericeum = red colour). The samples identified as hybrids by STRUCTURE, at a threshold (q = 0.90), are indicated by numbers. HL1 and HL2, allopatric populations of H. lutescens; HB1–HB7, sympatric populations; HS1–HS3, allopatric populations of H. sericeum; G, haploid gametophytes; S, diploid sporophytes; R, haploid recombinants.
PCoA analysis
The PCoA based on 111 SNP markers from 97 samples from allopatric and 352 samples from sympatric populations revealed that 79.08 % of the total variance in the data set is explained by the first two factors (co-ordinate 1 = 64.54 %, mostly summarizing the genetic difference between the two parental species; co-ordinate 2 = 14.54 %, mostly reflecting degrees of missing values of SNP markers; Fig. 4). The PCoA shows a strong genetic differentiation between two major groups corresponding to the two species along both axes, with 17 genetically differentiated samples in between (Fig. 4). The role of the non-specific markers (most of them were polymorphic in one of the parental species) is less likely to contribute strongly to the separation of samples within the clusters of each species, since running the PCoA after excluding non-specific SNP markers gave the same resulting picture.
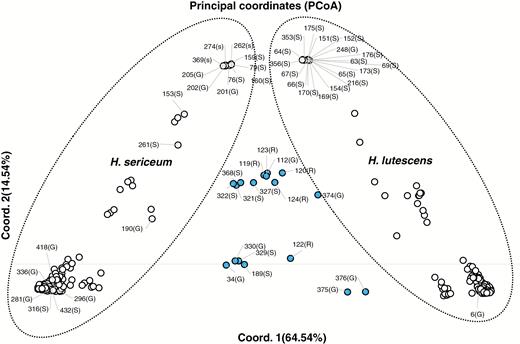
A distance-based (PCoA) plot of genetic structure, based on genetic distance of 111 SNPs between samples of Homalothecium plants. The circles encircling H. lutescens and H. sericeum were drawn on the basis of the STRUCTURE analysis in Fig. 3. Strongly admixed genotypes are indicated by blue dots labelled by their ID numbers and mildly admixed genotypes are indicated by white dots labelled by their ID numbers. The percentage of genetic distance explained by each of the PCoA axes is provided in parentheses. G, haploid gametophytes; S, diploid sporophytes; R, haploid recombinants.
Hybrid index analysis
Based on 85 species-specific SNPs, 93 of 352 samples (26 %) were identified as admixed in the hybrid index analysis: 16 haploid gametophytes, 51 diploid sporophytes and 26 haploid recombinants (see the upper panel in Fig. 5). Seventeen out of 93 admixed samples (18 %) displayed a balanced representation of the parental genomes (the hybrid index is between 0.3 and 0.7) and the other 76 samples (82 %) displayed admixture biased towards one or the other of the parental species: 42 samples (55 %) towards H. lutescens (the hybrid index is between 0.7 and 1.0) and 34 samples (45 %) towards H. sericeum (the hybrid index between 0.0 and 0.3). Out of 17 samples with balanced genomic representation, six were gametophytes, six were sporophytes and five were recombinants (see the lower panel in Fig. 5), i.e. all generations were represented among these strongly admixed samples.
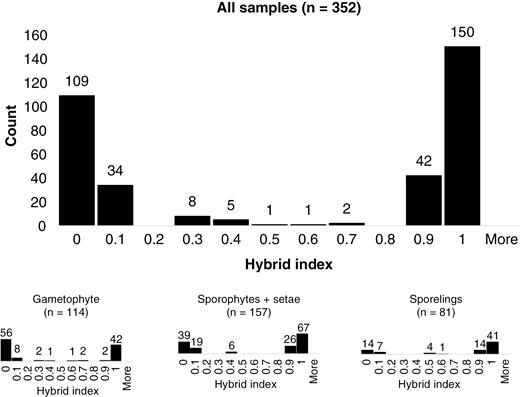
Histogram showing the distribution of the hybrid index for samples of Homalothecium (upper panel = all samples combined; lower panel = samples classified by life generations) from Öland, calculated from allelic profiles at 85 species-specific SNP loci. Samples with a hybrid index of 0 are H. sericeum without admixture, those with an index of 1 are H. lutescens without admixture; intermediate indices represent samples with various levels of genomic admixture of the two parental species.
Genomic comparisons across generations
The maternal gametophyte population as well as the composite sporeling samples showed a low mean level of detection failure in SNP genotyping (Supplementary data Table S4). On the other hand, analysis of DNA extracts from spore capsules showed a considerably higher failure rate in SNP genotyping, and even higher for the seta samples. This was not unexpected because low amounts of DNA were extracted from the setae, which had little biomass.
Genomic comparisons of maternal gametophytes and attached sporophytes
In total, 93 sporophytes from 72 maternal gametophytes were used for comparison of maternal and sporophyte genotypes from the allopatric and sympatric populations (see Supplementary data Table S4). Fifty out of 93 sporophytes were from the sympatric populations and the remaining sporophytes were from the allopatric populations (HL2 and HS1–HS3). In the sympatric populations, 50 sporophytes were collected from 50 gametophytes that were sporophytic (50 out of 114 gametophytes; 44 %). Out of 114 gametophytes, 17 were admixed (15 %: some with, and some without, sporophytes; Fig. 6).
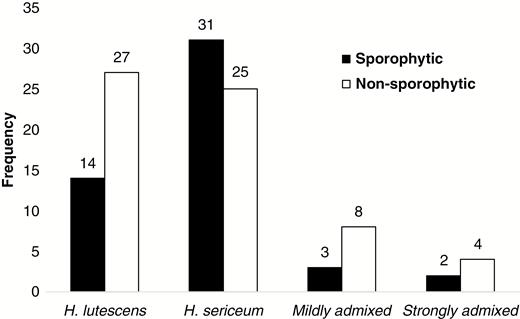
Number of maternal gametophytes with and without sporophytes found in sympatric populations classified by the 85 species-specific nuclear SNP markers. Mildly admixed = maternal gametophytes displayed admixed genotypes but biased towards either H. lutescens or H. sericeum. Strongly admixed = maternal gametophytes displaying balanced parental genomic representation.
The sub-set of 50 diploid sporophytic genotypes and their maternal gametophytes in the sympatric populations showed that 36 sporophytes (72 %) lacked any sign of admixture, and had genomes that were typical for H. lutescens (12 sporophytes) or H. sericeum (24 sporophytes). Fourteen sporophytes (28 %, samples labelled with black arrows in Fig. 7) were identified as admixed because they possessed heterozygotic allele(s) and/or occasional homozygotic allele(s) that differed from their maternal gametophytes and were diagnostic for the other parental species (Fig. 7). The majority of admixed sporophytes (eight out of 14) displayed genomes dominated by one of the parental species, whereas the remaining six sporophytes displayed genomes with more or less equal numbers of SNP markers from both parents (samples labelled with asterisks in Fig. 7).
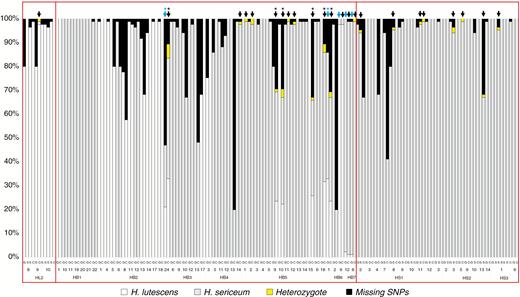
Proportions of SNPs in the maternal haploid gametophyte (G) and the attached diploid seta (S) or complete sporophyte (C) in allopatric populations of H. lutescens (HL2), H. sericeum (HS1–HS3) and sympatric populations of Homalothecium from Öland (HB1–HB7), respectively. Arabic numbers correspond to the numbers of sampling points in Fig. 1. Blue arrow, admixed maternal gametophyte; black arrow, admixed sporophyte; *, individuals with equal (balanced) representation of the two parental genomes.
Notably, we found nine admixed sporophytes (two of them derived from the same maternal plant) from allopatric populations, with each one or sometimes two ‘misplaced’ alleles in our hybrid index analysis (one out of eight sporophytes from an allopatric population of H. lutescens and eight out of 35 sporophytes from three allopatric populations of H. sericeum; see Fig. 7). These admixed/misplaced alleles represented two loci (i.e. 31 and 51) in one of the allopatric populations of H. lutescens and four loci in three of the allopatric populations of H sericeum (i.e. 6, 51, 54 and 70). None of these misplaced alleles was retrieved in any of the gametophytes from the allopatric populations (Fig. 7).
Among the 14 admixed sporophytes from the sympatric populations, seven had H. sericeum as maternal plants, two had H. lutescens as maternal plants, two had mildly admixed H. sericeum as maternal plants, one had mildly admixed H. lutescens as maternal plant and two maternal plants were admixed to the degree that they could not be assigned to species (Table 1). The contribution to sporophytes (F1 generation) by H. sericeum as the paternal plant varied from 1 % in mildly admixed sporophytes to 68 % in strongly admixed sporophytes, while the contribution of H. lutescens as the paternal plant varied from 2 % in mildly admixed sporophytes to 43 % in strongly admixed sporophytes (Table 1).
No. of sporophytes . | No. of maternal plants . | Pop . | No. of alleles determined (%), species . | Remarks . | ||
---|---|---|---|---|---|---|
. | . | . | Present only in mother . | Present in both sporophyte and mother . | No. of inferred alleles from father, paternal species . | |
1 | 1 | HL2 | 67 (97 %), HL | 69 (100 %), mildly admixed HL | 2? (3 %), HL | The male contribution cannot be established for two loci. The sporophyte is heterozygotic, but the female SNP data are missing |
2 | 2 | HB2 | 38 (88 %), strongly admixed | 43 (100 %), strongly admixed | 5? (12 %), Sp.? | The male contribution cannot be established for five loci. The sporophyte is heterozygotic, but the female SNP data are missing |
3 | 3 | HB2 | 81 (99 %), HS | 82 (100 %), mildly admixed HS | 1 (1 %), HS | One HL allele admixed to a HS male genotype |
4 | 4 | HB5 | 84 (99 %), HS | 85 (100 %), mildly admixed HS | 1 (1 %), HS | One HL allele admixed to a HS male genotype |
5 | 5 | HB5 | 83 (99 %), HS | 84 (100 %), mildly admixed HS | 1 (1 %), HS | One HL allele admixed to a HS male genotype |
6 | 6 | HB5 | 25 (57 %), HS | 44 (100 %), strongly admixed | 19 (43 %), HL | |
7 | 7 | HB5 | 18 (32 %), HL | 57 (100 %), strongly admixed | 39 (68 %), HS | |
8 | 8 | HB5 | 82 (99 %), HS | 83 (100 %), mildly admixed HS | 1 (1 %), HS | One HL allele admixed to a HS male genotype |
9 | 9 | HB5 | 82 (99 %), HS | 83 (100 %), mildly admixed HS | 1 (1 %), HS | One HL allele admixed to a HS male genotype |
10 | 10 | HB5 | 22 (29 %), HL | 57 (100 %), strongly admixed | 35 (61 %), HS | |
11 | 11 | HB5 | 46 (61 %), HS | 76 (100 %), strongly admixed | 30 (39 %), HL | |
12 | 12 | HB5 | 54 (96 %), strongly admixed | 56 (100 %), strongly admixed | 2? (4 %), Sp.? | The male contribution cannot be established for two loci. The sporophyte is heterozygotic, but the female SNP data are missing |
13 | 13 | HB5 | 83 (98 %), mildly admixed HL | 85 (100 %), mildly admixed HL | 2 (2 %), HL | Two HS alleles admixed to both a HL female genotype and a HL male genotype |
14 | 14 | HB5 | 83 (99 %), mildly admixed HS | 84 (100 %), mildly admixed HS | 1 (1 %), HS | One HL allele admixed to both a HS female genotype and a HS male genotype |
15 | 15 | HB6 | 83 (99 %), mildly admixed HS | 84 (100 %), mildly admixed HS | 1 (1 %), HS | One HL allele admixed to a HS female genotype and one HS allele admixed to a HS male genotype |
16 | 16 | HS1 | 80 (99 %), HS | 81 (100 %), mildly admixed HS | 1 (1 %), HS | One HL allele admixed to a HS male genotype |
17 | 17 | HS1 | 66 (99 %), HS | 67 (100 %), mildly admixed HS | 1 (1 %), HS | One HL allele admixed to a HS male genotype |
18 | 18 | HS1 | 82 (99 %), HS | 83 (100 %), mildly admixed HS | 1? (1%), HS | The male contribution cannot be established for one locus. The sporophyte is heterozygotic, but the female SNP data are missing |
19 | HS1 | 82 (99 %), HS | 83 (100 %), mildly admixed HS | 1? (1 %), HS | The male contribution cannot be established for one locus. The sporophyte is heterozygotic, but the female SNP data are missing | |
20 | 19 | HS2 | 79 (97 %), HS | 81 (100 %), mildly admixed HS | 2 (3 %), HS | Two HL alleles admixed to a HS male genotype |
21 | 20 | HS2 | 84 (99 %), HS | 85 (100 %), mildly admixed HS | 1 (1 %), HS | One HL allele admixed to a HS male genotype |
22 | 21 | HS2 | 57 (99 %), HS | 58 (100 %), mildly admixed HS | 1 (1 %), HS | One HL allele admixed to a HS male genotype |
23 | 22 | HS3 | 69 (99 %), HS | 70 (100 %), mildly admixed HS | One | 1 HL allele admixed to a HS male genotype |
No. of sporophytes . | No. of maternal plants . | Pop . | No. of alleles determined (%), species . | Remarks . | ||
---|---|---|---|---|---|---|
. | . | . | Present only in mother . | Present in both sporophyte and mother . | No. of inferred alleles from father, paternal species . | |
1 | 1 | HL2 | 67 (97 %), HL | 69 (100 %), mildly admixed HL | 2? (3 %), HL | The male contribution cannot be established for two loci. The sporophyte is heterozygotic, but the female SNP data are missing |
2 | 2 | HB2 | 38 (88 %), strongly admixed | 43 (100 %), strongly admixed | 5? (12 %), Sp.? | The male contribution cannot be established for five loci. The sporophyte is heterozygotic, but the female SNP data are missing |
3 | 3 | HB2 | 81 (99 %), HS | 82 (100 %), mildly admixed HS | 1 (1 %), HS | One HL allele admixed to a HS male genotype |
4 | 4 | HB5 | 84 (99 %), HS | 85 (100 %), mildly admixed HS | 1 (1 %), HS | One HL allele admixed to a HS male genotype |
5 | 5 | HB5 | 83 (99 %), HS | 84 (100 %), mildly admixed HS | 1 (1 %), HS | One HL allele admixed to a HS male genotype |
6 | 6 | HB5 | 25 (57 %), HS | 44 (100 %), strongly admixed | 19 (43 %), HL | |
7 | 7 | HB5 | 18 (32 %), HL | 57 (100 %), strongly admixed | 39 (68 %), HS | |
8 | 8 | HB5 | 82 (99 %), HS | 83 (100 %), mildly admixed HS | 1 (1 %), HS | One HL allele admixed to a HS male genotype |
9 | 9 | HB5 | 82 (99 %), HS | 83 (100 %), mildly admixed HS | 1 (1 %), HS | One HL allele admixed to a HS male genotype |
10 | 10 | HB5 | 22 (29 %), HL | 57 (100 %), strongly admixed | 35 (61 %), HS | |
11 | 11 | HB5 | 46 (61 %), HS | 76 (100 %), strongly admixed | 30 (39 %), HL | |
12 | 12 | HB5 | 54 (96 %), strongly admixed | 56 (100 %), strongly admixed | 2? (4 %), Sp.? | The male contribution cannot be established for two loci. The sporophyte is heterozygotic, but the female SNP data are missing |
13 | 13 | HB5 | 83 (98 %), mildly admixed HL | 85 (100 %), mildly admixed HL | 2 (2 %), HL | Two HS alleles admixed to both a HL female genotype and a HL male genotype |
14 | 14 | HB5 | 83 (99 %), mildly admixed HS | 84 (100 %), mildly admixed HS | 1 (1 %), HS | One HL allele admixed to both a HS female genotype and a HS male genotype |
15 | 15 | HB6 | 83 (99 %), mildly admixed HS | 84 (100 %), mildly admixed HS | 1 (1 %), HS | One HL allele admixed to a HS female genotype and one HS allele admixed to a HS male genotype |
16 | 16 | HS1 | 80 (99 %), HS | 81 (100 %), mildly admixed HS | 1 (1 %), HS | One HL allele admixed to a HS male genotype |
17 | 17 | HS1 | 66 (99 %), HS | 67 (100 %), mildly admixed HS | 1 (1 %), HS | One HL allele admixed to a HS male genotype |
18 | 18 | HS1 | 82 (99 %), HS | 83 (100 %), mildly admixed HS | 1? (1%), HS | The male contribution cannot be established for one locus. The sporophyte is heterozygotic, but the female SNP data are missing |
19 | HS1 | 82 (99 %), HS | 83 (100 %), mildly admixed HS | 1? (1 %), HS | The male contribution cannot be established for one locus. The sporophyte is heterozygotic, but the female SNP data are missing | |
20 | 19 | HS2 | 79 (97 %), HS | 81 (100 %), mildly admixed HS | 2 (3 %), HS | Two HL alleles admixed to a HS male genotype |
21 | 20 | HS2 | 84 (99 %), HS | 85 (100 %), mildly admixed HS | 1 (1 %), HS | One HL allele admixed to a HS male genotype |
22 | 21 | HS2 | 57 (99 %), HS | 58 (100 %), mildly admixed HS | 1 (1 %), HS | One HL allele admixed to a HS male genotype |
23 | 22 | HS3 | 69 (99 %), HS | 70 (100 %), mildly admixed HS | One | 1 HL allele admixed to a HS male genotype |
The number of paternal alleles (%) in the sporophyte (F1 generation) as inferred by counting the number of alleles present in the sporophytes and absent in the maternal plants. The designation to species of maternal plants and hypothetic paternal plants is indicated; HL, H. lutescens; HS, H. sericeum (n = 23).
No. of sporophytes . | No. of maternal plants . | Pop . | No. of alleles determined (%), species . | Remarks . | ||
---|---|---|---|---|---|---|
. | . | . | Present only in mother . | Present in both sporophyte and mother . | No. of inferred alleles from father, paternal species . | |
1 | 1 | HL2 | 67 (97 %), HL | 69 (100 %), mildly admixed HL | 2? (3 %), HL | The male contribution cannot be established for two loci. The sporophyte is heterozygotic, but the female SNP data are missing |
2 | 2 | HB2 | 38 (88 %), strongly admixed | 43 (100 %), strongly admixed | 5? (12 %), Sp.? | The male contribution cannot be established for five loci. The sporophyte is heterozygotic, but the female SNP data are missing |
3 | 3 | HB2 | 81 (99 %), HS | 82 (100 %), mildly admixed HS | 1 (1 %), HS | One HL allele admixed to a HS male genotype |
4 | 4 | HB5 | 84 (99 %), HS | 85 (100 %), mildly admixed HS | 1 (1 %), HS | One HL allele admixed to a HS male genotype |
5 | 5 | HB5 | 83 (99 %), HS | 84 (100 %), mildly admixed HS | 1 (1 %), HS | One HL allele admixed to a HS male genotype |
6 | 6 | HB5 | 25 (57 %), HS | 44 (100 %), strongly admixed | 19 (43 %), HL | |
7 | 7 | HB5 | 18 (32 %), HL | 57 (100 %), strongly admixed | 39 (68 %), HS | |
8 | 8 | HB5 | 82 (99 %), HS | 83 (100 %), mildly admixed HS | 1 (1 %), HS | One HL allele admixed to a HS male genotype |
9 | 9 | HB5 | 82 (99 %), HS | 83 (100 %), mildly admixed HS | 1 (1 %), HS | One HL allele admixed to a HS male genotype |
10 | 10 | HB5 | 22 (29 %), HL | 57 (100 %), strongly admixed | 35 (61 %), HS | |
11 | 11 | HB5 | 46 (61 %), HS | 76 (100 %), strongly admixed | 30 (39 %), HL | |
12 | 12 | HB5 | 54 (96 %), strongly admixed | 56 (100 %), strongly admixed | 2? (4 %), Sp.? | The male contribution cannot be established for two loci. The sporophyte is heterozygotic, but the female SNP data are missing |
13 | 13 | HB5 | 83 (98 %), mildly admixed HL | 85 (100 %), mildly admixed HL | 2 (2 %), HL | Two HS alleles admixed to both a HL female genotype and a HL male genotype |
14 | 14 | HB5 | 83 (99 %), mildly admixed HS | 84 (100 %), mildly admixed HS | 1 (1 %), HS | One HL allele admixed to both a HS female genotype and a HS male genotype |
15 | 15 | HB6 | 83 (99 %), mildly admixed HS | 84 (100 %), mildly admixed HS | 1 (1 %), HS | One HL allele admixed to a HS female genotype and one HS allele admixed to a HS male genotype |
16 | 16 | HS1 | 80 (99 %), HS | 81 (100 %), mildly admixed HS | 1 (1 %), HS | One HL allele admixed to a HS male genotype |
17 | 17 | HS1 | 66 (99 %), HS | 67 (100 %), mildly admixed HS | 1 (1 %), HS | One HL allele admixed to a HS male genotype |
18 | 18 | HS1 | 82 (99 %), HS | 83 (100 %), mildly admixed HS | 1? (1%), HS | The male contribution cannot be established for one locus. The sporophyte is heterozygotic, but the female SNP data are missing |
19 | HS1 | 82 (99 %), HS | 83 (100 %), mildly admixed HS | 1? (1 %), HS | The male contribution cannot be established for one locus. The sporophyte is heterozygotic, but the female SNP data are missing | |
20 | 19 | HS2 | 79 (97 %), HS | 81 (100 %), mildly admixed HS | 2 (3 %), HS | Two HL alleles admixed to a HS male genotype |
21 | 20 | HS2 | 84 (99 %), HS | 85 (100 %), mildly admixed HS | 1 (1 %), HS | One HL allele admixed to a HS male genotype |
22 | 21 | HS2 | 57 (99 %), HS | 58 (100 %), mildly admixed HS | 1 (1 %), HS | One HL allele admixed to a HS male genotype |
23 | 22 | HS3 | 69 (99 %), HS | 70 (100 %), mildly admixed HS | One | 1 HL allele admixed to a HS male genotype |
No. of sporophytes . | No. of maternal plants . | Pop . | No. of alleles determined (%), species . | Remarks . | ||
---|---|---|---|---|---|---|
. | . | . | Present only in mother . | Present in both sporophyte and mother . | No. of inferred alleles from father, paternal species . | |
1 | 1 | HL2 | 67 (97 %), HL | 69 (100 %), mildly admixed HL | 2? (3 %), HL | The male contribution cannot be established for two loci. The sporophyte is heterozygotic, but the female SNP data are missing |
2 | 2 | HB2 | 38 (88 %), strongly admixed | 43 (100 %), strongly admixed | 5? (12 %), Sp.? | The male contribution cannot be established for five loci. The sporophyte is heterozygotic, but the female SNP data are missing |
3 | 3 | HB2 | 81 (99 %), HS | 82 (100 %), mildly admixed HS | 1 (1 %), HS | One HL allele admixed to a HS male genotype |
4 | 4 | HB5 | 84 (99 %), HS | 85 (100 %), mildly admixed HS | 1 (1 %), HS | One HL allele admixed to a HS male genotype |
5 | 5 | HB5 | 83 (99 %), HS | 84 (100 %), mildly admixed HS | 1 (1 %), HS | One HL allele admixed to a HS male genotype |
6 | 6 | HB5 | 25 (57 %), HS | 44 (100 %), strongly admixed | 19 (43 %), HL | |
7 | 7 | HB5 | 18 (32 %), HL | 57 (100 %), strongly admixed | 39 (68 %), HS | |
8 | 8 | HB5 | 82 (99 %), HS | 83 (100 %), mildly admixed HS | 1 (1 %), HS | One HL allele admixed to a HS male genotype |
9 | 9 | HB5 | 82 (99 %), HS | 83 (100 %), mildly admixed HS | 1 (1 %), HS | One HL allele admixed to a HS male genotype |
10 | 10 | HB5 | 22 (29 %), HL | 57 (100 %), strongly admixed | 35 (61 %), HS | |
11 | 11 | HB5 | 46 (61 %), HS | 76 (100 %), strongly admixed | 30 (39 %), HL | |
12 | 12 | HB5 | 54 (96 %), strongly admixed | 56 (100 %), strongly admixed | 2? (4 %), Sp.? | The male contribution cannot be established for two loci. The sporophyte is heterozygotic, but the female SNP data are missing |
13 | 13 | HB5 | 83 (98 %), mildly admixed HL | 85 (100 %), mildly admixed HL | 2 (2 %), HL | Two HS alleles admixed to both a HL female genotype and a HL male genotype |
14 | 14 | HB5 | 83 (99 %), mildly admixed HS | 84 (100 %), mildly admixed HS | 1 (1 %), HS | One HL allele admixed to both a HS female genotype and a HS male genotype |
15 | 15 | HB6 | 83 (99 %), mildly admixed HS | 84 (100 %), mildly admixed HS | 1 (1 %), HS | One HL allele admixed to a HS female genotype and one HS allele admixed to a HS male genotype |
16 | 16 | HS1 | 80 (99 %), HS | 81 (100 %), mildly admixed HS | 1 (1 %), HS | One HL allele admixed to a HS male genotype |
17 | 17 | HS1 | 66 (99 %), HS | 67 (100 %), mildly admixed HS | 1 (1 %), HS | One HL allele admixed to a HS male genotype |
18 | 18 | HS1 | 82 (99 %), HS | 83 (100 %), mildly admixed HS | 1? (1%), HS | The male contribution cannot be established for one locus. The sporophyte is heterozygotic, but the female SNP data are missing |
19 | HS1 | 82 (99 %), HS | 83 (100 %), mildly admixed HS | 1? (1 %), HS | The male contribution cannot be established for one locus. The sporophyte is heterozygotic, but the female SNP data are missing | |
20 | 19 | HS2 | 79 (97 %), HS | 81 (100 %), mildly admixed HS | 2 (3 %), HS | Two HL alleles admixed to a HS male genotype |
21 | 20 | HS2 | 84 (99 %), HS | 85 (100 %), mildly admixed HS | 1 (1 %), HS | One HL allele admixed to a HS male genotype |
22 | 21 | HS2 | 57 (99 %), HS | 58 (100 %), mildly admixed HS | 1 (1 %), HS | One HL allele admixed to a HS male genotype |
23 | 22 | HS3 | 69 (99 %), HS | 70 (100 %), mildly admixed HS | One | 1 HL allele admixed to a HS male genotype |
The number of paternal alleles (%) in the sporophyte (F1 generation) as inferred by counting the number of alleles present in the sporophytes and absent in the maternal plants. The designation to species of maternal plants and hypothetic paternal plants is indicated; HL, H. lutescens; HS, H. sericeum (n = 23).
According to the paternity analysis of the mildly admixed sporophytes (Table 1), the paternal parent was in most cases inferred to be similar to the maternal parent, and thus referred to the same species, but admixed and providing 1–2 alleles specific to the other species (Table 1). In several of the strongly admixed sporophytes, the female parent is essentially a pure species (either H. lutescens or H. sericeum) and the male appears to belong to the other species (either H. lutescens or H. sericeum), based on the paternity analysis. The male contribution is visible not only as heterozygotes at occasional loci, but more commonly by the presence of the male alleles in homozygotic conditions. The female allele is apparently below the detection limit for these loci. This means that the assumption of F1 hybrid sporophytes having heterozygotes in all species-specific loci does not hold true. On five occasions (sporophyte nos 1, 2, 12, 18 and 19) we found heterozygotic expression in the sporophyte, but no allele detection in the maternal gametophyte, so that it was not possible to determine which allele had been provided by the mother and father, respectively (Table 1).
Genomic comparison of sporophytes and recombinants (sporelings)
The analysis of the genome in sporelings germinated from 83 sporophytes collected from sympatric populations showed that the majority of aggregated SNP genotypes of sporelings were identical to the diploid genotypes of parental sporophytes. The majority of alleles detected in the sporophyte were transmitted to their sporelings, although the failure rate (no SNP allele detection) was much higher for SNPs in the sporophytes (33 %) compared with the progenies (3 %; Fig. 8). The patterns of missing SNPs and the degree of genetic mixing are similar among sporophytes collected from the same patch in all sympatric populations (Fig. 8). The admixed sporophytes/sporelings have genomes mainly biased towards one of the parental species – except sporeling aggregates from patch 2 in population HB2 that displayed haplotypes homozygous for alleles typical for both H. lutescens and H. sericeum together with heterozygotes (Fig. 8). Five sporeling aggregates from this patch showed strong admixture. The failure rate of the SNP loci in these progenies was relatively high among sporeling aggregates, and the maternal sporophytes showed complete failure of SNP genotyping, which was not seen elsewhere, although SNP detection was frequently incomplete in seta samples (Fig. 8).
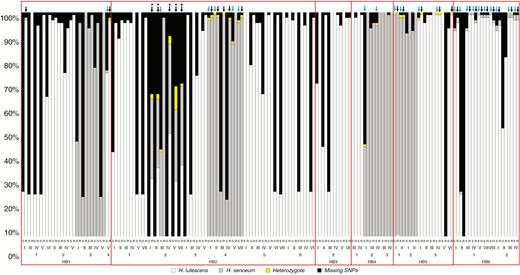
Proportions of SNPs in sporophytes (S) and offspring (R = recombinants; aggregate sample with multiple haploid sporelings; heterozygotic expression is due to occurrence of different alleles from the two parents). All samples were collected from sympatric populations in Öland. The pair of sporophyte and offspring is shown by Roman numerals. Arabic numbers correspond to the patch number of sporophytes taken from additional colonies outside the transect lines (marked by circles in Fig. 1). Hb1–Hb6 = sympatric populations. Blue arrow, admixed sporophyte; black arrow, admixed sporeling sample; *, samples with equal (balanced) representation of the two parental genomes.
Discussion
In this study, we show that SNP markers provide strong evidence for hybridization and introgression between H. lutescens and H. sericeum when gametophytes grow intimately mixed in sympatric populations. DNA samples from all generations, including the data set of maternal gametophytes and their associated sporophytes, and the data set of maternal sporophytes (setae) and their recombinant offspring (aggregate sporelings), showed evidence of gene transfer, probably in both parental directions. Although mildly admixed samples were more abundant, strongly admixed samples occurred in all generations and in most of the sympatric populations.
Pre- and post-fertilization barriers
In bryophytes, fertilization is mediated by male gametes that have to swim from the male sexual structures (antheridia) to the egg cell inside the female archegonium. Estimates of sperm dispersal capacity in moss populations suggest a short dispersal distance, of the order of few decimetres (Bisang et al., 2004) in comparison with spores, which have the potential to be wind dispersed over greater distances (Muños et al., 2004; Frahm, 2008; Rosengren et al., 2014). It is clear from our genotype data that the two species are growing mixed close enough for cross-fertilization to occur between normal-sized males and females within the sympatric populations. However, both species are nannandrous – meaning that they have dwarf males growing on female shoots (Fig. 9) (Hedenäs and Bisang, 2011), while normal-sized males are exceedingly rare, at least in H. lutescens (Rosengren, 2015). In nannandrous species, a close proximity of dwarf males to the female perichaetia is assumed to increase the probability of fertilization (Sagmo Solli et al., 2000; Hedenäs and Bisang, 2011; Rosengren and Cronberg, 2014). A recent spore germination experiment by Rosengren and Cronberg (2015) revealed that male spores of H. sericeum were able to develop into dwarf males on shoots of H. lutescens, suggesting an obvious pathway for cross-fertilization between the two species. In contrast, germination of spores from an unrelated species failed, suggesting that there is a barrier against recruitment of alien dwarf males between more strongly genetically separated lineages than the Homalothecium species.
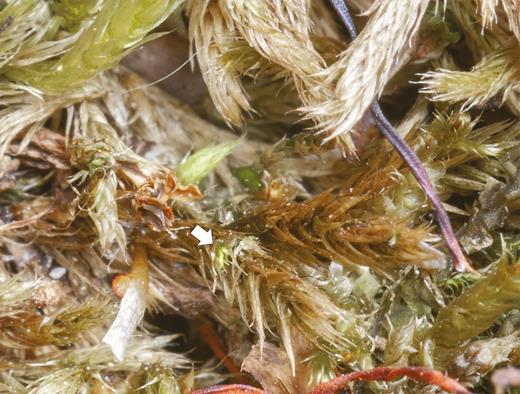
Dwarf male of the moss H. sericeum (white arrow) grows on the normal-sized female shoot.
In the fertilization process, species barriers appear to be weak in bryophytes due to non-specific attraction of male gametes to female archegonia and a general absence of specialized vectors (Anderson and Snider, 1982; Glime and Bisang, 2017). Penetration of archgonia by alien male gametes has been reported in a number of genera of bryophytes such as Mnium (Duckett et al., 1983), Aneura, Sphaerocarpos, Asterella, and Funaria (Showalter, 1926). We do not know how Homalothecium behaves during the fertilization phase but, considering that we find admixed samples, it appears that species barriers are held up rather by post-fertilization processes.
Degree of interspecific mixing and distribution of admixed samples across populations
In order to study the significance of hybridization events, reliable methods for detecting species, hybrids and backcrosses are needed. The ability to detect the direction of interspecific hybridization (and introgression) before the molecular era was based solely on observation of hybrid sporophytes (with intermediate characters) that grew on maternal gametophytes of ‘pure species’ as judged by morphological traits. Sometimes, it is difficult to differentiate between gametophytes of ‘pure species’ and ‘recombinant’ gametophytes on the basis of gross morphology alone, leading to an incorrect interpretation about interspecific hybridization. So far, few cases of gametophytic hybrids have been supported by genetic/molecular data (Glime and Bisang, 2017).
In this study, we used transcriptome data to select SNP markers that were fixed for alternative alleles in the two species of mosses to identify hybridization and admixture. The majority of samples were successfully genotyped, but the failure rate for SNP markers was increased for whole sporophytes and especially for extracts derived from setae (see Supplementary data Table S4; Appendix III). Overall, rare allelic combinations and instances of detection failure were similar or identical in sibling sporophytes attached to the same maternal gametophytes. The failure rate for SNP genotyping could have two different methodological explanations: (1) that the DNA concentration in samples, especially for setae, is close to the detection limit of our analytic method and/or (2) that heterozygotic expression in admixed sporophytes may push both alleles below the detection limit since each SNP allele only represents half the amount of DNA as compared with the two copies of the same allele at a homozygotic locus. It is also possible that genomic incompatibilities or dominance/recessive expression in allele pairs occur in admixed sporophytes. What we can see is that admixed samples sometimes show a relatively high SNP detection failure – the most extreme case being some strongly admixed recombinant progenies for which all SNP markers from parental setae completely failed to be detected. This tendency is also reflected in Fig. 4, in which the distribution along co-ordinate 2 largely displays a combination of diffuse admixture and failure of detection.
All three methods for detecting hybridization (STRUCTURE, PCoA and hybrid index analysis) confirmed genetic admixture in sympatric populations. In total, 17 of 449 samples (3.8 %) were classified as hybrids on the basis of their 111 SNP markers – all of which revealed strongly admixed genomes (in maternal gametophytes, sporophytes and sporelings), possibly primary hybrids or close to primary hybrids, recently formed and representing all sympatric populations in Öland (Fig. 3). The hybrid index revealed 72 (16 %) mildly admixed samples similar to H. lutescens (55 %) or H. sericeum (45 %). Results from our morphological analysis of the hybrid zones (W. Sawangproh et al., unpubl. res.) suggest that some of the samples that do not display admixture of SNPs may also be introgressed. A few mildly admixed sporophytes were also detected from the allopatric populations. Previous studies in the hybrid zones of the copper mosses Mielichhoferia elongata and M. mielichhoferiana showed that recombinant gametophytes, growing in mixed populations, are skewed in the direction of the pure M. mielichhoferiana parental type and that sporophytes appear to belong exclusively to gametophytes of M. elongata (Shaw, 1998), and in the peat mosses Sphagnum capillifolium and S. quinquefarium the progeny of the hybrid sporophytes are mainly mildly admixed samples that skewed in the direction of one parental species (Natcheva and Cronberg, 2007).
Introgression
Introgression is defined as gene flow between populations whose individuals hybridize and then successfully backcross (in the F1 generation) to one or both parental populations (Rhymer and Simberloff, 1996). Beyond F1 hybrids, the point at which an individual is no longer regarded as a hybrid but rather as a member of one of the parental populations that has undergone introgression is arbitrary (Anderson and Hubricht, 1938). A hybrid swarm is a population of individuals in which introgression has occurred to various degrees through varying numbers of generations of backcrossing to one or both parental taxa, in addition to mating among the hybrids themselves. Hybridization is not necessarily accompanied by introgression; for instance, the offspring of hybrid matings might all be sterile (Rhymer and Simberloff, 1996). Earlier studies of bryophyte populations with admixed individuals have not be able to establish whether the admixed individuals are fertile and the populations represent true introgression zones.
It is evident from our study that admixed gametophytes of Homalothecium can produce sporophytes and subsequently viable sporelings that show detectable signals for most of the SNP markers, indicating that they have been successfully transmitted from sporophyte to progeny. Our approach to analyse aggregates of sporelings has the disadvantage that we cannot determine the degree of genomic mixture at the individual level in the progeny – we do not know whether or not the parental contribution to individual sporelings is symmetric. It is also to be noted that admixed samples may be viable in early stages (such as sporelings), especially when cultivated in a protected environment in Petri dishes, but less fit to survive until sexual maturity in wild populations. We can conclude that the sympatric populations represent true introgression zones, with strong indications of interspecific gene transfer through the generations. This is very important since it means that adaptation can take place through transfer of alleles that are immediately exposed to selection in the dominant haploid phase after spore germination. Earlier studies (Shaw, 1998; Cronberg and Natcheva, 2002, Natcheva and Cronberg, 2007) have only been able to demonstrate that recombinants exist in sympatric populations, not that genetic material is transferred beyond the F1 generations.
Hybridization and introgression are not necessarily symmetric in terms of morphological traits or genomic contribution between the two parents. Several such cases of asymmetry have been reported in other species of mosses. For example, the crosses between Weissia controversa and Astomum ludovicianum or the crosses between W. controversa and A. muhlenbergianum (Sw.) Grout in the UK resulted in hybrid sporophytes that could develop on maternal gametophytes of all involved species (Smith, 1978). However, a similar experiment conducted in eastern North America showed that W. controversa acted as the paternal parent in all reported hybrid sporophytes with A. ludovicianum (Reese and Lemmon, 1965) or A. muhlenbergianum (Williams, 1966; Anderson and Lemmon, 1972; Anderson and Snider, 1982). Weissia controversa is known to be polyphyletic (Werner et al., 2005). So, it is not surprising that different accessions from different countries act in different ways.
Although, the SNPs were selected to be species specific, it is clear that some SNPs were more commonly represented in admixed samples, suggesting that gene transfer between species had occurred more readily at certain loci (genes) than at others. It is well documented that gene exchange between two species may not be uniform in space and time, across the genome. Harrison and Larson (2014) emphasize that species boundaries are semi-permeable – meaning that introgression is the result of a selective process, in which alleles at some loci or genomic regions are more likely than others to cross the boundary. It appears that species boundaries in the Homalothecium are semi-permeable and this may prevent complete fusion of the species. However, it should be noted that our markers are selected to be species specific, and that we do not know to what extent other genes are transferred between species. It is also possible that strongly admixed samples are subject to hybrid depression dependent on break up of adaptive gene complexes that are necessary for survival in the typical habitats of either species (reviewed in Todesco et al., 2016).
Paternal analysis
In bryophytes, the F1 hybrid is the short-lived diploid sporophyte, which develops from the maternal archegonium after fertilization and is partially dependent on nutrients from the maternal shoot throughout its development. Being diploid, a hybrid sporophyte contains a complete set of chromosomes from both parental gametophytes, leading to the expectation of heterozygotic expression at all loci with species-specific alleles. The heterozygotic condition was not found across all our species-specific loci in any of the strongly admixed sporophytes. Instead, we observed in strongly admixed sporophytes that the female allele was not expressed or, rather, was suppressed beyond the detection limit so that only the allele specific for the paternal species was detected as a homozygote. In theory, both the maternal and the paternal allele should be present in similar numbers in the sporophyte, and thus be detected equally well in the SNP typing, but it is possible that selective abortion during meiosis and prior to spore formation could reduce the presence of certain alleles.
Another anomaly occurred in several cases where two gametophytes of the same species, one or both with mild admixture, had mated to form a sporophyte with heterozygotes in occasional loci. Some of these heterozygotic loci were associated with SNP detection failure in the maternal parent. In such cases, it was impossible to determine which of the alleles were inherited from the mother or father. Complete failure of SNP detection in the maternal gametophytes is comparatively rare (approx. 4 %), so it seems likely that the missing SNP signal in the female is due to genomic incompatibility related to admixture of an alien allele. In other words, the admixed allele in the heterozygotic loci in the sporophyte is probably derived from the mother rather than the father. The underlying mechanism for expression levels of SNPs below the detection limit in these cases is uncertain since the basic assumption must be that the complete genome, including admixed alleles, is replicated into all vegetative cells in the gametophyte phase of the life cycle. In a study of hybrid fitness (W. Sawangproh and N. Cronberg, unpubl. res.), we could see similar signs of genomic incompatibility, in terms of a strong correlation between failure of SNP detection and reduced germination frequency in progeny from sporophytes. This could possibly be explained by selective abortion of spores with certain combinations of alleles. Nevertheless, it seems that most of the alleles are transmitted to the progeny, since we did not observe any notable difference between the SNP alleles present in the setae and in the corresponding progeny.
Effects of breeding systems
In species with facultative nannandry, such as the Homalothecium species, fertilization is brought about through either normal-sized males or dwarf males which grow as tiny epiphytes on normal-sized females. We know that normal-sized males are exceedingly rare in H. lutescens (Rosengren et al., 2014) and sexual reproduction involving dwarf males is probably the most common for both species. Rosengren and Cronberg (2015) were able to show that spores of H. sericeum applied to female shoots of H. lutescens readily develop into dwarf males, suggesting an obvious route for hybridization. The maternal gametophytes appear to serve as safe sites for germination for both species of Homalothecium where the short-lived (annual to biannual) dwarfs are protected against many of the environmental challenges in the natural substrates.
The dwarf males are recruited by wind-dispersed spores. In general, bryophyte spore dispersal is known to be strongly leptokurtic, with a majority of spores falling in the close vicinity of the female. The two species are markedly different in their spore dispersal strategies, H. sericeum having larger spores (14–26 µm; Hofmann, 1998) that probably disperse less than the small spores of H. lutescens (12–18 µm). This tendency may be increased by the peristome type present in H. sericeum (Zanatta et al., 2018), which is ‘hygrochastic’, meaning that spores are dispersed under relatively humid conditions. This is described as a safe-site strategy for germination, typical for epiphytic species that grow in relatively dry microhabitats, but the dispersal distance in moist air is reduced compared with dispersal under dry conditions, which is more common among mosses. It is not known if H. lutescens also has a hygrochastic peristome, but in general H. lutescens is somewhat less specialized to dry habitats (Rosengren et al., 2014). Differences in spore dispersal are important because short-distance spore movement may increase son–mother fertilizations in a nannandrous system.
Rosengren et al. (2016) presented evidence that son–mother fertilizations are relatively frequent in H. lutescens and that a large fraction of the spores from a sporophyte are likely to be deposited on the maternal gametophyte. Sometimes repeated son–mother fertilization occurs, a form of inbreeding that reduces the genetic variability by a factor 0.5 for each cycle so that the males after a few cycles become identical, or nearly identical, to the female. So there are two alternative explanations for the presence of mildly admixed samples – selective survival of mildly admixed haplotypes at the sporeling stage as a consequence of genomic incompatibilities or loss of paternal alleles through repeated son–mother fertilizations.
Repeated son–mother fertilization could be an explanation for the presence of alien alleles in several allopatric populations. Most of these cases were found in populations of H. sericeum, which were growing on churchyard walls. Several different alleles were involved, but always only detected in the sporophyte generation, which is somewhat hard to explain in outcrossing populations that should not deviate too much from Hardy–Weinberg proportions. A possible explanation for the surplus of heterozygotes in the sporophytes for these loci is related to the presence of dwarf males. Alien alleles could have entered the populations as wind-dispersed spores of the other species forming dwarf males, and then the introgressed genetic material could have been filtered through repeated son–mother fertilization so that eventually only a small part of the H. lutescens genome remains. These alien alleles do not enter the female gametophyte population, presumably because the spores are not viable in the natural habitat.
To summarize, we see that both strongly and mildly genetically admixed individuals occur in all three studied generations – gametophytes, sporophytes and progeny from sporophytes – providing evidence that the studied sympatric population behave as true hybrid zones. The nannandrous breeding system means that dwarf males can be recruited on the alien species and that backcrossing can occur by repeated son–mother fertilization.
Supplementary data
Supplementary data are available online at https://dbpia.nl.go.kr/aob and consist of the following.
Figure S1: frequency of heterozygotes across 111 nuclear SNP markers in the 449 samples.
Table S1: the study sites of parental and putatively hybridizing populations.
Table S2: the localities of allopatric populations of H. lutescens and H. sericeum used for total RNA extraction.
Table S3: frequency of polymorphism of different forms within and between SNPs of gametophyte individuals of H. lutescens and H. sericeum.
Table S4: summary information for plant material from hybridizing populations of H. lutescens and H. sericeum.
Appendix I: UPPMAX scripts. Appendix II: spore germination method. Appendix III: all genotyped SNP markers.
Funding
This study was partially supported by grants from Mahidol University’s Academic Development Scholarship, Elly Olssons fund, Svante Murbecks fund, Ove Almborns fund and The Royal Physiographic Society in Lund.
ACKNOWLEDGEMENTS
We thank the DNA Sequencing Facility at the Department of Biology (Lund University) for supporting the process of the SNP library construction. We would like to thank the Science for Life Laboratory (SciLifeLab) at Uppsala University for the genotyping performed by the SNP&SEQ Technology Platform, and the National Bioinformatics Infrastructure Sweden for bioinformatics support (NBIS).