-
PDF
- Split View
-
Views
-
Cite
Cite
Elham Dianati, Michael G. Wade, Barbara F. Hales, Bernard Robaire, Isabelle Plante, From the Cover: Exposure to an Environmentally Relevant Mixture of Brominated Flame Retardants Decreased p-β-Cateninser675 Expression and Its Interaction With E-Cadherin in the Mammary Glands of Lactating Rats, Toxicological Sciences, Volume 159, Issue 1, September 2017, Pages 114–123, https://doi.org/10.1093/toxsci/kfx123
- Share Icon Share
Abstract
Proper mammary gland development and function require precise hormonal regulation and bidirectional cross talk between cells provided by means of paracrine factors as well as intercellular junctions; exposure to environmental endocrine disruptors can disturb these processes. Exposure to one such family of chemicals, the brominated flame retardants (BFRs), is ubiquitous. Here, we tested the hypothesis that BFR exposures disrupt signaling pathways and intercellular junctions that control mammary gland development. Before mating, during pregnancy and throughout lactation, female Sprague-Dawley rats were fed diets containing that BFR mixture based on house dust, delivering nominal exposures of BFR of 0 (control), 0.06, 20, or 60 mg/kg/d. Dams were euthanized and mammary glands collected on postnatal day 21. BFR exposure had no significant effects on mammary gland/body weight ratios or the levels of proteins involved in milk synthesis, epithelial-mesenchymal transition, cell-cell interactions, or hormone signalling. However, BFR exposure (0.06 mg/kg/d) down-regulated phospho-ser675 β-catenin (p-β-catSer675) levels in the absence of any effect on total β-catenin levels. Levels of p-CREB were also down-regulated, suggesting that PKA inhibition plays a role. p-β-catSer675 co-localized with β-catenin at the mammary epithelial cell membrane, and its expression was decreased in animals from the 0.06 and 20 mg/kg/d BFR treatment groups. Although β-Catenin signaling was not affected by BFR exposure, the interaction between p-β-catSer675 and E-cadherin was significantly reduced. Together, our results demonstrate that exposure to an environmentally relevant mixture of BFR during pregnancy and lactation decreases p-β-catser675 at cell adhesion sites, likely in a PKA-dependant manner, altering mammary gland signaling.
Brominated flame retardants (BFRs) are incorporated into many consumer products to reduce the flammability of combustible materials and to meet fire safety standards (Watanabe and Sakai, 2003). Polybrominated diphenyl ethers (PBDEs) and hexabromocyclododecane (HBCDD) have been used widely as additive BFR in North America (Alaee etal., 2003; Stapleton etal., 2005). Because they do not form covalent bonds within the polymer matrices to which they are added, PBDEs and HBCDDs are easily released into the environment, resulting in ubiquitous human exposure (Besis and Samara, 2012). In 2008 the Government of Canada adopted a regulation prohibiting the manufacture of certain PBDEs congeners and the use, sale, offer for sale and import of those PBDEs, as well as mixtures, polymers and resins containing these substances (Environment Canada, 2008). Regulatory action was also adopted for HBCDDs, and should come into force in 2017 (Environment Canada, 2010). However, because PBDEs and HBCDDs are released from existing furnishings and other common household items and are persistent, human exposure will likely continue for many years.
The main routes of exposure of BFR are through inhalation and ingestion of dust, as well as dietary consumption (Besis and Samara, 2012). BFR are detected in breast milk, adipose tissue, serum and hair and are bioaccumulative (Hites, 2004; Toms etal., 2009; Zota etal., 2013). Various BFR congeners have been reported to act as endocrine disruptors. Exposure to PBDEs has been associated with adverse effects on male and female reproductive organs and on steroid and thyroid hormone signaling (Chevrier etal., 2010; Ernest etal., 2012; Lefevre etal., 2016a,b; Lilienthal etal., 2006). A previous study showed a significant delay in ductal outgrowth in the mammary gland at PND21 after perinatal exposure to DE-71 (Kodavanti etal., 2010). In vitro, decreased apoptosis and increased proliferation were observed when MCF-7 breast cancer cells were exposed to a combination of PBDEs (BDEs 47, 99, 100, and 209) and estradiol (Kwiecinska etal., 2011).
Mammary gland development and function involve bidirectional communication, composed of paracrine factors and cell junctions between luminal and myoepithelial cells, and are driven by multiple systemic hormones (Gudjonsson etal., 2005; Hennighausen and Robinson, 2005; Stewart etal., 2015). Among others, estrogens (E2), progesterone, prolactin, Jak2/Stat5 signaling pathways, and ERBB2/HER2/NEU receptor tyrosine kinases are involved in tertiary ductal branching, alveologenesis and the synthesis of milk during pregnancy and lactation (Gallego etal., 2001; Hewitt etal., 2002; Humphreys etal., 1997; Jackson-Fisher etal., 2004). Thus, changes in hormonal balance can perturb mammary gland development and function. In accordance, exposure to endocrine disruptors at sensitive periods of life, such as the perinatal life, puberty or pregnancy, have been linked to developmental defect and breast cancer (Cohn etal., 2012; Fenton, 2006). Those sensitive periods involved proliferation, differentiation and apoptosis events that are tightly orchestrated by signaling pathways.
Intercellular junctions are also required for normal mammary gland function. Dysregulation of junctional proteins is associated with breast developmental defects and cancer (Hatsell etal., 2003; Lanigan etal., 2009; Osanai etal., 2007; Plante and Laird, 2008; Stewart etal., 2015). Down-regulation and altered localization of junctional proteins are observed following exposure to a variety of pollutants, suggesting that environmental chemicals may alter mammary gland function (Leithe etal., 2010; Plante etal., 2002; Salian etal., 2009; Trosko, 2011). We recently showed that the composition of gap, adherens, and tight junctions in junctional nexuses and the expression of their components vary during mammary gland development (Dianati etal., 2016). Notably, gap junction proteins, Cx26, Cx30, and Cx32, adherens junction proteins, E-cadherin and β-catenin, as well as tight junction proteins, claudin 3 and claudin 7, were all up-regulated, while Cx43 and P-cadherin were down-regulated during pregnancy and/or lactation (Dianati etal., 2016). These data suggest stage-dependent requirements in cell-cell interactions during mammary gland development and a role for hormones and paracrine factors in their regulation (Nguyen etal., 2001; Risek etal., 1995; Rubenstein etal., 2003). Junctional proteins and their binding partners are also involved in regulating cellular signaling and transduction of signals. Among others, β-catenin plays a pivotal role in mammary gland development and function (Carraway etal., 2005; Hatsell etal., 2003). β-Catenin can either be bound to E-cadherin at the junctional nexus or act as a transcription factor in collaboration with TCF/Lef DNA-binding proteins. This dual role is controlled, in part, by phosphorylation of β-catenin by various kinases, including PKA, CKια, Akt, and GSK3β (Liu etal., 2002; Roura etal., 1999; Spirli etal., 2013; Taurin etal., 2006). Thus, dysregulation of β-catenin has been associated with abnormal mammary gland development, with the epithelial-to-mesenchymal transition (EMT) and with breast cancer (Carraway etal., 2005; Hatsell etal., 2003).
In this study, we tested the hypothesis that exposure to common household flame retardants during pregnancy and lactation alters mammary gland function and signaling pathways. Specifically, we evaluated the effects of an environmentally relevant mixture of PBDE and HBCDD congeners on mammary gland function and development, focusing on: (1) the milk synthesis pathway, (2) hormonal receptors, and (3) the expression, localization and interactions of junctional proteins.
MATERIALS AND METHODS
Exposure to a mixture of BFR
Formulation of the mixture of BFR and preparation of the diets were described previously (Berger etal., 2014; Lefevre etal., 2016a,b; Tung etal., 2016, 2017). Briefly, the BFR dietary mixture contained 3 different technical PBDE flame retardant mixtures (DE-71, DE-79, and BDE-209) and an HBCDD mixture that were combined to yield proportions of PBDE and HBCDD congeners that were comparable to median levels measured in Boston house dust (Allen etal., 2008; Stapleton etal., 2008). Sufficient mixture was combined with powdered diet (0, 0.75, 250, or 750 mg of BFR mixture/kg diet) to deliver a daily nominal dose of 0, 0.06, 20, or 60 mg/kg of body weight/day (mg/kg/d), respectively. Our lowest dose approximated the maximum human exposure, which was calculated based on the dust ingestion rate of 100 mg/d in children and then converted to rat by scaling ratio of 1:6.9 (human to rat body surface area) (Allen etal., 2008; Stapleton etal., 2008). Powdered diets were mixed thoroughly, pelleted and stored at 4°C for no more than 1 month prior to feeding.
Animals
Animals and treatment methods were previously described (Tung etal., 2016). Briefly, virgin female Sprague-Dawley rats were obtained from Charles River Laboratories (Charles River, St-Constant, Quebec, Canada). Animals were housed individually in accordance with the Guide to the Care and Use of Experimental Animals prepared by the Canadian Council Protocol 2012-015. Food and water were provided ad libitum; the rats were housed in rooms maintained with a 12L:12D photoperiod, 40%–70% humidity at 20°C–24 °C. Exposure began at least 1 week prior to mating and was maintained during gestation and lactation. Female rats were acclimated to control diets for 1 week and then randomly assigned to 1 of the 4 diet groups prior to the mating. Females in proestrus, as assessed by vaginal cytology, were then caged with proven breeder male Sprague-Dawley rats (maintained on the control diet) overnight. A sperm-positive vaginal swab on the following morning indicated mating and this was considered as gestational day 0. Females were returned to their home cage and provided with water and the appropriate dietary mixture ad libitum throughout gestation and lactation. Litter size was balanced at 8 pups per dam at postnatal day (PND)4. All females (n = 10–17 dams per group) were allowed to deliver (denoted as PND 0), dams were euthanized within 15 min after being separated from pups at PND 21 by abdominal aorta exsanguination under isofluorane anaesthesia. At the time of necropsy, the mammary glands were sampled and preserved differently depending on the downstream applications. Abdominal mammary glands (pair 4) were weighed and immediately flash-frozen in liquid nitrogen; inguinal mammary glands (pair 5) were also flash-frozen. These glands were used for western blot and immunoprecipitation analyses. Mammary gland pairs 2–3 (upper-thoracic and thoracic) were embedded in Tissue-Tek OCT cryomounting compound (VWR International, Ville Mont-Royal, Quebec, Canada) on dry ice or fixed in 4% formalin and used for histology and immunofluorescence. All unfixed samples were stored at −80 °C.
Western blotting
Frozen tissues were homogenized in ice-cold triple detergent lysis buffer (pH 8) (Tris 50 mM, NaCl 150 mM, 0.02% sodium azide, 0.1% SDS, 1% Nonidet P40, 0.5% sodium deoxycholate) supplemented with 1.25 M of NaF, 1 M of NaVO3 and Halt Protease and Phosphatase Cocktail Inhibitor (Fisher Scientific) and centrifuged at ≥4000 rpm (10 min at 4 °C). Aliquots of the supernatant were stored at −80 °C. Protein concentration was determined using the Pierce BCA protein assay kit (Thermo Scientific, Rockford, Illinois, USA). To perform semi-quantitative western blot analysis (Taylor etal., 2013), total proteins were loaded onto TGX Stain-Free™ Acrylamide gels (BIO-RAD, Mississauga, Ontario, Canada). Immediately after electrophoresis, the gels were transferred onto PVDF membranes using the Trans-Blot Turbo Transfer System (BIO-RAD). Total lane proteins were visualized using the ChemiDoc MP imaging system (BIO-RAD) and quantified using ImageLab 5.2 software (BIO-RAD) for normalization. Membranes were blocked with 5% dry milk in TBS-Tween 0.1% and probed overnight at 4 °C with primary antibodies diluted in 5% dry milk or 3% BSA in TBS-Tween 0.1% (Supplementary Table 1). Membranes were washed 3 times, for 5 min each, with TBS-Tween 0.1% and probed with secondary antibodies in TBS-Tween 0.1% (Supplementary Table 1). The signal was obtained using Clarity™ Western ECL Blotting Substrate (BIO-RAD) and visualized using the ChemiDoc MP imaging system (BIO-RAD). The density of each band was normalized to the total proteins in the lane using ImageLab 5.2 software (BIO-RAD). Protein extracts from 9 randomly picked animals per group were analysed (n = 9).
The acquisition of microscopic images and analysis of immunofluorescence
Immunofluorescence staining and analyses were performed as previously described (Dianati and Plante, 2017). Briefly, tissue cryosections (7 µm) were fixed in 4% formaldehyde and blocked in 3% BSA dissolved in TBS-Tween 0.1%. Primary antibodies were diluted in TBS-Tween 0.1% solutions and sections were incubated for 60 min at room temperature (or overnight at 4 °C) with primary antibodies (Supplementary Table 2). Staining was followed by 3 washes (5 min each) with TBS-Tween 0.1%. Sections were incubated with the appropriate secondary antibodies (Supplementary Table 2). Staining was followed by another series of 3 washes. Nuclei were stained with 4′, 6-diamidino-2-phenylindole (DAPI), and slides were mounted with Fluoromount-G (Cedarlane, Burlington, Ontario, Canada). Immunofluorescence images were obtained with a Nikon A1R+ confocal microscopic laser equipped with a spectral detector and analyzed using NIS-elements software (version 4). P-β-catser675 signal was quantified using the area of the immunofluorescence staining relative to that of the nucleus (DAPI) staining for each image. Cryosections from 4 randomly picked animals per group were analysed (n = 4); for each animal, 4 random areas of the section were quantified.
Immunoprecipitation
Immunoprecipitation analyses were done as previously described (Dianati and Plante, 2017). Briefly, the lysates used for immunoprecipitation were prepared from flash-frozen tissue, as described in the western blot section. Immunoprecipitation was performed using the PureProteome™ Protein G Magnetic Bead System (LSKMAGG02) (Millipore, Etobicoke, Ontario, Canada); 200 µl of lysates containing a total of 500 µg protein were incubated overnight at 4 °C with slow agitation with the primary antibody (Supplementary Table 3). The next day, 50 µl of magnetic beads were added to the incubated lysate-antibody solution, and the bead-antigen-antibody complex was incubated at room temperature for 90 min with slow agitation. After washes, the bead-antibody-protein complexes were dissociated using 2 consecutive incubations with 20 µl of glycine 0.2M (pH 2.5) to remove the IgG. Immunoprecipitation was followed by western blot analysis (Supplementary Table 3), as described above, using immun-Blot® low fluorescence PVDF membranes (BIO-RAD). Membrane signals were detected using either Clarity™ Western ECL Blotting Substrate (BIO-RAD) for HRP antibodies, or directly observed using the ChemiDoc MP imaging system for fluorophores (BIO-RAD). Protein-protein interaction was quantified as a ratio of the signal of the interacting protein to the signal of the precipitated target using ImageLab 5.2 software (BIO-RAD). Mammary gland protein extract from 4 randomly picked animals per group were analysed (n = 4).
Masson’s trichrome staining
Cryosections of mammary glands (n = 4) were cut (7 µm) and kept at −80 °C. At the time of coloration, sections were retrieved from −80 °C and fixed in Bouin’s overnight. Sections were then sequentially stained with Weigert’s iron hematoxylin (10 min), Biebrich scarlet-acid fuchsin (10 minutes), phosphomolybdic-phosphotungstic acid (20 minutes) and aniline blue (5 min). Sections were washed with warm tap water for 10 min between each coloration step. Finally, the sections were treated with 1% acetic acid for 5 min, dehydrated in an alcohol series (95% and 100%), cleared in xylene for 5 min and mounted using Permount (Fisher Scientific, Burlington, Ontario). Cryosections from 4 randomly picked animals per group were analysed (n = 4); for each animal, ≥4 random areas of the section were observed.
Statistical analyses
All statistical analyses were done using GraphPad Prism, version 6.01. Normal distribution of each data set and the presence of outliers were verified with a D’Agostino and Pearson omnibus normality test and Grubbs’ test (ESD method), respectively. For the data with normal distribution (α = .05), p-value was calculated using a parametric 1-way ANOVA followed by Dunnett’s multiple comparison tests; otherwise, a nonparametric Kruskal-wallis followed by Dunn’s multiple comparison tests was used.
RESULTS
BFR Treatments Did Not Affect Markers of Milk Synthesis or Mammary Gland Function
Effects of the mixture of BFR on pregnancy outcomes, pup growth and development, as well as on pups’ mammary gland development, are reported elsewhere (Tung etal., 2016, 2017) or currently under investigation. Exposure to the BFR mixture had no effects on dams mammary gland or body weights (data not shown), or on the ratio of mammary gland to the body weight of the dams (Supplementary Figure 1A). Mammary gland structure also remained unchanged between control and BFRs treated animals (Supplementary Figure 1B). However, T4 serum levels were reduced in dams, suggesting potential effects on mammary gland signaling or protein expression (Tung etal., 2016). The mammary gland levels of β-casein, a major milk protein, (Supplementary Figure 1C), or of Stat5 and p-Stat5tyr694, the down-stream effector of the prolactin receptor/Jak2/Stat5 signaling pathway, were not affected in response to BFR treatments (Supplementary Figs. 1D and 1E). We also evaluated the effects of BFR on indicators of epidermal growth factor (EGF), E2, and thyroid hormone signaling pathways. No significant changes were observed in the levels of EGFR/ErbB-1, pEGFR (Tyr1068), ErbB2, ERα, or thyroid hormone receptors α or β in the treated groups compared with controls (Supplementary Figure 2).
Effects of BRF Exposure on β-Catenin Levels and Phosphorylation
We next investigated whether the dual roles of β-catenin as an adherens junction protein and/or in signal transduction were affected by treatment with BFR. Western blot analysis showed that the total protein levels of β-catenin remained unchanged after exposure to BFR (Figure 1A). Similarly, the levels of non-p-β-catSer45 and p-β-catTyr654 were not altered in the mammary glands from BFR treatment groups (Figs. 1B and D). Interestingly, we observed a significant down-regulation of approximatively 50% in p-β-catSer675 levels in animal treated with the low BFR dose (0.06 mg/kg/d) compared with control (Figure 1C). To investigate whether p-β-catSer675 down-regulation was associated with a decrease in the levels or change in the activity of Akt (protein kinase B) and glycogen synthase kinase 3beta (GSK-3β), kinases known to be involved in its early phosphorylation on serine (Ser) 552 and Ser33/Ser37/Thr41, respectively, we determined their total protein levels as well as the levels of phosphorylated forms by western blotting. Protein levels of Akt, p-AktSer473, GSK-3β, and p-GSK-3βSer9 were not altered by BFR exposure, suggesting that the activity of these kinases was not altered (Figs. 2A–D). To evaluate PKA activity, we quantified the levels of CREB and phospho-CREB, a direct target of PKA (Rosenberg etal., 2002). Although protein levels of PKA and CREB were unchanged, a significant decrease (approximately 40%) in p-CREB was observed in groups in the 0.06 and 20 mg/kg/d BFR treatment groups (Figs. 2F and G), suggesting a decrease in PKA activity.
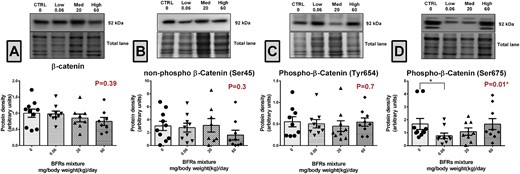
Effects of BFR treatments on the levels of β-catenin. Total proteins were extracted from the mammary glands of control dams or dams fed a BFR mixture diet formulated to deliver a daily nominal BFR mixture dose of 0.06, 20, or 60 mg/kg of body weight/day (mg/kg/d). Quantitative western blotting was done to evaluate β-catenin (A), non-p-β-catser45 (B), p-β-catser675 (C), and p-β-catTyr654 (D) protein expression. The columns represent the means ± SEM (n = 9) for each band normalized to the total protein level. p-values were calculated with an ANOVA (A) or a Kruskal-wallis (B–D) statistical tests.*p ≤ .05.
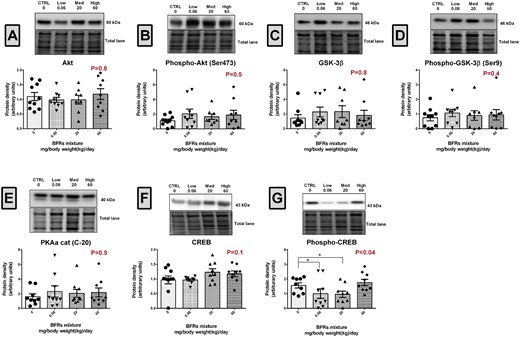
Effects of BFR treatments on the levels of Akt, GSK3β, PKA, CREB1, and their phosphorylated forms. Total proteins were extracted from the mammary glands of control dams or dams fed a BFR mixture diet formulated to deliver a daily nominal BFR mixture dose of 0.06, 20, or 60 mg/kg of body weight/day (mg/kg/d). Quantitative western blots were done to evaluate the expression of protein kinases involved in β-catenin phosphorylation Akt (A), Phospho-Akt (Ser473) (B), GSK-3β (C), Phospho-GSK-3β (Ser9) (D), and PKA (E), and of CREB (F) and phospho-CREB (G), a direct target of PKA. The columns represent the means ± SEM (n = 9) for each band normalized to the total protein level. p-values were calculated with a Kruskal-wallis (A, C–G) or an ANOVA (B) statistical tests. *p ≤ .05.
β-Catenin Signaling Is Not Affected by BFR Exposure
Previous studies have shown that phosphorylation of β-catenin at Ser675 increases its binding to transcriptional co-activators and promotes TCF/LEF-dependant gene transcription (Taurin etal., 2006). We examined the interaction of p-β-catSer675 with Lef1 and expression of their downstream targets to investigate whether p-β-catSer675 down-regulation is associated with a change in the β-catenin/Lef1 signaling pathway (Figure 3). Surprisingly, immunoprecipitation analysis revealed no interaction between p-β-catSer675 and Lef1 in control or treated animals (Figure 3A). Consistently, analysis of TCF/LEF-dependant gene expression showed that BFR exposure did not alter the expression of TCF1, c-Jun, c-Myc or Met in the rat mammary gland (Figs. 3B–E).
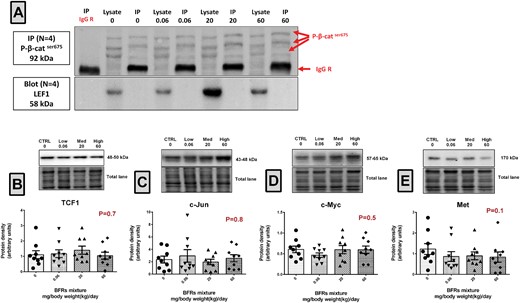
Evaluation of β-catenin/Lef1 interaction and of the levels of their downstream target genes. A, p-β-catser675 was immunoprecipitated using total lysates from mammary glands from control and BFR exposed animals. Biological replicates (n = 4) were processed at the same time but loaded and transferred on different gels/blots when required. Membranes were probed with p-β-catser675/Alexa rabbit 488 and LEF1/Veriblot HRP consecutively. B–E, Total proteins were extracted from the mammary glands from control, low, medium, high dose treated dams. Quantitative western blots were done to evaluate downstream targets of β-catenin-Lef1; TCF1 (B), c-Jun (C), c-Myc (D), and Met (E). The columns represent the means ± SEM (n = 9) for each band normalized to the total protein level. p-values were calculated with a Kruskal-wallis statistical test.
Endogenous p-β-catSer675 Is Localized at the Cell Membrane
Because our results did not show alterations in the expression of known β-catenin downstream targets (Figs. 3B–E), we compared the localisation of β-catenin and p-β-catSer675 in mammary glands from control and BRF exposed dams using confocal immunofluorescence. In control animals, endogenous p-β-catSer675 resides at basolateral and apical areas of the membrane where it co-localizes with β-catenin (Figure 4A). Interestingly, in animals exposed to the 0.06 and 20 mg/kg/d BFR mixtures, a lower expression of p-β-catSer675 was observed (Figs. 4B, C, and E). In animals from the highest dose treatment groups, a nonsignificant decrease was observed (Figs. 4D and E).
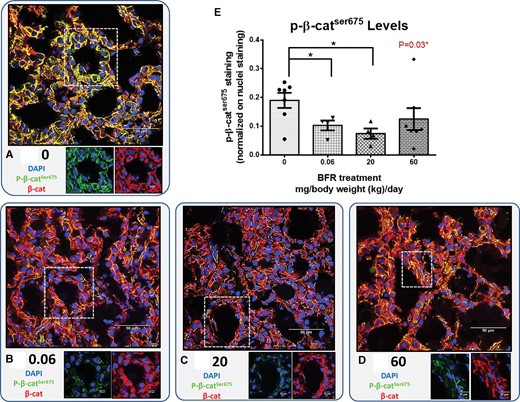
Localization of endogenous β-catenin and p-β-catser675 in mammary glands from the dams. Cryosections were cut (7 µm) and processed for immunofluorescence staining. A–D, Nuclei were stained with DAPI (blue). β-catenin (red) and p-β-catser675 (green) co-localized at the membrane, and the levels of p-β-catser675 differed dramatically in control and BFR-exposed animals. Images were obtained with a Nikon A1R+ equipped with a spectral detector and analyzed using NIS-elements software. E, The columns represent the means ± SEM (n = 4–7) for p-β-catser675 staining normalized to nuclei staining. p-values were calculated with a Kruskal-wallis statistical test. *p ≤ .05.
Expression of Adherens, Tight and Gap Junction Proteins and of Markers of the EMT Were Not Altered by Exposure to BFR
Since the localization of p-β-catSer675 at the cell membrane of epithelial cells was decreased in animals from the 0.06 and 20 mg/kg/d BFR treatment groups, we investigated whether other junctional proteins or markers of EMT were affected by the treatment. The expression levels of vimentin and N-cadherin, markers of mesenchymal cells, (Supplementary Figs. 3A and B), of E- and P-cadherin, adherens junction proteins expressed in luminal and myoepithelial cells, respectively (Supplementary Figs. 3C and D), of claudin-7 and occludin, components of tight junctions (Supplementary Figs. 3E and F), and of connexin 43 (Cx43) and connexin 32 (Cx32), constituents of gap junctions (Supplementary Figs. 3G and H), were not different between control and BFR-exposed rats. The localisations of E-cadherin, vimentin, Cx43, and Cx32 were also not affected (Supplementary Figure 4).
Effects of Exposure to BFR on the Interaction of p-β-catser675 With E-Cadherin
We investigated the interaction of p-β-catSer675 with E-cadherin, the marker for adherens junctions, since the localization of p-β-catSer675 at the cell membrane of epithelial cells was decreased in animals from the 0.06 and 20 mg/kg/d BFR treatment groups. Co-immunoprecipitation analysis showed that while E-cadherin was equally precipitated in control and treated animals (Figs. 5A top panel and B), the interaction of β-catenin with E-cadherin tended to be lower (p = .07) in the mammary glands of animals from the 0.06 mg/kg/d treatment group (Figure 5C). Interestingly, our results demonstrated that in this treatment group, the p-β-catSer675/E-cadherin interaction was significantly decreased (Figure 5D); a nonstatistically significant suppression was also observed in animals from the 20 and 60 mg/kg/d BFR treatment groups (Figure 5D). Interaction between p-β-catSer675 and E-cadherin was confirmed by performing the reciprocal co-IP (Supplementary Figure 5).
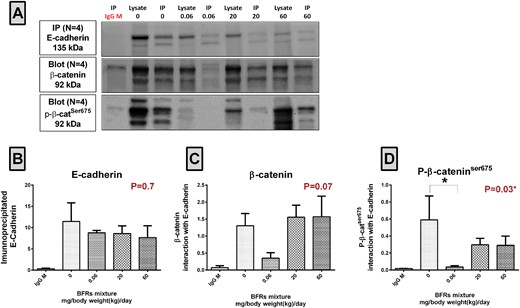
Analysis of the interactions between β-catenin and E-cadherin and between p-β-catser675 and E-cadherin. A, E-cadherin was immunoprecipitated using total lysates from mammary glands from control dams and dams fed a BFR mixture delivering nominal doses of 0.06, 20, and 60 mg/kg/d. Biological replicates (n = 4) were processed at the same time but loaded and transferred on different gels/blots when required. Membranes were probed with E-cadherin/Alexa mouse 647, p-β-catser675/veriblot HRP, and β-catenin/Alexa mouse 488 consecutively. B, The protein density of precipitated E-cadherin. C, β-catenin/E-cadherin protein interactions calculated as the ratio of β-catenin to E-cadherin. D, p-β-catser675/E-cadherin protein interactions calculated as a ratio of p-β-catser675 to E-cadherin. The columns represent the means ± SEM; P-values with a Kruskal-wallis statistical test. *p ≤ .05.
DISCUSSION
Because mammary gland development requires precise hormonal regulation and environmental endocrine disruptors may disturb this process, here, we investigated the effect(s) of an exposure to an environmentally relevant BFR mixture of during pregnancy and lactation on mammary gland development and function. Interestingly, we found that p-β-catSer675 levels were decreased in dams from the low dose, environmentally relevant, BFR treatment group. Furthermore, p-β-catSer675 was localized to the cell membrane in the lactating gland. An inhibition of the interaction of p-β-catSer675 with E-cadherin was observed that is likely linked with an effect on PKA activity as levels p-CREB, a direct target of PKA, were also down-regulated.
Although several studies have examined the effects of in utero and early life exposures to PBDEs and HBCDD on a range of aspects of growth and development, only one determined the effects on mammary gland development in female offspring (Kodavanti etal., 2010); in this study, exposure was to a technical mixture, DE-71. To the best of our knowledge, our research is the first to evaluate the effects of BFR exposure during gestation and lactation on mammary gland function and development in dams. Previously, we reported that exposure to the environmentally relevant mixture of BFR found in house dust in utero and during lactation decreased T4 levels, but had no impact on the weights of offspring at the time of weaning, although at later ages body weights were significantly reduced in the 2 highest dose treatment groups (Tung etal., 2016). This observation would suggest that exposure to BFR does not affect the lactation capacity of the dams, but could still dysregulate signaling pathways. We did not have observe treatment-related changes in tissue structure and the protein levels of a broad array of markers of milk synthesis (β-casein) or in measures of hormonal responsiveness (total or phosphorylated Stat5, ER alpha or thyroid hormone receptors), EFG signaling (EGF receptors ErbB-1 and 2, phosphor-ErbB1), adhesion proteins (N-, P- or E-cadherin), intermediate filaments (vimentin), tight junctions (claudin-7, occludin) or gap junctions (Cx32 and Cx43). However, β-catenin activation (p-β-catSer675) and interaction with E-cadherin were perturbed by low dose treatment with BFR.
p-β-catSer675 Expression and Interaction With E-Cadherin Were Perturbed by Exposure to BFR
Exposure to the BFR mixture caused a reduction in the levels of p-β-catSer675 immunoreactivity in the dam mammary gland. Notably, exposures to the lowest dose of BFR had the greatest effect, with a reduction in mean levels to roughly 50% of control, measured using 2 different assays. In dams receiving the intermediate dose, the decrease in p-β-catSer675 was significant only when immunofluorescence images were quantified. In dams exposed to the highest dose, p-β-catSer675 levels were not different from those observed in control samples. β-catenin phosphorylation at other residues was not altered by BFR exposure. Similarly, the levels of p-β-catSer675 associated with immunoprecipitated E-cadherin were more dramatically reduced in mammary glands from dams from the lowest dose treatment groups, whereas samples from dams receiving the higher doses did not differ from control.
It has been suggested that exposure to endocrine disruptors in sensitive periods of life can promote breast cancer (Cohn etal., 2012; Fenton, 2006). Interestingly, higher levels of polychlorinated biphenyls in early postpartum serum samples in women were correlated with a 6-fold increased risk of breast cancer in women (Cohn etal., 2012). In our study, we found a significant decrease in β-catenin phosphorylation at Ser675 and a reduced association with E-cadherin but we did not observe a change in β-catenin levels or localisation. Loss of cadherin-bound β-catenin is correlated significantly with poor clinical outcome in breast cancer (Dolled-Filhart etal., 2006); perturbing the role of β-catenin in signal transduction leads to precocious alveologenesis, adenocarcinoma, hyperplasia, hyperbranching and lactation defects (Incassati etal., 2010; Tepera etal., 2003; Teuliere etal., 2005). Interestingly, exposure to the lowest doses of BFR significantly downregulated the p-β-catSer675 levels in dams in the absence of effects on overall β-catenin levels. The consequences of reduced p-β-catser675 for the function and long term health of the lactating mammary gland is not clear. Thus, whether or not these modulations are associated with increased risk of breast cancer remains to be evaluated.
β-Catenin possesses many putative phosphorylation sites. Phosphorylation at Ser33, Ser37 and Thr41 by GSK3-β, and at Ser45 by the priming kinase CKια, increases ubiquitination and degradation by proteasomes (Liu etal., 2002). Alternatively, phosphorylation at Tyr654 increases the binding of β-catenin to E-cadherin in adherens junctions (Roura etal., 1999). Phosphorylation of β-catenin at Ser675 is less well studied. However, it has been demonstrated that Ser675 phosphorylation prevents β-catenin degradation and thereby promotes its transcriptional activity (Spirli etal., 2013; Taurin etal., 2006).
Consequently, we investigated the protein levels of PKA, Akt and GSK-3β, kinases that are linked to β-catenin phosphorylation (Fukumoto etal., 2001; Gordon and Nusse, 2006; Spirli etal., 2013; Taurin etal., 2006). Phosphorylation of Akt and GSK3β in BFR treatment groups did not differ from control; although phosphorylation of these kinases is commonly considered as evidence of their activation status, more studies are required to determine whether the activities of those kinases are truly unchanged. Since PKA levels are not indicative of its activation we examined the phosphorylation of CREB, a direct target of PKA (Rosenberg etal., 2002). Levels of p-CREB were reduced in animals from the 0.06 and 20 mg/kg/d treatment groups, suggesting that treatment with BFR indeed led to an inactivation of PKA that may explain the decrease in p-β-catSer675 levels that we observed. Interestingly, alterations of the CREB family of transcription factors are observed in hormono-dependent organs, and their dysregulation are observed in tumors (Rosenberg, etal., 2002).
Since β-catenin transcriptional activation is associated with phosphorylation at Ser675 and transcriptional activity of β-catenin requires its nuclear localisation (Estus etal., 2016; Spirli etal., 2013; Taurin etal., 2006), we examined whether there was a change in the localisation of this protein after treatment with BFR. We observed a treatment-related reduction in p-β-catSer675 immunoreactivity but did not see any apparent decrease in the nuclear localization of p-β-catSer675 in mammary gland epithelial cells. Furthermore, our results showed no interaction between p-β-catSer675/Lef1, and no changes in the expression of recognized downstream targets (Boon etal., 2002; Mann etal., 1999; Shtutman etal., 1999), suggesting that β-catenin signaling is not affected by exposure to BFR.
To further confirm the localisation and involvement of p-β-catSer675 at cell membrane, we performed an immunoprecipitation assay with E-cadherin, the adherens junction component that interacts with β-catenin at the membrane of luminal epithelial cells. Our co-immunoprecipitation assays demonstrated an interaction between E-cadherin and p-β-catSer675, thus confirming that β-catSer675 was indeed located at cell membrane. Moreover, a significant decrease in E-cadherin/p-β-catSer675 interactions was observed specifically in the mammary glands from dams in the lowest dose BFR treatment group. It is interesting that changes in p-β-catSer675 expression in the mammary glands of BFR exposed dams were observed most consistently in our lowest dose treatment groups. Nonmonotonic dose response curves and low-dose effects have been reported previously for endocrine active chemicals (Vandenberg etal., 2012)).
Our data suggesting that exposures during pregnancy may influence the function of the maternal mammary gland in a manner that may have consequences for the long-term health sheds light on a very understudied issue. Pregnancy and lactation clearly influence the likelihood of developing mammary gland cancers but there have been very few studies that have probed the possibility that events and exposures during pregnancy can modify this risk. Although we were not able to follow up these observations in this study, our data informs this hypothesis and may encourage others to examine this possibility.
CONCLUSION
The exposure of female rats during pregnancy and lactation to a mixture of BFR representative of those found in house dust significantly reduces the level of p-β-catSer675 expression in the mammary glands. This decrease is likely to be PKA-dependent manner and is accompanied by a decrease in p-β-catSer675/E-cadherin interaction at the cell membrane. Although there were no apparent adverse effects on the structure or function of the mammary gland during pregnancy and lactation, these effects may have significant consequences on signal transduction linked to PKA activity. Since the role of endogenous p-β-catSer675 is not yet fully understood, further investigations should establish its role in normal mammary gland development and the consequences of its dysregulation on involution and in long-term on breast cancer.
SUPPLEMENTARY DATA
Supplementary data are available at Toxicological Sciences online.
ACKNOWLEDGMENTS
We thank Claudia Lalancette, Pavine Lefèvre, Emily Tung, Alice Kawata, Marc Ridgen, and Martine Caplette for their technical assistance.
FUNDING
This research was funded by Canadian Institutes of Health Research (CIHR), Institute for Human Development, Child and Youth Health (RHF No. 100625 to B.F.H. and C.G.G.), Health Canada (M.G.) and a Natural Sciences and Engineering Research Council of Canada grant (NSERC No. 418233–2012 to I.P.). BFH and BR are James McGill Professors. I.P. is the recipient of a Fonds de Recherche du Québec-Santé (FRQS) and Quebec Breast Cancer Foundation career award, and a Leader Founds from the Canadian Foundation for Innovation. E.D. received a scholarship from the Fondation Universitaire Armand-Frappier.
REFERENCES
Comments