-
PDF
- Split View
-
Views
-
Cite
Cite
Danielle Kozlosky, Cathleen Doherty, Brian Buckley, Michael J Goedken, Richard K Miller, Dan Dongeun Huh, Emily S Barrett, Lauren M Aleksunes, Fetoplacental disposition and toxicity of cadmium in mice lacking the Bcrp transporter, Toxicological Sciences, Volume 197, Issue 2, February 2024, Pages 132–146, https://doi.org/10.1093/toxsci/kfad115
- Share Icon Share
Abstract
The environmental toxicant cadmium (Cd) impairs the growth of rodents and humans in utero which in turn heightens susceptibility to diseases later in life. We previously demonstrated that the maternal-facing efflux transporter, breast cancer resistance protein (human BCRP/ABCG2, mouse Bcrp/Abcg2) confers resistance against Cd toxicity in human trophoblasts. In the current study, we sought to determine whether the absence of Bcrp alters the fetoplacental disposition and toxicity of Cd in mice. Pregnant female wild-type (WT) and Bcrp-null mice (n = 9–10/group) were administered a single injection of saline (5 ml/kg) or CdCl2 (5 mg/kg) on gestational day (GD) 9. Following Cd treatment, Bcrp-null offspring were shorter and accumulated more Cd in their placentas on GD 17 compared with WT mice. Because Cd can adversely impact placentation and transplacental nutrient delivery in mice, multiple pathways were assessed using morphometrics and immunohistochemistry including placenta zonation, vasculature development, and nutrient transporter expression. Most notably, the placentas of Bcrp-null mice had reduced immunostaining of the cell adhesion marker, β-catenin, and the trophoblast marker, cytokeratin, as well as decreased expression of divalent metal nutrient transporters (Dmt1, Zip14, and ZnT1) following Cd treatment. In summary, the absence of Bcrp expression increased placental concentrations of Cd which was associated with shorter fetal size that may be related to differential changes in molecular patterns of placental development and nutrition.
One function of the placenta is to safeguard the fetus from exposure to toxicants. This protection is due, in part, to the expression of efflux transporters that enable the active removal of chemicals from the placenta to the maternal circulation. The breast cancer resistance protein (human BCRP/ABCG2, rodent Bcrp/Abcg2) is one such efflux transporter expressed by syncytiotrophoblasts and extravillous trophoblasts in humans and rodents (Allikmets et al., 1998; Vähäkangas and Myllynen, 2009). Substrates of BCRP include endogenous chemicals (ie, estrogens, uric acid, and bile acids) as well as a wide array of drugs including some prescribed during pregnancy (ie, glyburide and nitrofurantoin) (Gedeon et al., 2006, 2008; Ni et al., 2010). Impairment of BCRP function can therefore cause xenobiotics to accumulate in the placenta (and potentially fetus) leading to perinatal disease. Mice are often used to study BCRP function in vivo because the human and rodent genes share over 80% homology (Allen et al., 1999). Bcrp-null mice are fertile and have previously been used to assess the placental disposition of xenobiotics (Allen et al., 1999; Szilagyi et al., 2019; Zhang et al., 2007; Zhou et al., 2008).
Cadmium (Cd) is a toxic environmental metal. Human exposure to Cd occurs through use of tobacco products, exposure in the workplace, and consumption of contaminated water or diets. Cadmium accumulates in multiple organs including the placenta (Erboga and Kanter, 2016; Geng and Wang, 2019; Xiong et al., 2020; Yamagishi et al., 2016) where it binds to metallothionein to prevent subsequent cellular stress (Guo et al., 2018; Xiong et al., 2021; Zhu et al., 2021). The ubiquity of Cd is so pronounced that measurable levels have been detected in up to 98% of pregnant women though concentrations can vary significantly between countries (Arbuckle et al., 2016; Hoover et al., 2020; Xu et al., 2022). Epidemiological studies have demonstrated that a higher level of Cd exposure is often inversely associated with infant size which can lead to impaired development (Gardner et al., 2013; Geng and Wang, 2019; Kippler et al., 2012; Llanos and Ronco, 2009; Piasek et al., 2014; Romano et al., 2016; Sabra et al., 2017; Wang et al., 2018a; White et al., 2018; Zhang et al., 2016).
The growth of the fetus is influenced by a variety of factors, including disruption of placental development and the transplacental transfer of nutrients. The majority of exchange in the rodent placenta occurs in the labyrinth zone, which is the same region where Cd preferentially accumulates (Yamagishi et al., 2016). In the labyrinth, maternal blood vessels are lined with trophoblast cells and exhibit mature erythrocytes, whereas fetal sinusoids are comprised of endothelial cells and contain nucleated red blood cells (Green et al., 2020; Suzuki et al., 1997; Zhao et al., 2018). The vasculature is crucial for delivery of blood to the fetus and as a result, is sensitive to disruption by toxicants. The overall transfer of nutrients is dependent not only on blood flow, but also the expression of nutrient transporters in the placenta. Metal micronutrients essential for fetal growth and development, such as zinc (Zn), calcium, iron, and copper as well as toxic metals including Cd enter through divalent metal uptake transporters Dmt1/Slc11a2 (divalent metal transporter 1) and ZRT/IRT like protein 14 (Zip14/Slc39a14) (Fujishiro et al., 2012; Girijashanker et al., 2008; Himeno et al., 2009; Nakamura et al., 2012; Pinilla-Tenas et al., 2011; Tallkvist et al., 2001; Widhalm et al., 2020). Unlike Dmt1 and Zip14 transporters, ZnT1/Slc30a1 (zinc transporter 1) liberates Zn from intracellular stores in the placenta, enabling transfer to the fetus (Liuzzi et al., 2001). An additional critical nutrient for fetal growth is glucose, which is transferred from mother to fetus through facilitated carriers GLUT1/SLC2A1 (glucose transporter 1) and GLUT3/SLC2A3 (glucose transporter 3) (Illsley, 2000; Illsley and Baumann, 2020). Rodent studies have shown an inverse correlation between Cd exposure and the placental expression of both Zn (Fernandez et al., 2007; Wang et al., 2016) and glucose transporters (Xu et al., 2016).
Our laboratory recently identified Cd as a substrate of BCRP. Cells overexpressing the human BCRP transporter required a 7-fold higher Cd concentration to induce cytotoxicity comparable to cells transfected with an empty vector (Wen et al., 2021). Likewise, knockdown of BCRP protein expression in cultured human trophoblasts increased Cd accumulation and cytotoxicity in vitro (Zhang et al., 2023). Although these data are compelling, it is currently unknown whether BCRP/Bcrp alters Cd accumulation and early life development in vivo. Therefore, this study investigated the placental accumulation of Cd and mechanisms of fetoplacental toxicity in mice lacking expression of the Bcrp transporter.
Materials and methods
Reagents and chemicals
Cadmium chloride (CdCl2, catalog number 202908) was purchased from Sigma-Aldrich Chemical Co. (St. Louis, Missouri). Anti-ZnT1 (PA5-37463) and anti-Glut3 (MA5-32697) antibodies were purchased from Invitrogen ThermoFisher Scientific (Waltham, Massachusetts). Anti-Dmt1 (ab55735), anti-CD34 (ab8158), anti-VE-cadherin (vascular endothelial cadherin) (ab282277), anti-pan Cytokeratin (ab9377), anti-β-catenin (ab223075), anti-Glut1 (ab115730), and anti-Zip14 (ab140973) antibodies were purchased from Abcam (Boston, Massachusetts). Bxp-53 antibody (ALX-801-036-C100) was purchased from Enzo Life Sciences (Farmingdale, New York).
Animal treatment
Male and female C57BL/6Crl (strain 027, wild-type, WT) mice were obtained from Charles River Laboratories (Wilmington, Massachusetts). Bcrp-null mice were obtained from Taconic Biosciences (Taconic, New York) and backcrossed to the C57BL/6 027 mouse strain until over 99% congenic (Rutgers Infinite Biologics, Piscataway, New Jersey). All animals were provided food (Purina PicoLab Diet 5053 and 5058, WF Fisher, New Jersey) and water ad libitum. Groups of 7- to 12-week-old adult WT and Bcrp-null male and female mice were mated together overnight. The following morning, mice were separated, denoting gestational day (GD) 0. On GD9, pregnant female mice (n = 9–10/genotype) were administered a single dose of saline vehicle (0.9% NaCl, 5 ml/kg, IP) or CdCl2 (5 mg/kg, IP). The dose and the route of CdCl2 administration were based on prior studies investigating mechanisms of Cd-induced fetal growth restriction in mice (Lutz and Beck, 2000; Wang et al., 2012, 2018a; Zhu et al., 2019). Moreover, this dose of CdCl2 yields placental Cd concentrations consistent with prior human epidemiological studies across the globe (∼50 to over 4300 ng/g) (Al-Saleh et al., 2014, 2015; Guo et al., 2010; Kippler et al., 2010).
Dams, fetuses, and placentas were weighed and tissues were collected between 9:00 and 11:00 am on GD17. Fetal crown-to-rump length and placental diameter were measured using calipers (Fischer Scientific, Pittsburgh, Pennsylvania). The average of a vertical and a horizontal measurement determined placental diameter (d) which was used to calculate placental area (1/4πd2). Fetal trunk blood from individual fetuses was used to measure fetal glucose concentrations using a blood glucose monitoring system (TRUEtrack glucometer, Trividia Health, Fort Lauderdale, Florida). Measurements of the anogenital distance at the time of tissue collection were used to indicate fetal sex (Hotchkiss and Vandenbergh, 2005) and confirmed by PCR for genes specific to the Y-chromosome (Sry and Ssty1,2) (McFarlane et al., 2013; Touré et al., 2019).
Fetuses and placentas were snap-frozen in LN2 or preserved in RNAlater and stored at −80°C for mRNA and inductively coupled plasma-mass spectrometry (ICP-MS) analyses. Pieces of fetuses and placentas from each litter were fixed in 10% zinc formalin (Fischer Scientific) for morphological preservation and stored at 4°C for histology. The Rutgers Institutional Animal Care and Use Committee approved these experiments under protocol no. 999900006.
Inductively coupled plasma-mass spectrometry
Maternal plasma, fetal, and placental Cd concentrations were quantified using ICP-MS. Fetal and placental Zn concentrations were also measured. In brief, samples (100 μl or 20–1000 mg) were mixed with ultra-high purity concentrated HNO3 (5:1 HNO3, VWR, Radnor, Pennsylvania) and sonicated (ultrasonic water bath) for 1–3 h, followed by subsequent digestions using a CEM microwave system (CEM Mars X, Matthews, North Carolina) in 5-min intervals for a total of 25 min at 300 W (75%–100% power). Primary dilutions were created by adding MilliQ water to produce an acid concentration of 5% HNO3 (∼50-fold dilution). Samples (100 μl of primary dilutions) were further diluted with 5% HNO3 to the appropriate concentration range for ICP-MS analysis (dilution factor = 50- to 5000-fold). A Nu Attom high-resolution ICP-MS operated at low resolution (300) was used to quantify total Cd and Zn concentrations in digested samples. Three replicates of masses 110Cd, 111Cd, and 67Zn were measured in deflector jump mode with 500 µs peak dwell time, 200 sweeps, and 10 cycles, and averaged (RSD < 5%). Calibration standards were prepared on the day of analysis with Cd and Zn concentrations ranging from 0.001 to 10 μg/kg, in 5% HNO3, with instrument limits of detection (LOD) of 0.004 µg/kg for Cd and 0.05 µg/kg for Zn. The limit of quantification (LOQ) was calculated as the LOD multiplied by the dilution factor, with 0.20 µg/kg or 0.66 µg/kg wet weight for fetal and placental Cd, respectively, and 0.09 mg/kg or 0.41 mg/kg wet weight for fetal and placental Zn, respectively. QA/QC protocols were used during the extraction and analysis procedures. Quality control standards (NIST Calibrants SM-1811-001 and SM-1811-005) represented ∼20% of sample analysis and were measured to account for instrument drift and reproduced with RSD <3.1% for Cd and <1.3% for Zn. Analyte recovery was previously demonstrated to be 96%–101% in matrix-spiked control samples.
Placenta histomorphology
Placentas were fixed in zinc formalin for at least 48 h prior to being cut in half perpendicular to the chorionic plate prior to embedding in paraffin. Sections of placentas were cut to 5 µm prior to staining with hematoxylin and eosin. Histopathological evaluation of placenta sections was performed by a board-certified veterinary anatomic pathologist who examined slides for the presence of overt abnormalities, inflammation, cellular degeneration and necrosis, congestion, and apoptosis. In addition, 4 nonoverlapping images of the placental labyrinth zone were obtained at 20× magnification using a Jenoptik Gryphax microscope camera (Jenoptik, Fremont, California). Fetal and maternal blood vessels were distinguished according to key features. Fetal vessels are lined with endothelium and contain immature erythrocytes whereas maternal vessels are lined with trophoblast cells and carry mature red blood cells (Green et al., 2020; Suzuki et al., 1997; Zhao et al., 2018). ImageJ was used to outline and quantify the area of both types of sinusoids according to an established protocol (Guo et al., 2018; Mirbod, 2018; Wang et al., 2012; Ward et al., 2012).
RNA isolation and mRNA quantification
Pieces of fetal rump or placenta (≤ 30 mg) were placed in a round bottom 2 ml microcentrifuge tube with 700 ml of Buffer RLT. Samples were homogenized for 5 min at 50 Hz (Tissue-Lyser LT, Qiagen, Germantown, Maryland) after the addition of 1% β-mercaptoethanol and a 5-mm stainless steel bead. A RNeasy kit was used to isolate RNA from tissues according to the manufacturer’s protocol (Qiagen). RNA concentration (Nanodrop 2000 spectrophotometer, ThermoFisher Scientific) and purity (2100 Bioanalyzer System, Agilent, Santa Clara, California) were then determined, and cDNA was generated using a transcription kit (High-capacity cDNA reverse transcription kit, ThermoFisher Scientific) and a Thermal Cycler (MultiGene OptiMax Thermal Cycler, Labnet International Inc., Edison, New Jersey). mRNA expression of specific genes was determined using cDNA, Sybr Green dye (ThermoFisher Scientific), specific primers (Supplementary Table 1) (Integrated DNA Technologies, Inc., Coralville, Iowa), and a ViiA7 RT-PCR System (ThermoFisher Scientific). For fetal sex, raw Ct values over 27 indicated poor expression of genes specific to the Y-chromosome (Sry, Ssty1, 2).
Immunohistochemical staining
Paraffin-embedded sections (5 μm) were dewaxed in an oven at 60°C before being rehydrated in xylene and decreasing alcohol concentrations to water. Slides were placed in a citrate-based antigen unmasking solution (Vector Laboratories, Newark, California) and heated to 95°C by microwave. Endogenous peroxidases were quenched using 3% H2O2 for 10 min. Slides were then blocked using an Avidin/Biotin blocking kit (Vector Laboratories) for 15 min before application of 5% species-appropriate serum for 30 min. Sections were then incubated with primary antibodies against Bcrp/Bxp-53 (1:100), CD34 (1:100), VE-cadherin (1:50), pan-cytokeratin (1:100), β-catenin (1:100), Glut1 (1:500), Glut3 (1:200), Dmt1 (1:100), Zip14 (1:100), or ZnT1 (1:100) overnight at 4°C. Negative control slides did not include primary antibody. Twenty-four hours later, secondary antibody (antimouse, antirabbit, or antirat IgG) was added for 1 h before application of avidin-biotin complex (Abcam) for 30 min. Slides were then stained with 3,3′-diaminobenzidine (ImmPACT DAB Substrate Kit, Peroxidase, Vector Laboratories). Nuclei were counterstained with hematoxylin. Slides were then dehydrated and covered before immunostaining intensity was quantified using an ImageJ Fiji package (Crowe and Yue, 2019). Briefly, images of DAB-stained slides were exported into ImageJ Fiji package, following which image deconvolution was conducted. An examiner blinded to treatment groups determined the threshold of each DAB-stained image and quantified the signal area which was then normalized to the average measured nucleus size for semiquantitation of DAB staining intensity.
Placenta glycogen
Paraffin-embedded sections (5 μm) were stained with Periodic acid-Schiff (PAS) and hematoxylin. Slides were scanned at 20× magnification using an Aperio ImageScope slide scanner system (Lecia Biosystems, Illinois). ImageJ was used to quantify the percentage of positive PAS staining area in each placenta using a modification to a previously described protocol (He et al., 2017).
Statistical analysis
Measurements from individual male or female fetal-placental units were averaged within each litter and as a result the sample size was based on the number of litters per treatment group. Within-litter variation was controlled by selecting representative fetal-placental units from various positions across the uterine horn. No significant differences were observed between sexes. Therefore, experimental values from 1 male and 1 female offspring per dam were averaged together for each endpoint. Data are shown individually and expressed as mean ± standard error (SE). Differences across genotypes and treatment groups were analyzed with 2-way ANOVAs followed by a Šidák multiple comparison post hoc test using GraphPad Prism version 9 software (GraphPad Software Inc., La Jolla, California). Significance was set at p ≤ .05.
Results
Bcrp expression and Cd accumulation in WT and Bcrp-null pregnant mice treated with CdCl2
Consistent with prior literature (Staud et al., 2006; Wang et al., 2006), positive Bcrp immunostaining was observed on syncytiotrophoblasts in the placental labyrinth of WT fetuses (Figure 1A). Interestingly, the expression of Bcrp was increased 38% in the placentas of WT mice following Cd administration (Figure 1B). As expected, no Bcrp expression was detected in the placentas of Bcrp-null mice (Figs. 1A and 1B).
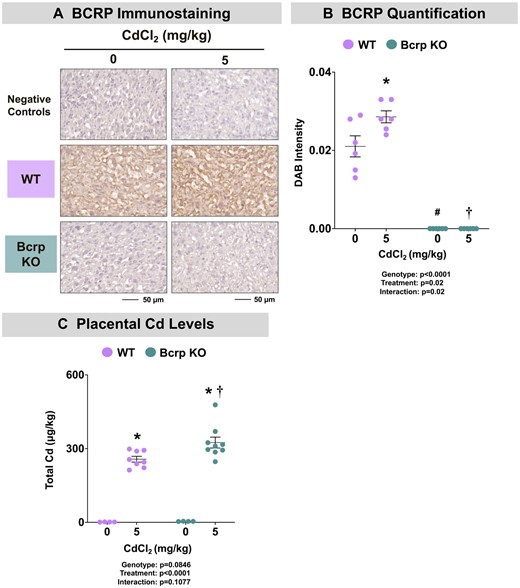
Bcrp expression and cadmium concentrations in offspring of WT and Bcrp-null pregnant mice treated with CdCl2. Pregnant mice were treated with a single injection of saline (5 ml/kg, IP) or CdCl2 (5 mg/kg, IP) on GD9. Fetuses and placentas were collected on GD17 and preserved in 10% zinc formalin for histology or snap-frozen in LN2 for Cd quantification. A, Sections (5 µm) of placentas from dams treated with saline or CdCl2 (5 mg/kg) were stained for Bcrp using immunohistochemistry (Bxp-53 antibody, 1:100). Negative control slides omitted incubation with primary antibody. Magnification was set at ×40, and the scale bar = 50 µm. B, ImageJ Fiji package was used to semiquantify the intensity of DAB staining. C, Total Cd concentrations in placentas were quantified using inductively coupled plasma mass spectrometry. Individual data are presented in addition to mean ± SE (n = 4–10 dams). Two-way ANOVA. *p ≤ .05, compared with saline-treated mice of the same genotype. #p ≤ .05, compared with saline-treated WT mice. †p ≤ .05, compared with Cd-treated WT mice.
To determine whether the absence of Bcrp expression altered Cd disposition, concentrations were quantified in maternal plasma, fetuses, and placentas of both genotypes. Cd concentrations in the plasma of vehicle-treated mice of both genotypes were near the limit of detection (Table 1). As expected, plasma Cd concentrations in dams of both genotypes treated with Cd were significantly elevated compared with matched controls (Table 1). Although Cd-treated Bcrp-null dams had slightly higher plasma Cd concentration compared with Cd-treated WT dams, this difference was not statistically significant (31%, p = .2) (Table 1). The concentration of Cd in placentas from vehicle-treated WT and Bcrp-null mice was near the limit of detection. Notably, the placentas of Bcrp-null mice accumulated higher Cd concentrations than WT placentas following Cd treatment (Figure 1C). Concentrations of Cd in WT and Bcrp-null fetuses were near the detection limit and were <1% of the Cd concentrations of the placenta (data not shown) suggesting minimal transplacental transfer.
. | WT . | Bcrp-null . | ||
---|---|---|---|---|
Treatment . | Saline . | 5 mg/kg CdCl2 . | Saline . | 5 mg/kg CdCl2 . |
Maternal Cd (μg/l) | 0.42 ± 0.06 | 1.86 ± 0.2* | 0.73 ± 0.25 | 2.43 ± 0.36* |
Placental efficiency | 7.7 ± 0.8 | 7.2 ± 0.47 | 6.7 ± 0.54 | 6.3 ± 0.27 |
Viable fetuses (%) | 88 | 85 | 86 | 79 |
Resorptions (%) | 13 | 15 | 14 | 21 |
Female sex (%) | 55 | 43 | 46 | 46 |
. | WT . | Bcrp-null . | ||
---|---|---|---|---|
Treatment . | Saline . | 5 mg/kg CdCl2 . | Saline . | 5 mg/kg CdCl2 . |
Maternal Cd (μg/l) | 0.42 ± 0.06 | 1.86 ± 0.2* | 0.73 ± 0.25 | 2.43 ± 0.36* |
Placental efficiency | 7.7 ± 0.8 | 7.2 ± 0.47 | 6.7 ± 0.54 | 6.3 ± 0.27 |
Viable fetuses (%) | 88 | 85 | 86 | 79 |
Resorptions (%) | 13 | 15 | 14 | 21 |
Female sex (%) | 55 | 43 | 46 | 46 |
Pregnant WT and Bcrp-null dams were treated with a single injection of saline (5 ml/kg, IP) or CdCl2 (5 mg/kg, IP) on GD9. Maternal plasma, fetuses, and placentas were collected on GD17 and measured for size and weight. Viable fetuses and resorption sites were counted. Measurements of the anogenital distance denoted fetal sex which was confirmed by PCR for genes specific to the Y chromosome. Placental efficiency was calculated as the ratio of fetal weight to placenta weight. Total Cd concentrations were quantified in maternal plasma samples using ICP-MS. The detection limit was 0.2 ng/ml. Data are presented as mean ± SE (n = 4 − 10). Two-way ANOVA.
p ≤ .05, compared with saline-treated mice of the same genotype.
. | WT . | Bcrp-null . | ||
---|---|---|---|---|
Treatment . | Saline . | 5 mg/kg CdCl2 . | Saline . | 5 mg/kg CdCl2 . |
Maternal Cd (μg/l) | 0.42 ± 0.06 | 1.86 ± 0.2* | 0.73 ± 0.25 | 2.43 ± 0.36* |
Placental efficiency | 7.7 ± 0.8 | 7.2 ± 0.47 | 6.7 ± 0.54 | 6.3 ± 0.27 |
Viable fetuses (%) | 88 | 85 | 86 | 79 |
Resorptions (%) | 13 | 15 | 14 | 21 |
Female sex (%) | 55 | 43 | 46 | 46 |
. | WT . | Bcrp-null . | ||
---|---|---|---|---|
Treatment . | Saline . | 5 mg/kg CdCl2 . | Saline . | 5 mg/kg CdCl2 . |
Maternal Cd (μg/l) | 0.42 ± 0.06 | 1.86 ± 0.2* | 0.73 ± 0.25 | 2.43 ± 0.36* |
Placental efficiency | 7.7 ± 0.8 | 7.2 ± 0.47 | 6.7 ± 0.54 | 6.3 ± 0.27 |
Viable fetuses (%) | 88 | 85 | 86 | 79 |
Resorptions (%) | 13 | 15 | 14 | 21 |
Female sex (%) | 55 | 43 | 46 | 46 |
Pregnant WT and Bcrp-null dams were treated with a single injection of saline (5 ml/kg, IP) or CdCl2 (5 mg/kg, IP) on GD9. Maternal plasma, fetuses, and placentas were collected on GD17 and measured for size and weight. Viable fetuses and resorption sites were counted. Measurements of the anogenital distance denoted fetal sex which was confirmed by PCR for genes specific to the Y chromosome. Placental efficiency was calculated as the ratio of fetal weight to placenta weight. Total Cd concentrations were quantified in maternal plasma samples using ICP-MS. The detection limit was 0.2 ng/ml. Data are presented as mean ± SE (n = 4 − 10). Two-way ANOVA.
p ≤ .05, compared with saline-treated mice of the same genotype.
Fetoplacental toxicity in WT and Bcrp-null pregnant mice treated with CdCl2
There were no differences in the number of viable fetuses, resorption sites, fetal sex ratio, or placental efficiency in either genotype across treatment groups (Table 1). All dams gained weight over gestation. Interestingly, vehicle-treated Bcrp-null dams had significantly lower weight gain than WT dams by GD17 (Supplementary Figure 1). Although not significant, a similar trend was detected between Cd-treated WT and Bcrp-null dams by GD17 (Supplementary Figure 1). No baseline differences in fetal weight/length or placental weight/area were observed between vehicle-treated WT and Bcrp-null mice (Figure 2). Notably, treatment with Cd did not alter placental weight in either genotype (Figure 2A); however, treatment with Cd reduced placental area to a similar extent in both WT and Bcrp-null offspring compared with matched controls (Figure 2B).
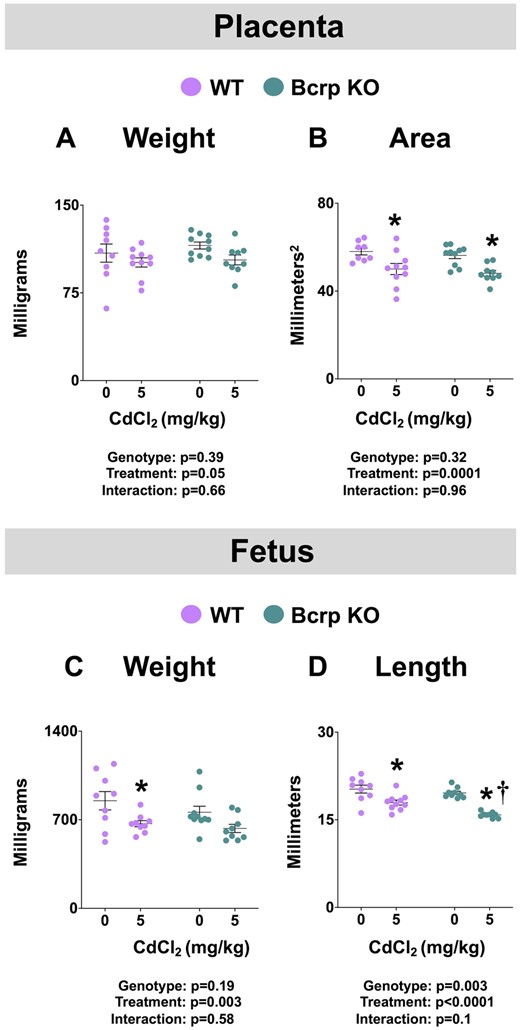
Fetoplacental toxicity in WT and Bcrp-null pregnant mice treated with CdCl2. Pregnant mice were treated with a single injection of saline (5 ml/kg, IP) or CdCl2 (5 mg/kg, IP) on GD9. Fetuses and placentas were collected on GD17. A, Placental and (C) fetal weights were measured. B, Using digital calipers, 2 measurements were taken across the surface of the placenta and averaged for diameter (d). Diameters were used (1/4πd2) to calculate placental areas. D, Fetal crown-to-rump lengths were measured with digital calipers. Individual data are presented in addition to mean ± SE (n = 8–10 dams). Two-way ANOVA. *p ≤ .05, compared with saline-treated mice of the same genotype. †p ≤ .05, compared with Cd-treated WT mice.
In addition, fetal weight was reduced in WT mice following Cd administration and tended to be even lower in Bcrp-null mice (p = .1) (Figure 2C). Treatment with Cd decreased the length of both WT (11%) and Bcrp-null (19%) fetuses; a change that was of significantly greater magnitude in Bcrp-null fetuses (Figure 2D).
Development of placentas in WT and Bcrp-null pregnant mice treated with CdCl2
No abnormal findings were detected upon histopathological examination of WT and Bcrp-null fetuses, placentas, or fetal membranes following Cd treatment (Figure 3A). Within the placenta, the area of the labyrinth and junctional zones were measured. Notably, the area of the various placental layers was similar across treatments and genotypes (Figure 3B and data not shown).
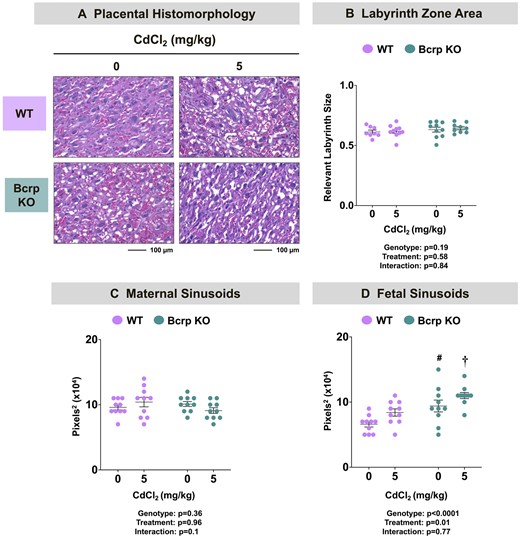
Placental histomorphology and vasculature in WT and Bcrp-null pregnant mice treated with CdCl2. Pregnant mice were treated with a single injection of saline (5 ml/kg, IP) or CdCl2 (5 mg/kg, IP) on GD9. Placentas collected on GD17 were fixed in 10% zinc formalin before routine processing. Morphometric analyses were completed on paraffin-embedded, H&E-stained sections of placentas. A, ×20 magnification images of the placental labyrinth zone of offspring from WT and Bcrp-null pregnant mice treated with saline or CdCl2 (5 mg/kg). Scale bar = 100 µm. B, Images (×4) of placenta halves were taken using Cytation5 Gen5 software. The areas of both the labyrinth zone and junctional zones were outlined separately using the polyline tool. Labyrinth zonal area was normalized to the sum of both zonal areas combined. The areas of (C) maternal and (D) fetal sinusoids in the placental labyrinth zone were quantified using ImageJ. Magnification was set to ×20. Individual data are presented in addition to mean ± SE (n = 8–10 dams). Two-way ANOVA. #p ≤ .05, compared with saline-treated WT mice. †p ≤ .05, compared with Cd-treated WT mice.
In the current study, no difference in the size of maternal blood vessels in the placental labyrinth of vehicle- or Cd-treated WT and Bcrp-null mice was observed (Figure 3C). Interestingly, fetal sinusoids were larger in vehicle- and Cd-treated Bcrp-null placentas compared with WT placentas (Figure 3D) suggesting a baseline difference between genotypes that was not impacted by Cd treatment.
CD34 is a marker of progenitor cells, particularly fetal endothelial cells (Escudero et al., 2014; Resta et al., 2006; Xu et al., 2019). Immunostaining for CD34 protein was observed largely on the basement membrane of endothelial cells in the placental labyrinth zone (Figure 4A). The baseline expression of CD34 in Bcrp-null placentas was about one-third of that detected in vehicle-treated WT placentas (p = .1) (Figure 4B). No change in CD34 immunostaining was detected in either genotype following Cd administration. Another protein with importance in the formation of vasculature is the endothelial cell adhesion protein, vascular endothelial (VE)-cadherin (Vestweber, 2008). In the mouse placenta, VE-cadherin is expressed on trophoblast and endothelial cells (Sung et al., 2022). In this study, fetal endothelium in the placental labyrinth stained positively for VE-cadherin immunostaining (Figure 4C). Baseline expression of VE-cadherin was unchanged across vehicle-treated WT and Bcrp-null placentas (Figure 4D). Treatment with Cd led to a 32% reduction in the immunostaining of VE-cadherin in WT mice with no change in Bcrp-null mice (Figure 4D).
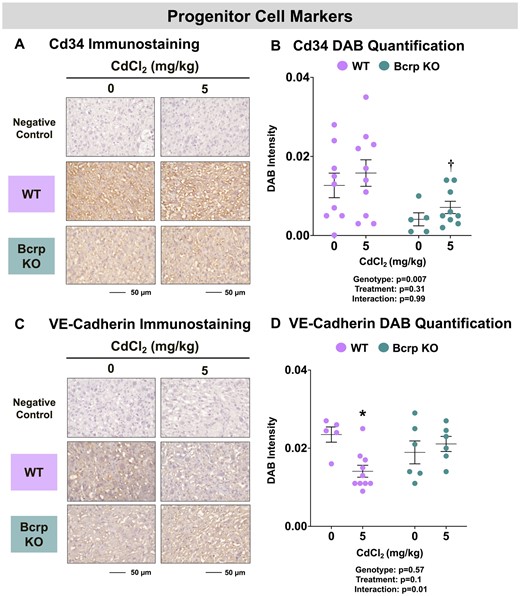
Immunostaining of progenitor cell markers in the placentas of WT and Bcrp-null pregnant mice treated with CdCl2. Pregnant mice were treated with a single injection of saline (5 ml/kg, IP) or CdCl2 (5 mg/kg, IP) on GD9. Placentas were collected on GD17. Placentas preserved in 10% zinc formalin were made into paraffin blocks following routine processing. Sections (5 µm) of placentas from dams treated with saline or CdCl2 (5 mg/kg) were stained for either (A) CD34 (1:100) or (C) VE-cadherin (1:50) using immunohistochemistry. Negative control slides omitted incubation with primary antibody. Magnification was set at ×40, and the scale bar = 50 µm. ImageJ Fiji package was used to semiquantify the intensity of (B) CD34 or (D) VE-cadherin DAB staining. Individual data are presented in addition to mean ± SE (n = 5–10 dams). Two-way ANOVA. *p ≤ .05, compared with saline-treated mice of the same genotype. †p ≤ .05, compared with Cd-treated WT mice.
Although the fetal vasculature is lined with endothelial cells, maternal sinusoids are lined with trophoblast cells. Recognizing this difference, the expression of the cell-adhesion marker, β-catenin, and the trophoblast cell marker, cytokeratin, was evaluated in WT and Bcrp-null placentas. Although the localization of β-catenin in the rodent placenta is unknown, in humans, this cell adhesion marker is expressed in trophoblasts (Wang et al., 2018b). In this study, immunostaining for β-catenin was similarly localized to trophoblasts lining maternal vessels in the placenta labyrinth (Figure 5A). Following Cd administration, the expression of β-catenin was reduced 74% in the placentas of Bcrp-null mice, with no notable changes in WT mice (Figure 5B).
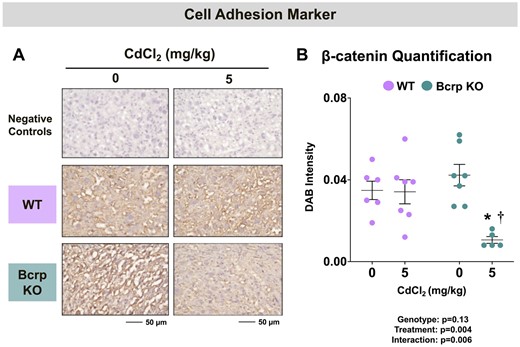
Immunostaining of the cell adhesion marker β-catenin in the placentas of WT and Bcrp-null pregnant mice treated with CdCl2. Pregnant mice were treated with a single injection of saline (5 ml/kg, IP) or CdCl2 (5 mg/kg, IP) on GD9. Placentas were collected on GD17. Placentas preserved in 10% zinc formalin were made into paraffin blocks following routine processing. Sections (5 µm) of placentas from dams treated with saline or CdCl2 (5 mg/kg) were stained for (A) β-catenin (1:100) using immunohistochemistry. Negative control slides omitted incubation with primary antibody. Magnification was set at ×40, and the scale bar = 50 µm. B, ImageJ Fiji package was used to semiquantify the intensity of β-catenin DAB staining. Individual data are presented in addition to mean ± SE (n = 5–7 dams). Two-way ANOVA. *p ≤ .05, compared with saline-treated mice of the same genotype. †p ≤ .05, compared with Cd-treated WT mice.
Cytokeratins are intermediate filaments that are important constituents of trophoblast cells in the rodent placental labyrinth (Zybina et al., 2011). In this study, positive staining for various cytokeratin proteins (using a pan-cytokeratin antibody) was observed in the placenta labyrinth (Figure 6A). The staining intensity for pan-cytokeratin was 150% higher in vehicle-treated Bcrp-null placentas compared with WT placentas (Figure 6B). Following Cd treatment, cytokeratin expression was reduced 70% in Bcrp-null placentas with no change in WT mice (Figure 6B).
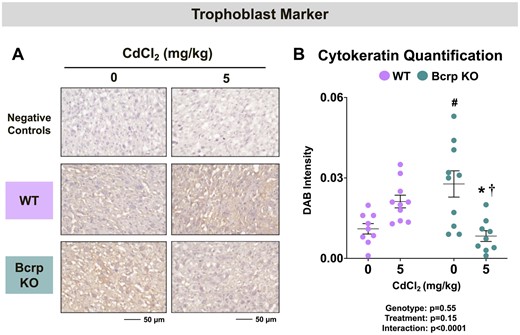
Immunostaining of the trophoblast marker pan-cytokeratin in the placentas of WT and Bcrp-null pregnant mice treated with CdCl2. Pregnant mice were treated with a single injection of saline (5 ml/kg, IP) or CdCl2 (5 mg/kg, IP) on GD9. Placentas were collected on GD17. Placentas preserved in 10% zinc formalin were made into paraffin blocks following routine processing. Sections (5 µm) of placentas from dams treated with saline or CdCl2 (5 mg/kg) were stained for (A) pan-cytokeratin antibody (1:100) using immunohistochemistry. Negative control slides omitted incubation with primary antibody. Magnification was set at ×40, and the scale bar = 50 µm. B, ImageJ Fiji package was used to semi-quantify the intensity of pan-cytokeratin. Individual data are presented in addition to mean ± SE (n = 9 − 10 dams). Two-way ANOVA. *p ≤ .05, compared with saline-treated mice of the same genotype. #p ≤ .05, compared with saline-treated WT mice. †p ≤ .05, compared with Cd-treated WT mice.
Expression of placental divalent metal transporters and fetoplacental nutrient concentrations in WT and Bcrp-null pregnant mice treated with CdCl2
Glucose and Zn are 2 of many nutrients required for growth, metabolism, and development. No differences in fetal or placenta glucose concentrations were observed in either genotype following vehicle or Cd administration (data not shown). Similarly, there were no baseline or treatment-related changes in placental glycogen levels (data not shown). Immunostaining for placental glucose transporters, Glut1 and Glut3 was also conducted. Both transporters were detected at the sinusoidal exchange surface in the placental labyrinth zone (Figs. 7A and 7C). No change in Glut1 or Glut3 staining intensity was detected between vehicle-treated WT and Bcrp-null placentas (Figs. 7B and 7D). The immunostaining of both Glut1 and Glut3 was reduced by one-third in WT placentas with no change in Bcrp-null mice following Cd treatment (Figs. 7B and 7D).
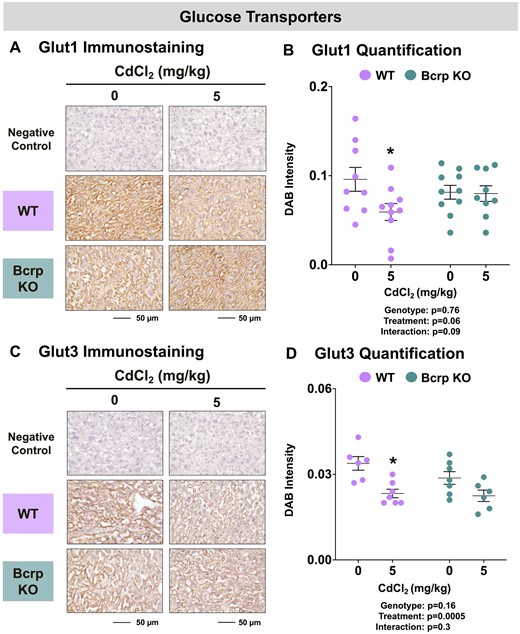
Immunostaining of glucose transporters in the placentas of WT and Bcrp-null pregnant mice treated with CdCl2. Pregnant mice were treated with a single injection of saline (5 ml/kg, IP) or CdCl2 (5 mg/kg, IP) on GD9. Placentas were collected on GD17. Placentas preserved in 10% zinc formalin were made into paraffin blocks following routine processing. Sections (5 µm) of placentas from dams treated with saline or CdCl2 (5 mg/kg) were stained for either (A) Glut1 (1:500) or (C) Glut3 (1:200) using immunohistochemistry. Negative control slides omitted incubation with primary antibody. Magnification was set at ×40, and the scale bar = 50 µm. ImageJ Fiji package was used to semi-quantify the intensity of (B) Glut1 or (D) Glut3 DAB staining. Individual data are presented in addition to mean ± SE (n = 6 − 10 dams). Two-way ANOVA. *p ≤ .05, compared with saline-treated mice of the same genotype.
Divalent metal transporters including Dmt1, Zip14, and ZnT1 mediate the transplacental uptake of Cd as well as Zn, copper, iron, manganese, and additional nutritional and toxic metals. Dmt1 and Zip14 enable the entry of various metals into the placenta from the maternal circulation whereas ZnT1 frees intracellular stores of Zn for the transplacental transfer to the fetus. Although localization data in the rodent placenta are lacking, studies have shown human syncytiotrophoblasts express DMT1 (Li et al., 2012), likely ZIP14 (Widhalm et al., 2020), and ZnT1 (Ford, 2004). In the current study, immunostaining for Dmt1 was detected on fetal sinusoids in the labyrinth whereas Zip14 was localized to the junctional zone (Figs. 8A and 8C). Although no baseline difference in placental Dmt1 (Figs. 8B) staining intensity between vehicle-treated WT and Bcrp-null placentas was detected, immunostaining for Zip14 (Figs. 3 and 8D) was 26% higher in vehicle-treated Bcrp-null placentas compared with WT mice. No change was detected in the staining intensity of Dmt1 or Zip14 in WT placentas following Cd treatment (Figs. 8B and 8D). By comparison, treatment with Cd reduced the immunostaining of Dmt1 by 37% and Zip14 by 49% in the placentas of Bcrp-null mice (Figs. 8B and 8D). Zip14 protein expression was 46% lower in Bcrp-null placentas than WT placentas following Cd treatment (Figure 8D). Found on trophoblasts in the placental labyrinth (Figure 9A), ZnT1 expression was similar in WT and Bcrp-null placentas (Figure 9B). Following Cd treatment, ZnT1 immunostaining was reduced 39% in the placentas of Bcrp-null mice compared with controls, with minimal change in WT mice. Likewise, ZnT1 immunostaining was 40% lower in Bcrp-null placentas compared with WT placentas following Cd treatment (Figure 9B).
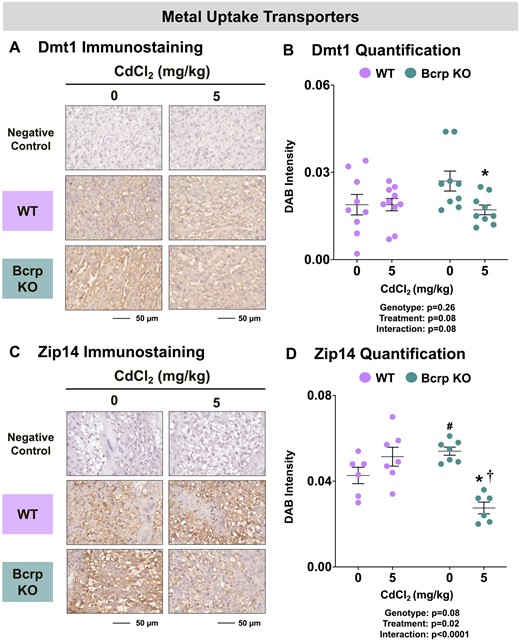
Immunostaining of metal uptake transporters in the placentas of WT and Bcrp-null pregnant mice treated with CdCl2. Pregnant mice were treated with a single injection of saline (5 ml/kg, IP) or CdCl2 (5 mg/kg, IP) on GD9. Placentas were collected on GD17. Placentas preserved in 10% zinc formalin were embedded in paraffin following routine histological processing. Sections (5 µm) of placentas from mice treated with saline or CdCl2 (5 mg/kg) were stained for (A) Dmt1 (1:100) or (C) Zip14 (1:100) using immunohistochemistry. Negative control slides omitted incubation with primary antibody. Magnification was set at ×40, and the scale bar = 50 µm. ImageJ Fiji package was used to semiquantify the intensity of (B) Dmt1 or (D) Zip14 DAB staining. Individual data are presented in addition to mean ± SE (n = 6 − 10 dams). Two-way ANOVA. *p ≤ .05, compared with saline-treated mice of the same genotype. #p ≤ .05, compared with saline-treated WT mice. †p ≤ .05, compared with Cd-treated WT mice.
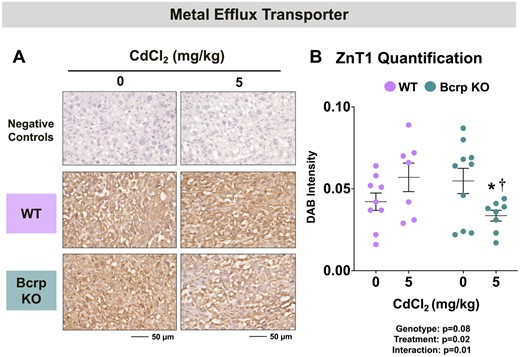
Immunostaining of metal efflux transporter in the placentas of WT and Bcrp-null pregnant mice treated with CdCl2. Pregnant mice were treated with a single injection of saline (5 ml/kg, IP) or CdCl2 (5 mg/kg, IP) on GD9. Placentas were collected on GD17. Placentas preserved in 10% zinc formalin were embedded in paraffin following routine histological processing. Sections (5 µm) of placentas from mice treated with saline or CdCl2 (5 mg/kg) were stained for (A) ZnT1 (1:100) using immunohistochemistry. Negative control slides omitted incubation with primary antibody. Magnification was set at ×40, and the scale bar = 50 µm. B, ImageJ Fiji package was used to semiquantify the intensity of ZnT1 DAB staining. Individual data are presented in addition to mean ± SE (n = 7 − 10 dams). Two-way ANOVA. *p ≤ .05, compared with saline-treated mice of the same genotype. †p ≤ .05, compared with Cd-treated WT mice.
Discussion
Cadmium accumulates in the placenta and is thought to reduce the delivery of nutrients to the fetus which can lead to restricted growth. The placenta can protect the fetus from direct xenobiotic exposures in part through efflux transporters, including BCRP/ABCG2, which function to remove chemicals from the placenta to the maternal circulation. Although we demonstrated that the human BCRP transporter confers resistance against Cd cytotoxicity in vitro (Wen et al., 2021; Zhang et al., 2023), it was not known whether similar protection is afforded to the placenta and fetus in vivo. In the present study, higher placental Cd concentrations and reduced fetal length were observed in Bcrp-null offspring on GD17 compared with WT offspring following a single injection of CdCl2 on GD9. Subsequent studies evaluated mechanisms of placental dysfunction, including alterations in the placental vasculature and proteins responsible for the transplacental transfer of nutrients, as potential explanations for reduced fetal growth in mice exposed to Cd. Notably, minimal changes were observed in placental zonation or progenitor cell marker immunostaining that could account for the differential toxicity of Cd between genotypes. Instead, the placentas of Bcrp-null mice had reduced immunostaining of the cell adhesion marker, β-catenin, and the trophoblast marker, cytokeratin, as well as decreased expression of divalent metal nutrient transporters (Dmt1, Zip14, and ZnT1) following Cd treatment. Taken together, the absence of Bcrp expression increased placental concentrations of Cd which was associated with shorter fetal size that may be related to differential changes in molecular patterns of placental development and nutrition.
Cd enters the placenta through divalent metal uptake transporters, namely Dmt1 and Zip14 (Nakamura et al., 2012; Widhalm et al., 2020). In the current study, there was no difference in placental Dmt1 immunostaining between genotypes on GD17. The absence of Bcrp resulted in elevated Zip14 expression in the placenta. It is unclear whether a similar pattern of expression was present on GD9 when CdCl2 was administered. If so, a greater Zip14 expression could have enhanced Cd uptake into the placentas, thereby favoring greater influx in Bcrp-null mice. Bcrp-null dams had significantly higher Cd concentrations on GD17, which may also have influenced the extent of placental Cd accumulation. Once in the placenta, the cellular toxicity imparted by free divalent Cd2+ is limited through sequestration by thiol-containing proteins (eg, metallothionein, Mt) (Barbier et al., 2005; Liu et al., 1995; Patra et al., 2011). No differences in Mt1 or Mt2 mRNA levels were observed in control or Cd-treated placentas of either genotype on GD17 (data not shown). This suggests that there was likely no difference in Cd sequestration within trophoblasts between WT and Bcrp-null mice and does not explain the higher Cd levels in Bcrp-null placentas.
Additional efflux transporters may also participate in the removal of Cd from the placenta, thereby providing some resistance against Cd toxicity. The multidrug resistance protein 1 (MDR1/P-gp/ABCB1 [multidrug resistance protein, P-glycoprotein]) is an efflux transporter with many overlapping substrates to BCRP and likewise is expressed on rodent placental trophoblasts. Prior research has shown that overexpression of the human MDR1 transporter in LLC-PK1 renal cells and treatment with an MDR1 inhibitor significantly increased levels of Cd (Endo et al., 2002; Kimura et al., 2005). This suggests that MDR1 may play a role in the transport of Cd in vitro. Our data show significantly higher basal expression of Mdr1a and 1b mRNAs on GD17 (Supplementary Figure 2) in the placentas of mice lacking Bcrp. Increased expression could reflect a compensatory change in Bcrp-null offspring to adapt to the lack of Bcrp transporter, and thus enhance placental Cd efflux.
Optimal development of the intertwined network of maternal and fetal sinusoids in the placental labyrinth is vital for the fetoplacental transfer of nutrients. In particular, exposing rodents to toxicants throughout gestation has been associated with reduced placental blood vessel area and vasculogenesis, resulting in the insufficient transfer of nutrients. Moreover, disruption of blood vessel development and an increase in placental sinusoidal area have been reported in some human pregnancy complications (eg, gestational diabetes and inflammatory chorioamnionitis). Such pathologic changes lessen placental barrier integrity and reduce fetal delivery of nutrients. Although we observed a similar overall size of WT and Bcrp-null placentas, the area of the fetal sinusoids was larger in Bcrp-null placentas compared with matched WT placentas at GD17 (although not changed by Cd administration). To explore vasculature development in WT and Bcrp-null mice, we measured CD34 protein expression. CD34 is a marker of hematopoietic stem cells and endothelial cells (Abe et al., 2011; Vieira et al., 2005). BCRP is also expressed in adult human hematopoietic stem cells in conjunction with CD34 (Abbott, 2003; Abbott et al., 2002; van den Heuvel-Eibrink et al., 2007). Therefore, lack of Bcrp may correlate with the lower baseline levels in CD34 seen in Bcrp-null placentas but appears to have little relevance to placental responses to Cd administration. Additional markers of vessel development (VE-cadherin), cell adhesion, (β-catenin), and trophoblast cells (pan-cytokeratin) were examined. Decreased VE-cadherin immunostaining was specific to WT mice whereas reduced cytokeratin and β-catenin immunostaining were observed in Bcrp-null mice following Cd exposure. In cells, Cd has been shown to reduce the interaction of β-catenin with epithelial adherens junction components (Chakraborty et al., 2010). As such, Cd may alter placental cell interactions in Bcrp-null offspring.
The net transfer of nutrients is dependent not only upon blood flow but also the enrichment of nutrient transporters. Prior studies have demonstrated that nutrients are often less abundant in Cd-exposed offspring compared with controls (Kanter et al., 2003; Wang et al., 2016; Wier et al., 1990; Xu et al., 2016). Likewise, Cd can reduce glucose transporter expression in mouse placentas (Xu et al., 2016). In this study, immunostaining for Glut1 and Glut3 was reduced in WT mice following Cd treatment, without much change in Bcrp-null placentas. However, no changes in fetal glucose or placental glycogen levels were observed suggesting that this pathway was not a major mechanism for growth restriction in either genotype at GD17. In addition to transporting Cd, Dmt1, Zip14, and ZnT1 are key transporters responsible for the transplacental movement of divalent metals. In this study, immunostaining for Dmt1, Zip14, and ZnT1 was reduced in the placentas of Bcrp-null mice following Cd administration. Although there were no significant differences in fetal or placental Zn concentrations, it is possible that downregulation of these transporters could alter the maternal-fetal delivery of iron, copper, manganese, and other nutritional metals that utilize the same transporters.
In conclusion, Bcrp-null offspring accumulated higher concentrations of Cd in their placentas which could contribute to the observed reduced length of the fetuses. This mechanistic research is important to the field of reproductive and developmental toxicology as Cd has been detected in 98% of pregnant women across the world (Arbuckle et al., 2016; Hoover et al., 2020; Xu et al., 2022). Moreover, Cd exposure is inversely correlated to birth size and perinatal health outcomes. Notably, the human BCRP protein expression varies over 7-fold in healthy human placentas due in part to a nonsynonymous polymorphism variant (rs2231142, Q141K/C421A) that decreases BCRP levels by 50% (Bircsak et al., 2018). Our team has previously demonstrated that infants with the reduced function variant 141K had smaller placentas at higher concentrations of Cd compared with infants with the WT 141Q allele, though no relationships with infant size were observed (Barrett et al., 2023). As a result, additional mechanistic and translational research are needed to dissect how the placental barrier transporters influence the toxicity of environmental chemicals including toxic metals.
Supplementary data
Supplementary data are available at Toxicological Sciences online.
Declaration of conflicting interests
DD Huh is a co-founder of Vivodyne Inc. and holds equity in Vivodyne Inc. and Emulate Inc. The other authors declared no potential conflicts of interest with respect to the research, authorship, and/or publication of this article.
Funding
This work was supported by the National Institutes of Environmental Health Sciences [grants R01ES029275, F31ES032319, T32ES007148, and P30ES005022] and the National Center for Advancing Translational Sciences [grant UL1TR003017].
Comments