-
PDF
- Split View
-
Views
-
Cite
Cite
Blake L Cooper, Shatha Salameh, Nikki Gillum Posnack, Comparative cardiotoxicity assessment of bisphenol chemicals and estradiol using human induced pluripotent stem cell-derived cardiomyocytes, Toxicological Sciences, Volume 198, Issue 2, April 2024, Pages 273–287, https://doi.org/10.1093/toxsci/kfae015
- Share Icon Share
Abstract
Bisphenol A (BPA) is commonly used to manufacture consumer and medical-grade plastics. Due to health concerns, BPA substitutes are being incorporated—including bisphenol S (BPS) and bisphenol F (BPF)—without a comprehensive understanding of their toxicological profile. Previous studies suggest that bisphenol chemicals perturb cardiac electrophysiology in a manner that is similar to 17β-estradiol (E2). We aimed to compare the effects of E2 with BPA, BPF, and BPS using human induced pluripotent stem cell-derived cardiomyocytes (hiPSC-CM). Cardiac parameters were evaluated using microelectrode array (MEA) technology and live-cell fluorescent imaging. Cardiac metrics remained relatively stable after exposure to nanomolar concentrations (1–1000 nM) of E2, BPA, BPF, or BPS. At higher micromolar concentrations, chemical exposures decreased the depolarization spike amplitude, and shortened the field potential, action potential duration, and calcium transient duration (E2 ≥ BPA ≥ BPF ≫ BPS). Cardiomyocyte physiology was largely undisturbed by BPS. BPA-induced effects were exaggerated when coadministered with an L-type calcium channel (LTCC) antagonist or E2, and reduced when coadministered with an LTCC agonist or an estrogen receptor alpha antagonist. E2-induced effects were not exaggerated by coadministration with an LTCC antagonist. Although the observed cardiac effects of E2 and BPA were similar, a few distinct differences suggest that these chemicals may act (in part) through different mechanisms. hiPSC-CM are a useful model for screening cardiotoxic chemicals, nevertheless, the described findings should be validated using a more complex ex vivo and/or in vivo model.
Bisphenol A (BPA) is a high production volume chemical that is widely used in the manufacturing of epoxy resins and consumer and medical-grade polycarbonate plastics the incidence of arrhythmias in a manner comparable with BPA (Duty et al., 2013; Iribarne-Durán et al., 2019; Vandenberg et al., 2007). Given its relative abundance in the environment, biomonitoring studies indicate that >90% of the U.S. population is routinely exposed to BPA (Calafat et al., 2005, 2008; Lehmler et al., 2018; Vandenberg et al., 2010; Ye et al., 2015). Of concern, heightened BPA exposure is associated with adverse cardiovascular outcomes (Lind et al., 2021; Ramadan et al., 2020), including an increased risk of hypertension, atherosclerosis, coronary heart disease, and depressed heart rate variability (Bae et al., 2012; Bae and Hong, 2015; Melzer et al., 2010; Moon et al., 2021; Shankar and Teppala, 2012). Further, longitudinal cohort studies performed in the United States suggest that individuals with elevated BPA exposure have an approximately 40% higher risk of cardiovascular and all-cause mortality (Bao et al., 2020; Chen et al., 2023). These health associations have prompted a renewed interest in understanding the link between bisphenol chemical exposure and cardiovascular toxicity.
To date, experimental studies suggest that the biological effects of BPA may be mediated through estrogen receptor signaling and/or inhibition of cardiac ion channels (Cooper and Posnack, 2022). Multiple groups have demonstrated that BPA rapidly and reversibly blocks voltage-gated sodium and calcium channels in a dose-dependent manner (Deutschmann et al., 2013; Feiteiro et al., 2018; Hyun et al., 2021; Liang et al., 2014; Michaela et al., 2014; O’Reilly et al., 2012; Prudencio et al., 2021; Wang et al., 2011). In cardiac tissue, sodium channel blockers slow depolarization and conduction velocity—whereas calcium channel blockers reduce heart rate and slow atrioventricular conduction. Calcium flux also plays a vital role in cardiomyocyte excitation-contraction coupling, which is also disrupted by BPA exposure (Hyun et al., 2021; Posnack et al., 2015; Ramadan et al., 2018; Yan et al., 2011). A few studies have also demonstrated that BPA modulates intracellular calcium handling through estrogen receptor signaling—akin to 17β-estradiol (Belcher et al., 2011; Yan et al., 2013, 2011). In vitro studies report an immediate decrease in peak calcium and potassium current upon exposure to 17β-estradiol (E2), the predominant circulating estrogen in women (Berger et al., 1997; Jiang et al., 1992; Meyer et al., 1998).
Over time, BPA-related health concerns have shifted consumer preference for non-BPA products and influenced stricter manufacturing regulations, which in turn, has led to the introduction of new replacement chemicals—many of which structurally resemble BPA (Lehmler et al., 2018; Yu et al., 2015). Unfortunately, the safety profile of these alternative chemicals remains unclear, with some studies suggesting that these “regrettable substitutions” have comparable biological effects to BPA (Delfosse et al., 2012; Kojima et al., 2019; Trasande, 2017; Wang et al., 2022). For example, 1 study found that bisphenol S (BPS) altered intracellular calcium signaling and increased (Duty et al., 2013; Iribarne-Durán et al., 2019; Vandenberg et al., 2007) the incidence of arrhythmias in a manner comparable with BPA (Gao et al., 2015). Although another study reported that BPS is a less potent antagonist of L-type calcium channels (LTCCs) with negligible effects on cardiac electrophysiology (Prudencio et al., 2021), which could be attributed to slight differences in its chemical structure (Deutschmann et al., 2013).
In the presented study, we aimed to evaluate the direct effects of BPA on cardiac electrophysiology and compare its potency to both E2 and alternative bisphenol chemicals (eg, BPS and BPF). Based on prior work identifying BPA as a potent inhibitor of LTCCs, we anticipated that BPA would perturb cardiac electrophysiology and calcium handling to a greater extent than BPS. To date, bisphenol toxicology studies have largely been limited to rodent model systems, which requires the careful consideration of species-specific differences when predicting human risk (Edwards and Louch, 2017; Ripplinger et al., 2022). With this in mind, we evaluated the cardiotoxicity of bisphenol chemicals using human induced pluripotent stem cell-derived cardiomyocytes (hiPSC-CM). hiPSC-CM are considered a promising “new approach methodology” for cardiac risk assessment based on their similarity to human cardiomyocyte ion channel expression, action potential morphology, and contractile machinery (Chen et al., 2016; Gintant et al., 2017; Parish et al., 2020; Sinnecker et al., 2013).
Materials and methods
Reagents
A list of all chemical reagents is provided in Supplementary Table 1. Stock solutions were prepared in >99.9% anhydrous dimethyl sulfoxide (DMSO) and working concentrations were diluted in cell culture media (iCell cardiomyocyte maintenance media for all MEA experiments or Tyrode’s salt solution for all imaging experiments). Treatment groups included: 0.01–100 μM E2, BPA, BPF, or BPS; 10 nM Bay K8644 (BayK); 100 nM verapamil; 10 μM 4,4′,4″-(4-propyl-[1H]-pyrazole-1,3,5-triyl)trisphenol (PPT); 10 μM diarylpropionitrile (DPN); 1 μM ICI 182 780 (ICI); 1 μM 1,3-bis(4-hydroxyphenyl)-4-methyl-5-[4-(2-piperidinylethoxy)phenol]-1H-pyrazole dihydrochloride (MPP); 1 μM 4-[2-phenyl-5,7-bis(trifluoromethyl)pyrazolo[1,5-a]pyrimidin-3-yl]phenol (PHTPP); 1 μM progesterone. The range of bisphenol concentrations was selected to mimic environmental exposure (1–100 nM), maximum clinical or occupational exposure (1–10 μM), and supraphysiological exposure levels (>10 μM) (Bousoumah et al., 2021; Ramadan et al., 2020). Pharmacological agent concentrations were selected based on previous reports (Hamilton et al., 1987; Long et al., 1998; Paterni et al., 2014; Zhou et al., 2009) and preliminary potency experiments. For drug combinations, hiPSC-CM were pretreated with a pharmacological agent (15–20 min), then BPA or E2 was added (15–20 min) before recording electrical signals. DMSO was used as a vehicle control (0.1%). To reduce the risk of environmental contamination, nonpolycarbonate bisphenol-free plastic consumables were used for experiments (including polystyrene cell culture plates from Axion BioSystems).
hiPSC-CM preparation
hiPSC-CM (iCell cardiomyocytes2, donor no. 01434, Fujifilm Cellular Dynamics) were thawed and plated on fibronectin-coated glass-bottom dishes (100 000 cells/cm2) or microelectrode array (MEA) plates (40 000 cells/well; 24-well plate, Axion Biosystems), as described previously (Cooper et al., 2021; Prudencio et al., 2021). In total, 14 individual batches of cells (from thaw) were used in these experiments (lot no.: 105455, 105998, 106049). Female donor hiPSC-CM were used, as prior studies suggest that bisphenol toxicity may be exaggerated in female cells (Belcher et al., 2011; Gao et al., 2015). Cells were maintained under standard cell culture conditions (37°C, 5% CO2) in iCell cardiomyocyte maintenance media (Fujifilm Cellular Dynamics) supplemented with 1 mg/ml ciprofloxacin (Sigma Aldrich). Four days after plating, hiPSC-CM formed a confluent, spontaneously beating monolayer and cell monolayers were subsequently used for ongoing experiments.
Microelectrode array recordings
MEA plates were equilibrated for 15–20 min in a temperature-controlled (37°C) cell monitoring system (Maestro Edge system, Axion Biosystems); electrical signals were recorded at baseline and after chemical treatment (Figs. 1A–M). Electrical signals were recorded during spontaneous rhythm and in response to external stimulation via a single embedded pacing electrode (1–2 Hz, 400–600 µs stimulus duration, 800–900 mV, 20–30 µA; 12.5 kHz sampling frequency). Cardiac parameters included: propagation consistency (percent of electrodes with a consistent propagation pattern), spontaneous beating rate, spike amplitude (an indicator of depolarization, akin to an electrocardiogram QRS complex), conduction velocity, extracellular field potential duration rate-corrected with the Fridericia formula (FPDc; an indicator of repolarization, akin to an electrocardiogram QT interval), local extracellular action potential duration (APD; akin to an electrocardiogram QT interval, measured by way of enhanced cardiomyocyte-electrode coupling), beat amplitude (an indicator of contractility, measured by a change in impedance across electrodes), and excitation-contraction delay (time between depolarization spike and contractility waveform peak), as described previously (Abassi et al., 2012; Clements, 2016). Each dataset was manually reviewed (B.C., S.S.) to select 1 electrode per well with the largest signal amplitude (eg, action potential or t-wave). Waveforms from the selected electrode were averaged (20 s). Individual wells were omitted if the electrical signal had an insufficient signal-to-noise ratio (preventing accurate T-wave identification) or if hiPSC-CM were not pacing at the desired frequency. Exclusion criteria were established for each parameter based on the measurement range at baseline (ie, 24–64 bpm, 330–470 ms FPDc). To account for slight differences between individual plates or hiPSC-CM lot variability, vehicle controls (DMSO) were included and recorded on each MEA plate.
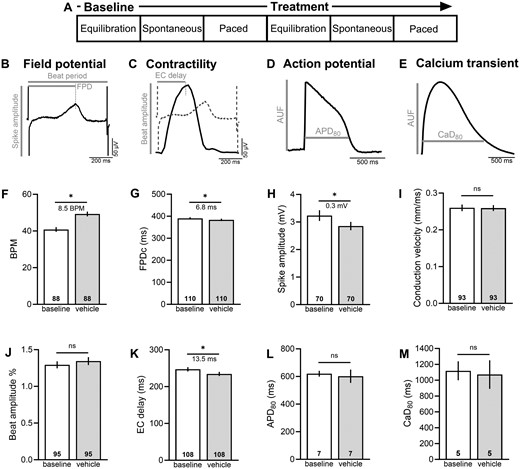
Experimental protocol. (A) hiPSC-CM were equilibrated (15–20 min), and then basal cardiac metrics were measured during spontaneous beating or in response to external pacing. Thereafter, hiPSC-CM were treated with a specified chemical for 15–20 min, and then cardiac metrics were measured again. Representative traces and fiducial markers are shown for (B) field potential recording, (C) contractility, (D) optical action potential, and (E) intracellular calcium transient. (F–K) MEA parameters were compared between baseline and 0.1% DMSO (vehicle) to establish threshold values for chemical treatment studies. (L, M) Action potentials and calcium transients were also compared between baseline and 0.1% DMSO (optical recordings were performed at room temperature). Number of independent replicates reported in each bar. Mean and 95% confidence interval are reported; t test with *p < .05. BPM recorded during spontaneous rhythm, all other parameters reported in response to pacing. APD80, action potential duration at 80% repolarization; AUF, arbitrary unit of fluorescence; BPM, beats per minute; CaD80, calcium transient duration at 80% recovery; EC delay, excitation-contraction delay time; FPDc, field potential duration, rate corrected.
Fluorescent imaging
Separately, hiPSC-CM were loaded with a calcium indicator dye (10 μM Fluo 4-AM, Thermo Fisher Scientific) to evaluate calcium handling metrics or a potentiometric dye (1:1000 FluoVolt, Thermo Fisher Scientific) to confirm action potential measurements observed in MEA experiments. Cell monolayers were incubated with dye for 20 min at room temperature (22°C), then washed in dye-free Tyrode’s salt solution (Sigma-Aldrich; g/l: 0.2 CaCl2, 0.1 MgCl2, 0.2 KCl, 8.0 NaCl, 0.05 NaH2PO4, 1.0 d-glucose, 1.0 NaHCO3), as described previously (Cooper et al., 2021). Cell monolayers were maintained in Tyrode’s solution for all imaging experiments. Pace-induced calcium transients and action potential recordings were acquired using a Nikon TiE microscope system, equipped with 470 nm excitation LED (SpectraX, Lumencor), 505–530 nm emission filter, and Photometrix 95B sCMOS back-illuminated camera. Cardiac monolayers were paced using field stimulation (1.5× threshold voltage, 0.2–1 Hz). Optical signals were analyzed using custom software (Cooper et al., 2021; Jaimes et al., 2016). Cardiac parameters included: calcium transient activation time, duration time (CaD30 or CaD80; activation to 30% or 80% recovery), Tau (decay time constant), and APD time (APD30 or APD80; activation to 30% or 80% repolarization time). To account for slight differences between cardiomyocyte preparations, optical recordings were performed at baseline and again after chemical treatment on the same monolayer of cells.
Statistical analysis
Results were reported as the mean (95% confidence interval lower bound, upper bound), unless otherwise indicated. Statistical analysis was performed using either a Student’s t test (2 groups) or 1-way analysis of variance (ANOVA) with Holm-Sidak multiple comparison test (3 or more groups), as indicated in each figure legend. An adjusted p value < .05 was considered statistically significant. Replicates are defined in each figure.
Results
Experimental protocol
We first measured variations in hiPSC-CM physiology under baseline conditions and following exposure to 0.1% DMSO—which was used as a vehicle in subsequent bisphenol studies (Figs. 1F–M). Application of DMSO had no effect on cardiomyocyte conduction velocity (baseline: 0.26 [0.25, 0.27], vehicle: 0.259 [0.25, 0.27] mm/ms) or beat amplitude (baseline: 1.29 [1.25, 1.34], vehicle: 1.34 [1.29, 1.40] %). Supplementing cell culture media with 0.1% DMSO slightly shifted the spontaneous beating rate (baseline: 40.8 [39.5, 42.1], vehicle: 49.3 [48.0, 50.6] BPM, p < .0001), FPDc (baseline: 390.4 [385.1, 395.6], vehicle: 383.6 [378.3, 388.8] ms, p < .001), depolarization spike amplitude (baseline: 3.24 [3.04, 3.43], vehicle: 2.85 [2.70, 3.00] mV, p < .0001), and excitation-contraction delay (baseline: 247.0 [241.2, 252.9], vehicle: 234.5 [228.8, 240.1] ms, p < .001). To take these variations into account, MEA measurements were compared between DMSO and bisphenol chemical exposure. The application of DMSO did not have an effect on optical measurements of APD (APD80 baseline: 620 [599, 641], vehicle: 602 [554, 650] ms) or calcium transient duration (CaD80 baseline: 1119 [999, 1238], vehicle: 1071 [891, 1251] ms)—therefore, measurements were compared between baseline and chemical exposure.
Acute effects of E2 and BPA on cardiac electrophysiology
For toxicity studies, we first identified the timeframe in which BPA or E2 alter cardiac electrophysiology (Figure 2). Previous studies report rapid effects of BPA, with no discernable effect on cardiomyocyte viability up to 100 μM (Hyun et al., 2021; Posnack et al., 2014; Ramadan et al., 2018). hiPSC-CM spontaneous beating rate remained relatively stable after exposure to low doses of BPA (1–100 nM), whereas 30 μM BPA transiently increased the beating rate (20 min vehicle: 62.0 [57.1, 66.9], BPA: 77.1 [72.9, 81.4] BPM, p < .0001) with effects waning over time (Figure 2A). Similarly, 30 μM E2 rapidly increased the beating rate within 5 min, but thereafter all spontaneous activity ceased until approximately 24 h later (Figure 2B). We also noted an immediate effect of BPA and E2 on the FPDc, which had a nonlinear dose-response. At lower doses, FPDc was slightly longer following acute BPA (20 min vehicle: 328.3 [319.9, 336.7], 100 nM: 342.0 [332.6, 351.4] ms, p < .05) or E2 treatment (20 min 1 nM: 341.2 [333.9, 348.5], 100 nM: 338.9 [331.8, 345.9] ms, p < .05; Figs. 2C and 2D). Although at higher doses, FPDc shortened with acute BPA (20 min 30 μM: 300.3 [294.3, 306.3] ms, p < .0001) or E2 treatment (30 μM: 273.4 [266.3, 280.6] ms, p < .0001). Notably, these effects were reversible after the removal of BPA- or E2-supplemented media (data not shown). Others have reported arrhythmias (triggered activity) in primary cardiomyocytes after BPA exposure (Belcher et al., 2011; Yan et al., 2011), but we did not observe rhythm or propagation irregularities in BPA-exposed hiPSC-CM (Figure 2E). However, acute 30 μM E2 treatment significantly reduced the propagation consistency (20 min vehicle: 94.3 [89.3, 99.3], 30 μM: 52.1 [38.4, 65.8] %, p < .0001).
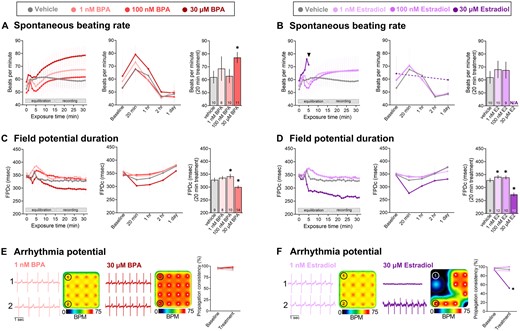
Time-dependent effects of BPA and E2. A and B, Spontaneous beating rate following BPA or E2 exposure. ANOVA with multiple comparisons test, *p < .05 compared with vehicle (0.1% DMSO). C and D, Field potential duration (rate-corrected with Fridericia formula) following BPA or E2 exposure. ANOVA with Holm-Sidak multiple comparisons test, *p < .05 compared with vehicle (0.1% DMSO). E and F, Arrhythmia potential as determined by propagation consistency across the microelectrode array. Raw signals from 2 individual electrodes are shown, as well as a heatmap of the spontaneous beating rate across the 16-electrode array. Paired t test, *p < .05 compared with baseline recording on same electrode. Mean and 95% confidence interval are reported. BPM recorded during spontaneous beating, FPDc recorded in response to 1.5 Hz pacing frequency. N/A: Not assessed due to loss of spontaneous beating activity within this single lot of hiPSC-CMs.
Dose-dependent effects of E2 and bisphenol chemicals on hiPSC-CM electrophysiology
After confirming that the cardiac effects of E2 and BPA occur within an acute timeframe (∼20 min), we next sought to compare the potency of bisphenol chemicals. The spontaneous beating rate was inconsistent between treatment groups, with loss of activity observed upon exposure to higher concentrations of E2, BPA, and BPF—but not BPS (Figure 3). As such, cardiac parameters were assessed in response to external pacing to account for rate-dependent adaptations.

Effect of E2 and bisphenol chemicals on hiPSC-CM spontaneous beating. hiPSC-CM spontaneous beating activity slowed after exposure to higher concentrations of (A) E2, (B) BPA, (C) BPF, but not BPS (D). Values reported as the percentage of cardiomyocytes with spontaneous activity, as recorded from cell monolayers plated atop microelectrode arrays. n = 11–40 individual cardiomyocyte preparations per dose, per treatment.
Because BPA inhibits voltage-gated Na+ channels through interaction with the anesthetic receptor site (O’Reilly et al., 2012; Prudencio et al., 2021), we examined the direct effect of E2 and bisphenol chemicals on the depolarization spike amplitude which corresponds to Na+ influx (Figure 4). Spike amplitude remained unchanged at lower concentrations of E2 and BPA, but decreased at higher micromolar concentrations (≥10 μM; Figs. 4A–C). Compared with vehicle control, E2 and BPA reduced the spike amplitude by 14%–17.8% at 30 μM (vehicle: 2.88 [2.73, 3.02], E2: 2.35 [1.95, 2.74], BPA: 2.45 [2.23, 2.68] mV) and by 85.3%–87.1% at 60 μM (E2: 0.42 [0.33, 0.51], BPA: 0.37 [0.33, 0.41] mV; Figs. 4B and 4C). This aligns with the reported half-maximal inhibitory concentration (23–56 μM) for BPA on sodium channel current (Hyun et al., 2021; O’Reilly et al., 2012; Prudencio et al., 2021). Comparatively, BPF and BPS exposure had a less pronounced effect on the depolarization spike amplitude. Next, we measured conduction velocity across the MEA—as a reduced spike amplitude is expected to delay electrical propagation within a cardiomyocyte monolayer. Conduction velocity slowed after acute exposure to E2 or BPA, with less pronounced effects observed with BPF or BPS exposure (Figs. 4D and 4E). To account for any slight differences between hiPSC-CM experiments due to lot variability or plating density, both raw (Figs. 4B and 4D) and percent change data are reported (Figs. 4C and 4E)—wherein the latter accounts for differences in baseline measurements before applying a chemical treatment.
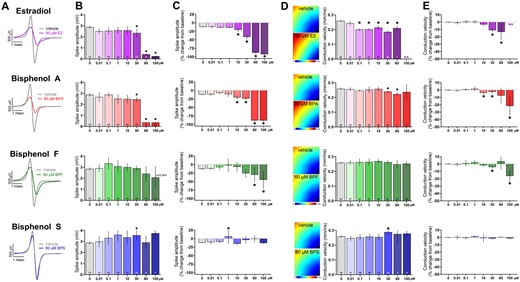
Effect of E2 and bisphenol chemicals on depolarizing spike amplitude and conduction velocity. A, Representative traces of depolarizing spike recorded from hiPSC-CM, paced at 1.5 Hz, following acute (15 min) exposure to 60 μM E2, BPA, BPF, or BPS. Note: The y-axis is truncated in order to view the small depolarizing spike resulting from E2 or BPA exposure. B, Spike amplitude, chemical dose response. C, Percent change in spike amplitude after chemical exposure, relative to matched baseline recording. D, left: Activation maps recorded from a monolayer of hiPSC-CM, paced at 1.5 Hz, following acute (15 min) exposure to 60 μM E2, BPA, BPF, or BPS. Activation is initiated at the pacing electrode in lower right corner, as indicated. Right: Conduction velocity, chemical dose response. E, Percent change in conduction velocity after chemical exposure, relative to matched baseline recording. Number of independent replicates reported in each bar. Mean and 95% confidence interval are reported; ANOVA with multiple comparisons test, *p < .05 relative to vehicle control. N/A: not assessed due to loss of activity. Conduction velocity was only measured on hiPSC-CM with consistent electrical propagation.
Previous studies demonstrated that BPA shortens the extracellular FPD (analogous to the electrocardiogram QT interval), which may be related to its inhibitory effect on voltage-gated Ca2+ channels (Hyun et al., 2021; Prudencio et al., 2021). At a pacing frequency of 1.5 Hz, the rate-corrected FPD shortened upon exposure to higher concentrations of E2, BPA, and BPF (Figure 5). As an example, compared with vehicle control, the FPDc shortened by 11.8%–18% at 30 μM dose (vehicle: 383.6 [378.3, 388.8], E2: 314.3 [307.5, 321.1], BPA: 333.7 [329.2, 338.2], BPF: 338.3 [329.8, 346.8] ms; Figs. 5A–C). In comparison, BPS exposure had no measurable effect on FPDc at any of the tested concentrations (0.01–100 μM). Both the raw (Figs. 5B and 5D) and percent change FPDc data are reported (Figure 5C) to account for any slight differences in hiPSC-CM phenotype between experiments. In a subset of studies, we also tested the electrical restitution properties of hiPSC-CM by incrementally increasing the pacing frequency to assess rate-dependent adaptations. In control preparations, the FPDc shortened by 14.3%–16.2% as the pacing frequency increased from 1 to 2 Hz, with a clearly defined negative slope (Figure 5D). This rate-dependent adaptation was maintained in the presence of BPS (0.1–60 μM), but exposure to elevated concentrations of E2, BPA, or BPF resulted in a flattened electrical restitution curve. As an example, vehicle control samples had a restitution slope of −57 [−71, −44] to −71 [−81, −63] across all replicates, which flattened significantly compared with 30 μM E2 (−1 [−14, 12] slope), BPA (−31 [−43, −19] slope), and BPF (−51 [−71, −31] slope; Figure 5D). FPDc measurements were inconsistent at 100 μM E2 and BPA, as hiPSC-CM became unresponsive to electrical stimulation.
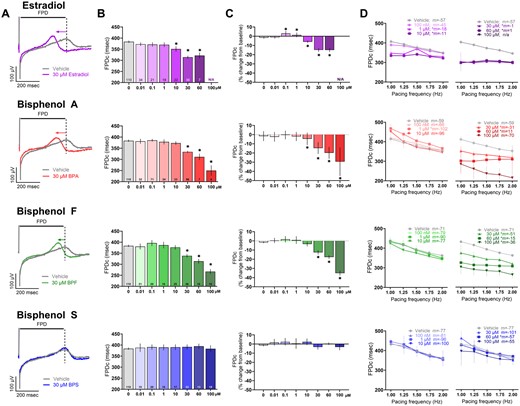
Effect of E2 and bisphenol chemicals on extracellular field potential duration. A, Representative extracellular field potential traces recorded from hiPSC-CM, paced at 1.5 Hz, following acute (15 min) exposure to 30 μM E2, BPA, BPF, or BPS. Note: The y-axis is truncated in order to zoom in on the T-wave (dotted line). B, Field potential duration, rate-corrected (FPDc), chemical dose response. C, Percent change in FPDc after chemical exposure, relative to matched baseline recording. D, Electrical restitution (left: lower concentrations, right: higher concentrations; vehicle data duplicated) with pacing rate increased stepwise from 1 to 2 Hz; slope (“m”) is indicated for each chemical. Number of independent replicates reported in each bar. Mean and 95% confidence interval are reported; ANOVA with multiple comparisons test, *p < .05 relative to vehicle control. N/A: Not assessed due to loss of activity. Samples omitted if not responsive to the desired pacing frequency.
In a subset of studies, hiPSC-CM were electrically induced to increase cell-to-electrode coupling to record local extracellular action potentials (Hayes et al., 2019). The dose response patterns were similar to FPD results, wherein the APD shortened following exposure to increasing concentrations of E2, BPA, and BPF. As an example, compared with vehicle control, APD90 shortened by 7.9%–20.2% at 30 μM dose (vehicle: 396.7 [388.1, 405.3], E2: 316.7 [309.8, 323.6], BPA: 365.3 [352.6, 325.3], BPF: 360.3 [345.4, 375.2] ms; 1.5 Hz pacing frequency; Figs. 6A and 6B). Comparatively, BPS had no effect on APD90 at any of the tested concentrations (0.01–100 μM). We also tested rate-dependent adaptations, wherein APD90 shortened by 11.4% (−101 [−128, −75] slope) in control cell preparations as the pacing frequency increased from 1 to 2 Hz (Figure 6C). Conversely, higher concentrations of E2, BPA, and BPF produced a flattened electrical restitution curve compared with the control—whereas exposure to lower doses of BPS (0.01–10 μM) resulted in a slightly steeper curve. We also analyzed optical action potentials using a voltage-sensitive dye, which yielded similar results (Figure 6D, 0.5 Hz frequency). APD80 shortening was observed after exposure to E2 (baseline: 867 [791, 943], 30 μM E2: 566 [484, 649] ms), BPA (baseline: 783 [680, 886], 30 μM BPA: 568 [510, 626] ms), and BPF (baseline: 862 [763, 960], 30 μM BPF: 688 [637, 738] ms)—but not BPS (baseline: 757 [639, 876], 30 μM BPS: 742 [627, 856] ms).
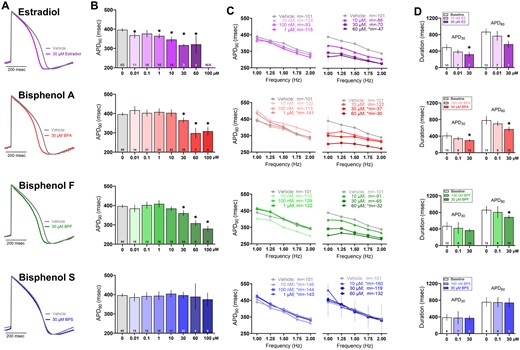
Effect of E2 and bisphenol chemicals on cardiac action potential duration. A, Representative action potential traces recorded from hiPSC-CM following 15 min exposure to 30 μM E2, BPA, BPF, or BPS (1.5 Hz pacing frequency, 37°C). B, Local extracellular action potential duration at 90% repolarization (APD90), dose response. C, Electrical restitution (left: lower concentrations, right: higher concentrations; vehicle data duplicated) with pacing rate increased stepwise from 1 to 2 Hz; slope “m” is indicated for each chemical. D, Action potential duration at 30% and 80% repolarization, measured optically using a voltage-sensitive dye (0.5 Hz pacing frequency, room temperature). Number of independent replicates reported in each bar. Mean and 95% confidence interval are reported; ANOVA with multiple comparisons test, *p < .05 relative to vehicle control (A–C) or baseline measurement preceding chemical exposure (D). N/A: Not assessed due to loss of activity. Samples omitted if not responsive to the desired pacing frequency or if optical signals were of insufficient quality for analysis.
Dose-dependent effects of E2 and bisphenol chemicals on calcium handling and contractility
Prior studies suggest that E2 and BPA interfere with intracellular calcium transients, which may be a consequence of LTCC inhibition and/or the phosphorylation of key regulatory proteins (Cooper and Posnack, 2022; Gao et al., 2013; Hyun et al., 2021; Prudencio et al., 2021). To evaluate such effects, we recorded calcium transients from hiPSC-CM using an indicator dye (Fluo4; Figure 7A). All treated samples showed rate-dependent adaptations, wherein the calcium transient duration (CaD) shortened as the pacing frequency was increased (0.2–1 Hz)—but to varying degrees. Rate-dependent shortening of CaD80 was slightly blunted with a flattened curve observed after exposure to E2 (baseline: 48.9%, 30 μM E2: 42.7% shortening)—but a greater change upon exposure to BPA (baseline: 46.1%, 30 μM BPA: 35.5% shortening), BPF (baseline: 42.5%, 30 μM BPF: 33% shortening), or BPS (baseline: 48.4%, 30 μM BPS: 37.2% shortening; Figure 7B).
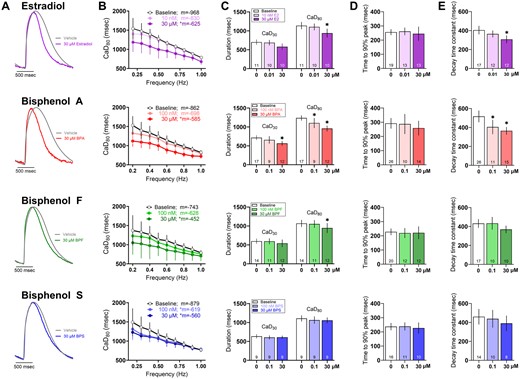
Effect of E2 and bisphenol chemicals on intracellular calcium transients. A, Representative calcium transients recorded from hiPSC-CM following 15 min exposure to 30 μM E2, BPA, BPF, or BPS. Calcium transients were measured optically using a calcium-indicator dye (0.5 Hz pacing frequency, room temperature). B, Calcium transient duration at 80% (CaD80) as the pacing rate was increased stepwise from 0.2 to 1 Hz; slope “m” is indicated for each chemical. C, CaD30 and CaD80. (D) Time to 90% peak fluorescence. (E) Decay time constant all measured at 0.5 Hz frequency. Number of independent replicates reported in each bar. Mean and 95% confidence interval are reported; ANOVA with multiple comparisons test, *p < .05 relative to baseline measurements preceding chemical treatment. Samples omitted if not responsive to the desired pacing frequency or if optical signals were of insufficient quality for analysis.
At an intermediate pacing frequency (0.5 Hz), the calcium transient duration at 80% recovery (CaD80) was shorter after exposure to 30 μM E2 (baseline: 1137 [1042, 1231], E2: 938.6 [821.6, 1056] ms), BPA (baseline: 1237 [1167, 1306], BPA: 958.3 [881.8, 1035] ms), and BPF (baseline: 1068 [974.4, 1161], BPF: 945.6 [815.4, 1078] ms)—but BPS had no measurable effect on CaD80 (Figure 7C). The timing of calcium-induced calcium release did not appear to be altered, as assessed by the time to reach 90% of the calcium transient peak (measured via fluorescence, Figure 7D). Instead, the effects on calcium handling were related to the recovery phase (assessed by the decay time constant, Figure 7E) reflecting the time required for calcium sequestration into the sarcoplasmic reticulum or extrusion from the cell. CaD80 shortening may be caused by increased phospholamban phosphorylation which enhances sarcoplasmic reticulum calcium-ATPase activity (Gao et al., 2013) and/or less inward calcium current which diminishes calcium release from the sarcoplasmic reticulum and shortens the calcium transient amplitude (Prudencio et al., 2021; Ramadan et al., 2018). Such differences in calcium handling were rate-dependent and masked at faster pacing frequencies.
Next, we assessed the impact of chemical exposure on the magnitude and timing of cardiomyocyte contraction. We anticipated that perturbations in calcium handling would reduce contractility in BPA-treated hiPSC-CM, as reported previously in rodent heart and atrial preparations (Pant et al., 2011; Posnack et al., 2015). Indeed, the contractile beat amplitude decreased following exposure to increasing concentrations of E2 (baseline: 1.32% [1.29, 1.34], 60 μM: 0.56% [0.54, 0.57], 100 μM: 0.53% [0.50, 0.55]), BPA (30 μM: 1.15% [1.07, 1.23], 60 μM: 0.65% [0.61, 0.69], 100 μM: 0.57% [0.52, 0.61]), or BPF (100 μM: 0.97% [0.71, 1.22])—whereas BPS had no effect on contractility (Figs. 8A–C). We also measured the coupling time between the electrical and contractile signal and noted a significant 46%–61% longer coupling time after exposure to 60–100 μM E2 or BPA (Figs. 8D and 8E). Excitation-contraction coupling time was unaffected by either BPF or BPS. Although these metrics may be linked to LTCC inhibition, concomitant inhibitory effects on the fast voltage-gated sodium channel may also play a role. Indeed, a reduction in inward sodium current will alter the activity of the sodium-calcium exchanger, which would also impact calcium influx and excitation contraction coupling (Bers and Despa, 2009; Eisner et al., 2017).
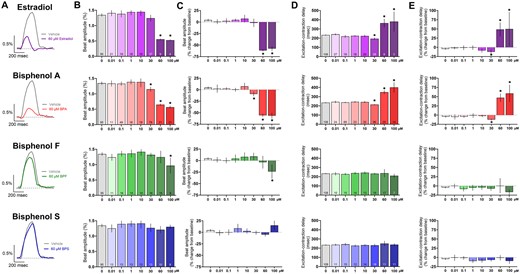
Effect of E2 and bisphenol chemicals on cardiomyocyte contraction. A, Representative recordings of hiPSC contraction and relaxation (impedance-based measurement) following 15 min exposure to 60 μM E2, BPA, BPF, or BPS (1.5 Hz pacing frequency). B, Beat amplitude, dose response. C, Percent change in beat amplitude after chemical exposure, relative to matched baseline recording. D, Excitation-contraction coupling time, dose response. E, Percent change in excitation-contraction coupling time, relative to matched baseline recording. Number of independent replicates reported in each bar. Mean and 95% confidence interval are reported; ANOVA with multiple comparisons test, *p < .05 relative to vehicle control (0.1% DMSO). Samples omitted if not responsive to the desired pacing frequency or if the contraction amplitude was too small to measure accurately.
BPA-induced effects are exaggerated by coadministration with E2 or calcium channel antagonist
Several studies have suggested that BPA cardiotoxicity is related to its interaction with estrogen receptors and/or the inhibition of voltage-gated calcium channels (Belcher et al., 2011; Deutschmann et al., 2013; Feiteiro et al., 2018; Michaela et al., 2014; Prudencio et al., 2021; Yan et al., 2011). Accordingly, we utilized pharmacological tools to assess the cellular targets of E2 and BPA, and to determine whether these agents exert an additive effect. For these proof-of-concept studies, a single concentration of E2 and BPA was used (30 μM), which was efficacious in our prior results and aligns with the half-maximal inhibitory concentration of BPA on voltage-gated calcium channels (Hyun et al., 2021; Prudencio et al., 2021). In this subset of experiments, 30 μM BPA and 30 μM E2 increased the spontaneous beating rate to a similar degree (+25.6% and +35.7%, respectively; Figure 9). Next, we evaluated whether this effect could be attenuated by pretreating hiPSC-CM with an LTCC agonist (Bay K8644), which effectively reduced the effects of BPA and E2 on beating rate (Figs. 9A and 9B). Pretreatment with an LTCC antagonist (verapamil) exaggerated the effect of BPA on beating rate, but not E2—suggesting a different mechanism of action.
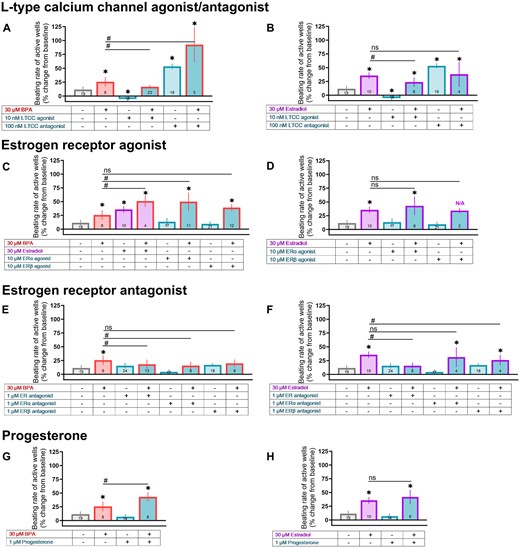
BPA-induced changes on beating rate are exaggerated by coadministration with E2 or calcium channel antagonist. hiPSC-CM were pretreated with a pharmacological agent (15 min) followed by exposure to either 30 μM BPA or 30 μM E2. A and B, Pretreatment with an LTCC agonist (Bay K8644) or antagonist (verapamil). C and D, Pretreatment with at ERα agonist (PPT) or ERβ agonist (DPN). E and F, Pretreatment with a nonspecific ER antagonist (ICI 182 780), ERα antagonist (MPP), or ERβ antagonist (PHTPP). G and H, Pretreatment with progesterone. Vehicle and pharmacological agent data repeated across graphs for clarity. Number of independent replicates reported in each bar. Values reported as a percent change from baseline measurement, immediately preceding chemical treatment (mean and 95% confidence interval). ANOVA with multiple comparisons test, *p < .05 relative to vehicle control (0.1% DMSO), #p < .05 relative to either 30 μM BPA alone or 30 μM E2 alone. Samples omitted if spontaneous beating activity ceased. N/A: Not applicable due to loss of spontaneous activity across multiple samples, which prevented statistical analysis.
Studies also suggest that BPA cardiotoxicity is amplified by E2—likely through estrogen receptor signaling (Belcher et al., 2011; Yan et al., 2011, 2013), and curtailed by progesterone (Ma et al., 2017). In our hiPSC-CM model, BPA+E2 caused an exaggerated effect on the spontaneous beating rate (+50.8% above baseline), as did BPA+ERα agonist (PPT; +50% above baseline)—but not BPA+ERβ agonist (DPN; Figure 9C). Pretreatment with either a nonspecific ER antagonist (ICI 182 780) or ERα antagonist (MPP) slightly reduced the beating rate effects of BPA—but pretreatment with an ERβ antagonist did not (PHTPP; Figure 9E). Unexpectedly, progesterone pretreatment exaggerated the effects of BPA on beating rate (+43% above baseline; Figure 9G). A few notable differences were observed when compared with E2. First, coadministration with either an ERα or ERβ agonist did not cause an additive effect on beating rate (Figure 9D). Notably, when E2 was added with an ERβ agonist, loss of spontaneous electrical activity was observed for most replicates. Second, the effects of E2 were attenuated by an ERβ antagonist, but not an ERα antagonist (Figure 9F). Third, the effects of E2 were not altered by progesterone pretreatment (Figure 9H).
Finally, we assessed whether these same pharmacological tools prevented FPDc shortening in BPA or E2-treated hiPSC-CM. In this subset of experiments, 30 μM BPA and 30 μM E2 shortened the FPDc to a similar degree (−13.4% and −17%, respectively), whereas BPA+E2 exaggerated this effect (−21.4%; Figs. 10E and 10F). Pretreatment with an LTCC agonist (Bay K8644) reduced the effects of both BPA and E2 on FPDc, and an LTCC antagonist (verapamil) exaggerated this effect when coadministered with BPA, but not E2 (Figs. 10A–D). FPDc shortening was (slightly) exaggerated when BPA was combined with an ERα agonist (PPT; −16.4%), nonspecific ER antagonist (ICI 182 780; −22.3%), or progesterone (−16.7%)—and reduced when combined with an ERβ agonist (DPN; −12.7%) or ERα antagonist (MPP; −7.7%; Figure 10). E2-treated cells yielded slightly different results; first, an exaggerated shortening of FPDc was not observed with either the ERα agonist (PPT) or progesterone (Figs. 10H and 10P). Second, the nonspecific ER antagonist reduced the effects of E2 (Figure 10L). These findings suggest that E2 and BPA may act on different targets, or may differ in potency for LTCCs and/or estrogen receptors. Additional MEA metrics (eg, conduction velocity, spike amplitude) are thoroughly reported in Supplementary Table 2.
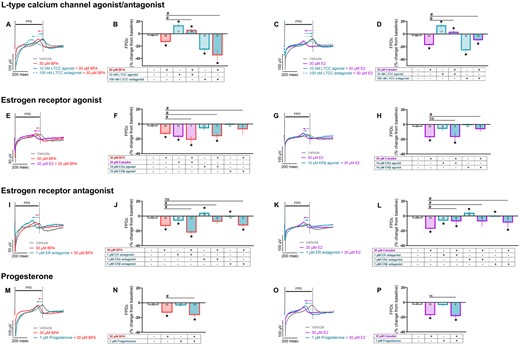
BPA-induced changes on FPDc are exaggerated by coadministration with E2 or calcium channel antagonist. hiPSC-CM were pretreated with a pharmacological agent (15 min) followed by exposure to either 30 μM BPA or 30 μM E2. Field potentials recorded in response to 1.5 Hz pacing. A–D, Pretreatment with an LTCC agonist (Bay K8644) or antagonist (verapamil). E–H, Pretreatment with at ERα agonist (PPT) or ERβ agonist (DPN). I–L, Pretreatment with a nonspecific ER antagonist (ICI 182 780), ERα antagonist (MPP), or ERβ antagonist (PHTPP). M–P, Pretreatment with progesterone. Vehicle and pharmacological agent data repeated across graphs for clarity. Number of independent replicates reported in each bar. Values reported as a percent change from baseline measurement, immediately preceding chemical treatment (mean and 95% confidence interval). ANOVA with multiple comparisons test, *p < .05 relative to vehicle control (0.1% DMSO), #p < .05 relative to either 30 μM BPA alone or 30 μM E2 alone. Samples omitted if not responsive to the desired pacing frequency.
Discussion
To the best of our knowledge, this is the first in vitro study to compare the effects of bisphenol chemicals and estradiol on cardiac physiology using a human relevant model. Key findings of our study include: (1) bisphenol chemicals and estradiol alter cardiac physiology acutely and reversibly (within minutes), indicating nongenomic activity that likely occurs before these chemicals are metabolized and excreted. (2) Despite similarities in structure, the potency of estradiol and bisphenol chemicals differ. Across multiple cardiac metrics, an approximate potency hierarchy includes E2 ≥ BPA ≥ BPF ≫ BPS. (3) BPA and E2 cause similar effects on cardiomyocytes (eg, FPD and APD shortening, smaller depolarizing spike amplitude), but the underlying mechanisms are not identical. (4) Although there were a few exceptions (eg, APD shortening), perturbations in cardiac physiology were largely observed at elevated micromolar concentrations. This finding highlights the fact that hiPSC-CM are a useful screening tool, whereas future studies could validate key findings using a higher-order model (eg, intact electrical conduction system, atrial versus ventricular chamber differences, contractile function against a mechanical load).
Our findings highlight the acute, nongenomic activity of bisphenol chemicals—which aligns with previous experimental studies. First, BPA and E2 have been shown to inhibit voltage-gated sodium channels (O’Reilly et al., 2012; Wang et al., 2011, 2013), which could explain the reduction in cell excitability and slowing of electrical conduction observed in the present study. Next, both BPA and E2 have been shown to interfere with intracellular calcium handling in isolated ventricular cardiomyocytes and hiPSC-CM within a short timeframe (2–15 min) (Hyun et al., 2021; Jiang et al., 1992; Ramadan et al., 2018; Yan et al., 2011). Potential mechanisms underlying the effects on calcium handling and contractility include the phosphorylation of key calcium handling proteins (Gao et al., 2013; Liang et al., 2014), and/or the inhibition of inward calcium current (Deutschmann et al., 2013; Hyun et al., 2021; Jiang et al., 1992; Liang et al., 2014; Meyer et al., 1998; Prudencio et al., 2021). Although the reported potency of BPA on LTCCs does vary across models, ranging from approximately 16.5% at 1 nM BPA in hiPSC-CM, approximately 12% at 10 nM in rat ventricular cardiomyocytes, and approximately 10% at 5 μM BPA in transfected cell lines (Liang et al., 2014; Ma et al., 2023b; Prudencio et al., 2021). As expected with calcium channel inhibitors, we found that both BPA and E2 hasten repolarization by shortening the APD and FPD in hiPSC-CM in a dose-dependent manner, which aligns with previous work (Hyun et al., 2021; Jiang et al., 1992; Prudencio et al., 2021). Of interest, at least 1 study suggests that BPA exposure can delay repolarization through the inhibition of hERG channels at low nanomolar concentrations, before the onset of calcium channel inhibition at higher concentrations (Ma et al., 2023a). Our results complement this finding and provide valuable insight into the effects of BPA at elevated exposure levels. Although micromolar concentrations may only be observed in occupational or clinical settings (Ramadan et al., 2020), exposure to multiple bisphenol chemicals may elicit pronounced effects (Arrokhman et al., 2023). Nevertheless, the effects of E2 and BPA on cardiac electrophysiology and calcium handling have been shown to occur quickly and reversibly. Our pharmacology study focused on calcium channel activation and/or inhibition; however, the effects of bisphenol chemicals can be multifactorial and future studies should explore the interaction between bisphenols and other voltage-gated ion channels (eg, Nav1.5, hERG).
In the presented study, we observed similar perturbations in hiPSC-CM physiology upon exposure to either BPA or E2; yet, our pharmacological studies indicate that these 2 chemicals have a few distinct features. First, pretreatment with either an LTCC antagonist or ERα agonist exaggerated the effects of BPA on beating rate and FPD (see Supplementary Table 2 for additional MEA measurements)—but a similar effect was not observed with E2. Second, pretreatment with an ERα antagonist reduced (albeit only slightly) the effects of BPA on beating rate and FPD—but this was not observed with E2. Notably, a link between BPA cardiotoxicity and estrogen receptor signaling has previously been documented, whereby the effects of BPA were abolished in an ERβ knockout mouse or following treatment with an ERβ blocker (Belcher et al., 2011; Gao et al., 2013; Liang et al., 2014; Yan et al., 2011). This result differs from the current study, as pretreatment with an ERβ blocker did not completely absolve the effects of BPA on cardiac physiology. Distinct outcomes between studies could be attributed to species-specific differences (eg, isolated rodent ventricular cardiomyocytes vs hiPSC-CM), and/or the contribution of external stressors (eg, isoproterenol, ischemia-reperfusion injury) that were used in parallel with bisphenol exposure in prior studies. Collectively, these studies highlight the complexity of (acute) BPA effects on the heart, which can be influenced by multiple factors including its interaction with cardiac ion channels, as well as estrogen receptor signaling (Supplementary Figure 1). To fully address these different factors, future work could compare ER expression levels in cardiomyocytes between different models and/or utilize a longer exposure timeframe to address BPA-induced effects at the genomic level.
Consistent with our earlier work (Prudencio et al., 2021), this study confirmed that the potency of E2 and bisphenol chemicals differ markedly. We previously showed that BPA is a more potent inhibitor of cardiac ion channel currents (INa, ICa,L, IKr), as compared with either BPF or BPS. Pharmacological studies suggest that bisphenol chemicals physically interact with the extracellular component of voltage-gated calcium channels—and this interaction is influenced by the angular orientation of each bisphenol compound (calcium channel inhibition: BPA > BPF > BPS) (Deutschmann et al., 2013). Further, a recent study comparing 14 different bisphenol chemicals using aortic ring preparations reported that BPS had negligible biological effects (Tvrdý et al., 2023). In agreement, we report here that BPS had negligible effects on hiPSC-CM beating rate, FPD, APD, or CaD—suggesting it may offer a superior cardiac safety profile as compared with BPA. However, future work is still required to extensively study these replacement chemicals, as other groups have reported that BPS can interfere with calcium handling and contractility in (rodent) ventricular cardiomyocytes or intact heart preparations (Ferguson et al., 2019; Gao et al., 2015). This discrepancy may be attributed to the species differences and the experimental model used in each study. As examples, rodent ventricular cardiomyocytes have a shorter APD and rely more heavily on the sarcoendoplasmic reticulum calcium ATPase for calcium removal compared with human cells (Bers, 2008; Ripplinger et al., 2022); yet, hiPSC-CM do not fully replicate adult human ventricular myocytes given their automaticity and immature calcium handling machinery (Karbassi et al., 2020; Salameh et al., 2023). Nevertheless, comparative studies have demonstrated excellent correlation between FPDc measurements in hiPSC-CM and clinical QTc measurements in response to pharmacological agents—supporting the use of hiPSC-CM as a translational model and screening tool for toxicological assessments (Blinova et al., 2017).
Conclusion and limitations
Epidemiological studies suggest an association between BPA exposure and adverse cardiovascular outcomes (Bao et al., 2020; Cooper and Posnack, 2022; Moon et al., 2021), yet there remains a paucity of experimental evidence for the source of bisphenol-induced effects. This study builds upon our previous findings (intact rodent heart model [Prudencio et al., 2021])—by using hiPSC-CM, which have been regarded as a new alternative approach methodology with high translational potential (Daley et al., 2023). In the current study, we present novel information about the acute effects of bisphenol chemicals (BPA, BPF, and BPS) on cardiac electrophysiology—and compare these findings with the most prominent circulating estrogen, 17β-estradiol. Using a broad range of environmentally and clinically relevant doses (7 doses from 0.01–100 μM), we determined that bisphenols have an immediate, nongenomic effect on cardiac electrophysiology and intracellular calcium handling. Observed effects occurred in a dose-dependent manner, with more significant perturbations observed at micromolar concentrations—which may only be observed in occupational or clinical environments. Of interest, BPS had negligible effects on cardiac physiology even at the highest dose tested (100 μM), suggesting that it could be a safer replacement chemical—whereas BPF exerted modest effects on beating rate, spike amplitude, field potential, and APD. Given the efforts to remove BPA from (some) consumer products in favor of “safer” chemical alternatives (eg, BPS, BPF), additional work will be required to fully evaluate the biological activity and safety of these replacement chemicals (Rochester and Bolden, 2015; Trasande, 2017). Notably, nuclear receptor transactivation assays indicate that BPA, BPF, and BPS have comparable estrogenic activities—but slight differences in their chemical structure may explain their variable potency toward other nuclear receptors and/or ion channels (Deutschmann et al., 2013; Kojima et al., 2019). Current bisphenol toxicity studies have largely been limited to endocrine effects, yet, our study highlights the importance of investigating other organ systems—which may have unique responsiveness to bisphenol analogs.
Although these results add important insight to our understanding of bisphenol cardiac toxicity, a few limitations should be considered. First, we utilized hiPSC-CM in our study, which are known to have an immature structural phenotype (eg, sparse T-tubules, less organized sarcomeres). Compared with adult ventricular cardiomyocytes, hiPSC-CM continue to beat spontaneously in cell culture and have a reduced inward rectifier K+ (IK1) current (Karakikes et al., 2015; Kim et al., 2015). Nevertheless, hiPSC-CM have comparable ion channel (Karakikes et al., 2015; Koivumäki et al., 2018) and estrogen receptor (Uosaki et al., 2015) expression to adult cardiomyocytes—allowing them to serve as a valuable, high-throughput model for cardiotoxicity testing. Notably, the hiPSC-CM utilized in this study were derived from a single female donor to control for cell line variability and isolate the outcomes specifically attributed to bisphenol chemical exposure. Future work should interrogate the effects of donor sex, age, and ethnicity to improve broad applicability. Second, it is important to note that data collected from a cellular monolayer is distinct from a coordinated three-dimensional (3D) tissue. Although iCell hiPSC-CM are a composition of different cell types (nodal, atrial, ventricular), the majority are ventricular cardiomyocytes, therefore these cells may be less sensitive to toxins that target pacemaker or atrial cardiomyocytes. Accordingly, in vitro screening studies should be validated using a more complex 3D model. With the introduction of “heart-on-a-chip” (3D cardiac microtissues derived from hiPSCs along with microfluidic chips [Abulaiti et al., 2020]) and “clinical trial in a dish” (the ability of hiPSC derivatives to recapitulate patient-specific drug responses [Lam and Wu, 2021]), future cardiotoxicity studies could attain greater translational value using these models. Ex vivo whole heart studies can also provide a better understanding of bisphenol cardiotoxicity, as this model benefits from an intact electrical conduction system. Finally, a future focus on in vivo models would take into account bisphenol chemical metabolism, which is an important consideration for longer time course studies, as BPA is metabolized and excreted within a few hours (Völkel et al., 2002).
Supplementary data
Supplemental files available at Toxicological Sciences online.
Declaration of conflicting interests
The authors declare that the research was conducted in the absence of any commercial or financial relationships that could be construed as a potential conflict of interest.
Acknowledgments
The authors gratefully acknowledge Devon Guerrelli, Jenna Pressman, Anysja Roberts, and Sam Allen for technical assistance and helpful scientific discussions related to this project.
Author contributions
B.C. and S.S. performed experiments; B.C., S.S., N.G.P. analyzed data; B.C. and N.G.P. prepared figures; B.C. and N.G.P. drafted the manuscript; B.C. and N.G.P. conceived and designed experiments; B.C., S.S., and N.G.P. approved the manuscript.
Funding
National Institutes of Health (R01HL139472, F31HL162549), The Sheikh Zayed Institute for Pediatric Surgical Innovation, and the Children’s Research Institute.
Comments