-
PDF
- Split View
-
Views
-
Cite
Cite
Amanpreet Kaur, Norman B Best, Thomas Hartwig, Josh Budka, Rajdeep S Khangura, Steven McKenzie, Alejandro Aragón-Raygoza, Josh Strable, Burkhard Schulz, Brian P Dilkes, A maize semi-dwarf mutant reveals a GRAS transcription factor involved in brassinosteroid signaling, Plant Physiology, Volume 195, Issue 4, August 2024, Pages 3072–3096, https://doi.org/10.1093/plphys/kiae147
- Share Icon Share
Abstract
Brassinosteroids (BR) and gibberellins (GA) regulate plant height and leaf angle in maize (Zea mays). Mutants with defects in BR or GA biosynthesis or signaling identify components of these pathways and enhance our knowledge about plant growth and development. In this study, we characterized three recessive mutant alleles of GRAS transcription factor 42 (gras42) in maize, a GRAS transcription factor gene orthologous to the DWARF AND LOW TILLERING (DLT) gene of rice (Oryza sativa). These maize mutants exhibited semi-dwarf stature, shorter and wider leaves, and more upright leaf angle. Transcriptome analysis revealed a role for GRAS42 as a determinant of BR signaling. Analysis of the expression consequences from loss of GRAS42 in the gras42-mu1021149 mutant indicated a weak loss of BR signaling in the mutant, consistent with its previously demonstrated role in BR signaling in rice. Loss of BR signaling was also evident by the enhancement of weak BR biosynthetic mutant alleles in double mutants of nana plant1-1 and gras42-mu1021149. The gras42-mu1021149 mutant had little effect on GA-regulated gene expression, suggesting that GRAS42 is not a regulator of core GA signaling genes in maize. Single-cell expression data identified gras42 expressed among cells in the G2/M phase of the cell cycle consistent with its previously demonstrated role in cell cycle gene expression in Arabidopsis (Arabidopsis thaliana). Cis-acting natural variation controlling GRAS42 transcript accumulation was identified by expression genome-wide association study (eGWAS) in maize. Our results demonstrate a conserved role for GRAS42/SCARECROW-LIKE 28 (SCL28)/DLT in BR signaling, clarify the role of this gene in GA signaling, and suggest mechanisms of tillering and leaf angle control by BR.
Introduction
Plant architecture is a key determinant of plant fitness and crop yield. Above-ground plant architecture influences plant competition, light harvesting, and reproductive capacity. In an agricultural context, manipulating plant height and leaf angle are effective strategies to improve crop yields per unit of land area (Lambert and Johnson 1978; Duvick 2005; Salas Fernandez et al. 2009; Liu et al. 2017; Paciorek et al. 2022). A notable example of this is the tremendous increase in wheat (Triticum aestivum) and rice (Oryza sativa) yields achieved during the 20th century by growing semi-dwarf lodging-resistant varieties (Khush 2001). In maize (Zea mays), manipulation of leaf angle has contributed to yield increases by facilitating greater plant densities per unit area (Lambert and Johnson 1978; Liu et al. 2017). Efforts are underway to deploy semi-dwarf maize using the brachytic2 gene (Bage et al. 2020; Patent 10472684). A comprehensive understanding of the genetic and molecular mechanisms controlling architectural traits will facilitate breeding efforts to improve crop productivity and provide insights into the mechanisms controlling these traits in natural systems.
In maize, above-ground architecture is primarily defined by plant height, ear height, and leaf architecture. Plant height and leaf architecture are regulated by phytohormones, including gibberellins (GAs), brassinosteroids (BRs), and auxins. Maize dwarf mutants have been characterized with defects in GA biosynthesis or signaling, including dwarf1 (d1), dwarf3 (d3), dwarf5 (d5), and anther ear1 (an1) encoded by recessive loss-of-function alleles in biosynthetic enzymes as well as D8 and D9 encoded by semi-dominant alleles in the DELLA-domain GRAS transcription factors that regulate GA-associated gene expression (Winkler and Freeling 1994; Bensen et al. 1995; Winkler and Helentjaris 1995; Chen et al. 2014; Fu et al. 2016). These mutations result in short stature, increased tillering, and the retention of anthers in the ear florets. Other dwarf mutants, originally called nana plants (na) (Hutchison 1922; Lindstrom 1923; Li 1933), result from BR-deficiency due to loss-of-function mutations in biosynthetic enzymes including nana plant1 (na1), nana plant 2 (na2), and brassinosteroid-deficient dwarf1 (brd1) (Hartwig et al. 2011; Makarevitch et al. 2012; Best et al. 2016). These BR-deficient mutants exhibit short stature, dark green and erect leaves, reduced tillering, and the presence of pistils in the tassel florets. An additional class of dwarf mutants, called brachytic (br), includes the br2 mutant defective in the P-glycoprotein1 ABC transporter (Multani 2003). Other maize mutants defective in auxin production have more pleiotropic phenotypes, such as vanishing tassel 2 (vt2), which are semi-dwarf, have a reduced number of leaves, and a severe barren tassel phenotype (Phillips et al. 2011).
Several genes regulating leaf angle have been identified in maize via mutant analysis. The classical maize genes liguleless1 (lg1), liguleless2 (lg2), liguleless3 (lg3), and rough sheath1 (rs1) regulate leaf angle by establishing the blade/sheath boundary (Becraft and Freeling 1994; Fowler and Freeling 1996; Moreno et al. 1997; Walsh et al. 1998; Muehlbauer et al. 1999). Mutations in an APETALA2 (AP2) transcription factor, DWARF & IRREGULAR LEAF (DIL1), lead to dwarf stature and erect leaves (Jiang et al. 2012). The role of BRs in determining leaf angle has been elucidated in Arabidopsis (Arabidopsis thaliana), rice (Li et al. 2020; Cao et al. 2022), and in BR loss-of-function mutants of maize including loss of na1, na2, and brassinosteroid-deficient dwarf1 (brd1), as well as RNAi of brassinosteroid insensitive1(zmbri1), which all display an erect leaf phenotype indicating a conserved role of BRs in modulating leaf angle (Hartwig et al. 2011; Makarevitch et al. 2012; Kir et al. 2015; Best et al. 2016). Remarkably, many of these genes have subsequently been identified as candidates for natural variation in leaf angle, including lg1, lg2, and brd1 (Tian et al. 2019). In addition to finding alleles in genes identified by classical mutagenesis studies, a number of QTLs have been molecularly identified and subsequently linked to these pathways. For example, an allele of abi2-vp1-transcription factor12/rav-like1 (abi12/zmravl1) alters leaf angle by regulating the BR pathway in maize (Tian et al. 2019). Although direct interaction of LG1 with the BR pathway has not been established, DROOPING LEAF1 (DRL1) interacts with LG1 in vitro, which may ultimately repress BRD1 expression and reduce BR levels and leaf angle (Tian et al. 2019). A recent study showed that ZmLG2 targets key transcription factors required for BR signaling, BZR1 (BRASSINAZOLE RESISTANT1), and ZmBEH1 (BZR1/BES1 HOMOLOG GENE1), which further bind to the promoter of a GRAS domain transcription factor, GRAS42 (also known as SCARECROW-LIKE 28 after the name of the ortholog in Arabidopsis thaliana), to regulate leaf angle (Wang et al. 2022). BRs also interact with other phytohormones to control leaf angle (Best et al. 2016). GA application increases leaf angle in na2-1 mutants but not in the wild-type controls, indicating that GA controls leaf angle via an interaction with the BR pathway (Best et al. 2016).
Studies in Arabidopsis have characterized the BR biosynthesis and signaling pathways (Clouse 2011; Chung and Choe 2013; Kim and Russinova 2020). The identification of homologs of several Arabidopsis BR signaling genes in rice suggests conserved mechanisms of BR signaling but our knowledge of BR signaling components in monocots is still limited (Zhang et al. 2014; Kir et al. 2015). In addition, several BR signaling components like DWARF AND LOW TILLERING (DLT), BRASSINOSTEROID UPREGULATED1 (BU1), and TAIHU DWARF1 (TUD1) have been identified through genetic studies in rice (Tanaka et al. 2009; Tong et al. 2009, 2012; Hu et al. 2013; Zhang et al. 2014). Identification of gene functions can help us better understand BR signaling, and the recovery of different genes from mutant screens in monocots as compared to Arabidopsis raises the possibility of distinct signaling mechanisms in monocots. These components have identified additional interactions between hormone pathways. For instance, studies in rice have shown the role for interactions between auxin and BR in leaf angle control, whereas mutants in the auxin-regulated SMALL ORGAN SIZE1 (SMOS1) and DLT genes display an epistatic interaction in rice and DLT is required for SMOS1 overexpression to influence plant height and culm width (Hirano et al. 2017; Qiao et al. 2017).
The rice DLT gene is a GRAS family transcription factor that acts as a positive regulator of BR signaling (Tong et al. 2009). Rice dlt mutants are semi-dwarfs with a reduced number of tillers and short, wide, and more upright leaves. BR biosynthetic gene expression is increased in the dlt mutant indicating that DLT is a negative regulator of BR biosynthesis (Tong et al. 2009). The mutants also show altered accumulation of transcripts of BR-regulated genes consistent with the role of DLT in BR signaling. The transcription of DLT is regulated by a direct interaction of BZR1 with the DLT promoter (Tong et al. 2009). At the protein level, DLT is regulated by GLYCOGEN SYNTHASE KINASE2 (GSK2) that phosphorylates both DLT and BZR1 and controls downstream BR signaling (Tong et al. 2012). DLT has also been reported to modulate GA levels in rice (Li et al. 2010). The d62 mutant allele of dlt in rice showed high expression of GA biosynthetic genes and low endogenous GA levels suggesting that DLT plays a role in regulating both hormonal pathways and bridges GA-BR regulation to alter plant architecture. Mutants in the Arabidopsis ortholog of DLT, SCL28, dramatically altered cell size, the progression through G2/M phase of cell cycle in root meristems, and the selection of cell division planes (Goldy et al. 2021). A study of maize mutants in the DLT ortholog, gras42, also found the mutants had more upright leaves and determined that maize BZR/BEH homologs bind to the gras42 promoter suggesting that gras42 acts downstream of BR perception (Wang et al. 2022).
In this study, we identified multiple mutant alleles of the maize ortholog of the rice DLT gene, gras42. These mutants share a semi-dwarf, reduced tillering, and erect leaves phenotype. We studied the expression consequences of loss of gras42 to explore its role in BR and GA signaling. While gras42 acted as a positive regulator of BR-responsive gene expression, it suppressed GA-induced gene expression, and these effects were found to be tissue-specific. A Genome-wide Association study (GWAS) of natural variation in GRAS42 expression identified known BR pathway genes as regulators of gras42 expression.
Results
Isolation of a dwarf and low tillering mutant of maize
The gras42 gene (Yilmaz et al. 2009) encodes a maize homolog of the rice gene DLT, which was identified based on a semi-dwarf low-tillering phenotype (Tong et al. 2009). Maize gras42 (gene model v5 Zm00001eb378590; v4 Zm00001d045507; v3 AC234164.1_FG004) is found in a syntenic genomic context in maize, rice, and sorghum (Sorghum bicolor) and is sister to the AT1G63100/AtGRAS-8/SCARECROW-LIKE 28 (SCL28) gene of Arabidopsis in a prior phylogenetic analysis (Guo et al. 2017). A gene disruption in the coding sequence resulting from the insertion of a Mutator (Mu) element was sequenced as mu10211049 (NCBI accession HQ135270, GI:308313849) as part of the Uniform-Mu project (McCarty et al. 2005), and is available through the Maize COOP as gras42-mu1021149 (Fig. 1). The Mu allele, gras42-mu1021149, encodes a recessive self-fertile, semi-dwarf mutant with upright crinkled leaves (Fig. 1). Backcrossing the mutant to A619, A632, B73, and W22 resulted in wild-type plants in the F1 and a 3:1 segregation in the F2 demonstrating that this mutant phenotype is encoded by a single recessive locus. A similar phenotype was identified segregating as a single monogenic recessive trait among families of the CML247 inbred line (Hartwig 2011). Crosses between the gras42-mu1021149 and semi-dwarf CML247 individuals failed to complement gras42-mu1021149 indicating that these were allelic mutants (Hartwig 2011). A third line with similar phenotype was identified in a W22 EMS family generated by Dr. Clifford Weil (03INW22CW0578) among the 2009 field season of the TILLInG project at Purdue University (Till et al. 2004; Weil and Monde 2007). All crosses between these three mutants failed to complement, indicating the phenotypes were recessive and due to alleles at the same gene. Sequencing of the gras42 allele in the TILLInG line identified a change from tryptophan to stop codon at the 74th codon. The mutator allele was previously described as brachytic crinkled leaf1 (bcr1) (Hartwig 2011) and upright leaf angle1-1 (url1-1) and was also described by Wang et al. (2022), who referred to it as scl28-1 after the Arabidopsis ortholog, along with a second allele scl28-2 (gras42-2), both of which caused upright leaf angles. We therefore refer to the spontaneous allele from CML247 as gras42-3 and the EMS allele (03INW22CW0578) as gras42-4.
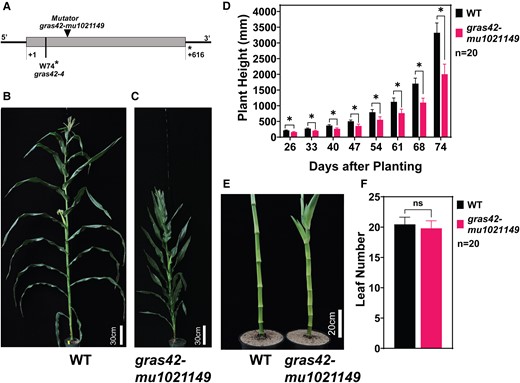
Phenotypic characteristics of gras42-mu1021149A) Structure of the gras42 locus (Zm00001d045507) and mutant alleles. Solid gray box depicts the exon. Solid black lines depict 5′ and 3′ untranslated regions. Mutant allele gras42-mu1021149 is depicted by a triangle at the site of Mutator transposable element insertion and gras42-4 by a dashed line showing a premature stop codon resulting from a single-nucleotide substitution (G221A). Asterisk indicates a stop codon. First and last amino acid positions of GRAS42 are indicated; B) Mature gras42-mu1021149 heterozygote (gras42-mu1021149/+; wild type; WT); C) Mature gras42-mu1021149 homozygote; D) Plant height of WT and gras42-mu1021149. Error bars are ± SD. Asterisk indicates significant difference between WT and gras42-mu1021149 determined by Student's t-test (P < 0.000001); E) Stem and internodes in WT and gras42-mu1021149; F) Number of leaves in WT and gras42-mu1021149 siblings. Error bars are ± SD. Significant differences were determined using Student's t-test. ns indicates difference is not significant.
Loss of gras42 results in short plants with upright and wide leaves
The phenotype of gras42-mu1021149 was investigated in a segregating F2 population. The mutants were discernibly different in height from their wild type (WT, gras42-mu1021149/+) siblings 26 d after sowing (DAS). This difference in plant height got progressively greater until maturity when homozygous mutants were approximately 60% the height of their wild-type siblings (Fig. 1). The reduced height of the mutant was driven entirely by changes in the internode length, as no differences in node number were noted at maturity (Fig. 1). In addition, the leaves of gras42-mu1021149 were significantly shorter and wider as compared with wild-type siblings (Fig. 2). Consistent with the overall upright habit of the mutant plants, the leaf angle was more upright and significantly different from wild type for all leaves above the ear node (Fig. 2).

Leaf morphology of gras42-mu1021149. A) Leaf size of fully extended leaf 15 in heterozygote (WT) and homozygote gras42-mu1021149; B) Width of leaves in WT and gras42-mu1021149; C) Length of leaves in WT and gras42-mu1021149 siblings; D) Fully extended leaf 10 in WT; E) Fully extended leaf 10 in gras42-mu1021149; F) Leaf angle (leaf 10) of gras42-mu1021149 heterozygote and homozygote in degrees (°). The numbers of leaves were counted starting from the base of the plant with the first developing true leaf counted as one. In bar plots, error bars are ± SD. Asterisk indicates significant difference between WT and gras42-mu1021149 determined by Student's t-test (P < 0.0001).
Expression of GRAS42 in wild-type and gras42-mu1021149 mutant plants
We analyzed publicly available single-cell transcriptomic data from maize vegetative shoot apices (Satterlee et al. 2020) to identify cell clusters enriched for GRAS42 transcripts. Two-cell populations exhibited high accumulation of GRAS42 transcripts (Fig. 3). Using 23 known genes involved in S phase and 190 genes involved in G2/M phase of cell division, clusters of cells corresponding to different phases of cell cycle were marked. The two cell clusters, where the expression of GRAS42 was the highest, were both comprised of G2/M cells (Fig. 3). Further exploration of the two G2/M clusters with high expression of GRAS42 revealed that one of these clusters was enriched in epidermal cells as evidenced by expression of epidermal marker OUTER CELL LAYER4 (OCL4; Zm00001eb024680) (Fig. 3). Within the epidermal marked cells, maize stomatal lineage markers TOO MANY MOUTHS-LIKE1 (TMM1; Zm00001eb159500) and SPEECHLESS2 (ZmSPCH2; Zm00001eb204110) are hypothesized to mark the maize stomatal lineage in all three phases of the cell cycle (Supplementary Fig. S1). GRAS42 expression appears to overlap with these stomatal markers in the epidermal G2/M cell population but is even more differentiated indicating that it is likely expressed during cell divisions that potentially include those leading to stomata. This result was consistent with previously reported investigations of the Arabidopsis homolog, SCL28, in roots where expression occurred in G2/M cells and mutants had defects in meristematic epidermal cell walls (Goldy et al. 2021). These results suggest that the consequences of the gras42-mu1021149 mutant on gene expression should be greatest in RNA samples extracted from tissues that contain a high proportion of actively dividing cells.
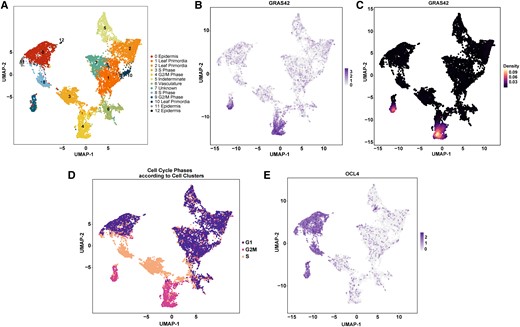
Uniform manifold approximation and projection (UMAP) plots for expression in published single-cell transcriptomic data (Satterlee et al. 2020). A) Clusters of cells for different cell types. UMAP identified 13 clusters numbered from 0 to 12 and coded by different colors; B) Expression of gras42 in cell clusters; C) Density plot for gras42;D) Cell cycle phases assessed by gene co-expression; E) Expression of ocl4 in cell clusters.
We performed RNA sequencing on heterozygous and mutant siblings from an F1 family of a gras42-mu1021149/+ x gras42-mu1021149/gras42-mu1021149 cross. Seedlings were grown to the V3 stage (Abendroth et al. 2011) and RNA was extracted from three separate tissue collections: shoot apices including stem and SAM tissue; expanding leaf sheaths; and the base of the third leaf blade (L3 base). As expected from the single-cell analysis, the abundance of the GRAS42 transcript varied across these three tissues with the highest abundance in the actively dividing shoot apices (Fig. 4) and no expression was detected in RNA extracted from the base of the mature collared L3. These results are similar to DLT expression studies in rice where actively dividing and elongating tissues showed the highest expression of DLT transcripts (Tong et al. 2009).
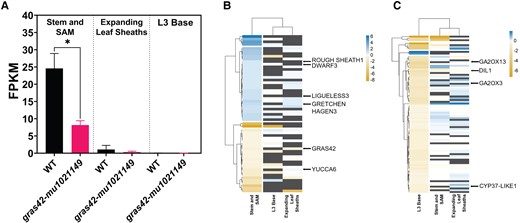
Transcriptomic analysis of gras42-mu1021149 vs WT (gras42-mu1021149/+). A) Expression levels (FPKM) of gras42 gene in WT (gras42-mu1021149/+) and gras42-mu1021149 as detected from RNA sequencing in three tissues: stem and shoot apical meristem (SAM), expanding leaf sheaths and base of third leaf (L3 base). Error bars are ± SD, n = 3. Asterisk indicates significant difference between WT and gras42-mu1021149 determined by Student's t-test (* = P < 0.05); B) Heatmap showing log2 fold change of 58 genes differentially expressed in gras42-mu1021149 stem and SAM tissue across the three tissue types. Blue color indicates genes induced in gras42-mu1021149, and gold color represents genes repressed in gras42-mu1021149 vs WT. Dark gray color represents the genes that were not present in the specific tissue; C) Heatmap showing log2 fold change of 85 genes differentially expressed in gras42-mu1021149 L3 base compared to wild type across the three tissue types.
The Mu insertion in gras42-mu1021149 dramatically decreased GRAS42 accumulation in stem and SAM tissue relative to the wild type. We examined the reads in the mutant and wild-type samples and identified that the mutant accumulated transcripts from the transcriptional start site up to the location of the Mu element insertion in gras42-mu1021149, corresponding to the first third of gene (Supplementary Fig. S2). Some reads did map to the region of the gras42 gene downstream of the Mu insertion but accumulated to a much lower level than seen at the 5′ end upstream of the Mu insertion. Furthermore, no junction reads spanning the insertion or read pairs corresponding to a contiguous transcript were identified, suggesting that these 3′ reads derived from promoter activity encoded by the Mu element. This indicates that no full-length GRAS42 protein can be encoded by these transcripts. Similar transcript accumulation patterns on either side of the transposon insertion have been observed at other Mu insertions (Ellison et al. 2023). Similar to the effect of the Mu insertion on expression in the stem and SAM sample, GRAS42 transcripts in RNA from expanding leaf sheaths displayed a greater proportion of the reads derived from the sequence at 5′ to the Mu insertion than the 3′ end (Supplementary Fig. S3).
A paralog of gras42 in maize
A search for homologs of gras42 at PLAZA Monocots 5.0 database identified a paralog in maize, gras47 (Zm00001d041498). Prior phylogenetic studies identified this paralog as also being sister to SCL28 in Arabidopsis and DLT in rice (Guo et al. 2017), suggesting a duplication of this gene in the lineage leading to maize. We performed a phylogenetic analysis of gras42 homologs in amborella (Amborella trichopoda), asparagus (Asparagus officinalis), garlic (Allium sativum), Arabidopsis, brachypodium (Brachypodium distachyon), rice, setaria (Setaria italica and Setaria viridis), sorghum, and maize. The two paralogs in maize resulted from a maize-lineage duplication that occurred after the divergence of maize and sorghum (Supplementary Fig. S4). We did not detect GRAS47 transcripts in the wild-type samples in our RNA-seq experiment. In addition, we observed no compensatory increase in the expression level of gras47 in the gras42-mu1021149 mutant. In addition, transcripts from gras47 were not detectably expressed (Supplementary Table S1) in 167 of the 173 RNA-seq experiments available in the gene expression database qTeller (Woodhouse et al. 2021b). For experiments in qTeller where both genes were expressed, gras47 was expressed to a lesser degree than gras42. The expression levels of gras47 suggest that it is likely a pseudogene and does not contribute to plant growth and development. For these reasons, we propose that the phenotypes observed in gras42-mu1021149 mutant are the result of a loss of gras42 and not a partial loss phenotype due to the loss of one of two functional copies.
RNA-Seq analysis of gras42-mu1021149 mutant reveals tissue-specific changes in gene expression
We analyzed the RNA sequencing data from homozygous gras42-mu1021149 mutants and heterozygous controls (phenotypically normal) to identify differentially expressed genes (DEGs). Each of the three tissues sampled at the V3 developmental stage was analyzed separately. The number of significant DEGs (FDR adjusted P-value < 0.05 and |log2FC| ≥ 1) in gras42-mu1021149 as compared to wild type varied in the three tissues, as expected from the tissue-specific variation in GRAS42 accumulation across these three tissues. In stem and SAM, where GRAS42 was expressed (Fig. 4), we identified 58 DEGs, 32 of which were upregulated in gras42-mu1021149 and 26 were downregulated (Fig. 4). Despite no detectable expression of GRAS42 in the base of L3 (Fig. 4), 85 DEGs were detected, of which 83 were downregulated in gras42-mu1021149, and only two were upregulated (Fig. 4). No DEGs were detected in gras42-mu1021149 in the expanding leaf sheaths despite the expression of GRAS42 in these samples (Fig. 4). The DEGs in stem and L3 base were largely non-overlapping and only two genes were differentially expressed in both tissue samples. The lack of substantial overlap between the DEGs in these tissues indicated that the gene expression consequences of gras42-mu1021149 are likely tissue-specific. This was also evident from the lack of any consistent pattern in the direction of fold change of the DEGs across the other tissues (Fig. 4). Moreover, the majority of the genes that were altered by gras42-mu1021149 in stem and SAM were either not expressed or not impacted in the other two tissues (Fig. 4, Supplementary Table S2).
Among the 58 DEGS in the stem including SAM tissue, there were several notable developmental regulators. Genes with altered expression included rough sheath1 (rs1, Zm00001d018742) and lg3 (Zm00001d040611), both of which are key regulators in leaf angle and development (Becraft and Freeling 1994; Muehlbauer et al. 1999). Both rs1 and lg3 are members of class I KNOX family and encode homeodomain proteins (Bolduc et al. 2014). The expression of both genes was only detected in stem (including SAM) but not in the expanding leaf sheaths and L3 base. Dominant gain-of-function Lg3-O and Rs1-O mutants exhibit blade-to-sheath transformation phenotypes and upright leaf angle. Transcripts from rs1 and lg3 were upregulated in gras42-mu1021149 as compared to wild type suggesting that these genes may be playing a role in the leaf angle phenotype of this mutant. Transcripts encoded by maize dwarf3 (d3, Zm00001d045563), a GA biosynthetic gene, also increased in accumulation in gras42-mu1021149. In addition, gras42-mu1021149 showed a decrease in the accumulation of YUCCA6 (Zm00001d008255) transcripts involved in auxin biosynthesis (Jeong et al. 2007). There was also increased accumulation of transcripts encoded by a paralog of the auxin-responsive and auxin-conjugating enzyme auxin amido synthase6 which is a member of the GretchenHagen3 family of proteins (aas6, Zm00001d043244) (Aoi et al. 2020).
In gras42-mu1021149 L3 base, 85 genes were differentially expressed as compared to wild type. The transcript levels of a maize homolog of the constitutive photomorphogenic dwarf gene of Arabidopsis (Ohnishi et al. 2012) encoding a CYP90A1 involved in BR biosynthesis, cytochrome P450 37-like 1 (cyp37l1, Zm00001d004957) were downregulated in gras42-mu1021149 as compared to wild-type plants. But the gene encoding this enzyme was not affected in stem and expanding leaf sheaths. Genes encoding GA2oxidases (Zm00001d039394, Zm00001d043411), major GA catabolic enzymes, were downregulated in gras42-mu1021149. Transcripts from the maize dwarf & irregular leaf1 (dil1, Zm00001d038087) gene, encoding an AP2 transcription factor, were decreased in abundance in gras42-mu1021149. The dil1 mutant is remarkably similar in appearance to gras42-mu1021149, and is a semi-dwarf with short, wide, crinkled, and more upright leaves (Jiang et al. 2012). The similarity of these two mutants suggests future experiments to determine if they are in the same pathway.
In Arabidopsis, SCL28 promotes cell expansion and endoreplication by activating SIAMESE-RELATED (SMR) cyclin-dependent kinase inhibitors (Nomoto et al. 2022). There are 12 SMR paralogs in maize and none of them were among the DEG in any of our three tissues. However, the pattern of gras42-mu1021149 effects on these genes was non-random in the stem and SAM tissue. All 12 SMR paralogs were decreased in their accumulation in gras42-mu1021149 as compared to wild-type controls in stem and SAM tissue (Supplementary Table S3). This effect was not consistent across tissues with no discernable effect on accumulation of these genes in expanding leaf sheaths (five up and six down) or L3 base (six up and six down) between gras42-mu1021149 and wild-type controls.
None of the expression patterns illuminated by the single-cell transcriptome analysis were evident in the DEG sets. We performed a co-regulatory module analysis from the single-cell transcriptomic data and identified the top 200 co-expressed genes with gras42 (Supplementary Table S4). The genes co-expressed with gras42 were not enriched among the DEGs in any of the three tissues. This suggests that loss of gras42 did not disrupt the developmental patterns and cellular identities responsible for co-expression in the single-celled transcriptomic data. Furthermore, as was observed for the SMR genes above, there was no enrichment of core cell cycle regulators among the transcript changes affected by gras42-mu1021149. This suggests that the colocalization of GRAS42 transcripts with epidermal markers and the G2M phase of the cell cycle neither predicts its targets nor its role in the control of plant development.
Loss of maize gras42 does not coordinately impact the expression of GA and BR biosynthetic and core signaling pathway genes
Previous findings in rice have shown enhanced expression of BR biosynthetic genes in rice dlt mutants (Tong et al. 2009). In our RNA-seq analysis, the CYP37L1 (Zm00001d004957) transcript was identified as a DEG in L3 base RNA (Fig. 5), but no other BR biosynthetic genes were identified as DEG in gras42-mu1021149 even at a more relaxed nominal P-value < 0.05 cutoff in any of the three tissues (Fig. 5 and Supplementary Fig. S5). We extended the analysis to include sterol biosynthetic genes and only identified the sterol methyltransferase1-like1 (smt1l1, Zm00001d013035) gene as differentially expressed in gras42-mu1021149 and only in stem and SAM samples. In addition, no genes with known roles in BR signaling (e.g. maize homologs of BRI1, BZR1, GSK1, BES1) were differentially expressed even at a relaxed cutoff of an uncorrected P-value < 0.05 in comparisons between gras42-mu1021149 and heterozygous controls (Fig. 5 and Supplementary S5). These findings indicate that maize gras42 is not a key regulator of transcription for genes in sterol or BR biosynthesis and signaling.
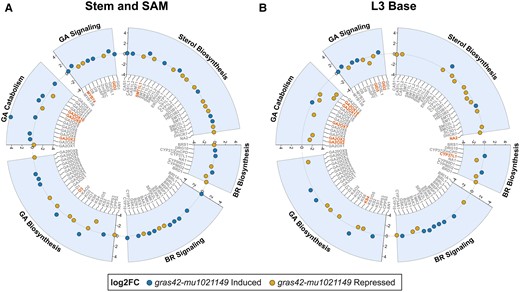
Expression of brassinosteroid (BR) and gibberellin (GA) biosynthetic and core signaling genes in maize gras42-mu1021149 mutant. Circle plots showing log2 fold change of BR and GA pathway genes in gras42-mu1021149 mutant in A) stem and shoot apical meristem (SAM) and B) base of third leaf (L3 base). Blue dots indicate genes induced in gras42-mu1021149 as compared to WT and gold dots indicate genes repressed in gras42-mu1021149. The genes highlighted in red were significant at unadjusted P-value (P ≤ 0.05) from DEG analysis. The genes in gray were not significant in DEG analysis at P ≤ 0.05.
Li et al. (2010) demonstrated that the rice dlt mutant, d62, had reduced endogenous levels of GA1 and increased expression of GA biosynthetic genes. We examined our maize gras42-mu1021149 RNA-seq experiments for changes in transcript levels of the maize orthologs to the GA biosynthetic genes cps1 (ent-copalyl diphosphate synthase 1), ks1 (ent-kaurene synthase 1), ko1 (ent-kaurene oxidase 1), kao1 (ent-kaurenoic acid oxidase 1), and ga2oxidases (Supplementary Table S5). To reduce the false negative rate inherent in transcriptome-wide false discovery rate controls (used above in the DEG analysis) we used an unadjusted P-value below 0.05 to determine the statistical significance of these genes for differential expression. This had very little effect on the detection of differential gene expression, indicating that the GA pathway was not the main target of GRAS42 in maize. As mentioned above, and similarly to rice d62, the kaurene oxidase encoded by d3 (Zm00001d045563) was increased in abundance in the gras42-mu1021149 stem and SAM samples (Fig. 5, Supplementary Table S5). However, there was no significant change in the transcript levels of any of the other GA biosynthetic genes in gras42-mu1021149 relative to wild type in this tissue. Furthermore, gras42-mu1021149 had tissue-specific effects on gene expression and no consistent pattern of gene expression effects on these genes was detected (Fig. 5). The other significant DEG in our data were ga2oxidases, ga2ox3, ga2ox9, and ga2ox10 (Zm00001d043411, Zm00001d008909, and Zm00001d012712, respectively), where loss of gras42-mu1021149 resulted in increased expression (Fig. 5, Supplementary Table S5). No changes in GA biosynthetic genes were noted in expanding leaf sheaths (Supplementary Fig. S5). A number of ga2oxidases (ga2ox2 [Zm00001d002999], ga2ox3, ga2ox7 [Zm00001d038695], ga2ox13 [Zm00001d039394], and ga2ox14 [Zm00001d035994]) were impacted by gras42-mu1021149 in L3 base but the effect was in the opposite direction as observed in the stem and SAM samples demonstrating no consistent pattern for the loss of gras42 on GA biosynthetic gene expression (Fig. 5). The an1 (Zm00001d032961) gene, which encodes the copalyl diphosphate synthase required for GA biosynthesis, was also decreased in abundance in L3 base samples (Fig. 5). While it is possible that gras42 is involved in maintaining GA homeostasis in maize, the lack of correspondence in gene expression effects between tissues suggest that this is not the primary role for this transcription factor.
The expression of GA signaling genes was also examined. Transcript abundance of the two DELLA-domain GRAS transcription factor encoding genes, d8 (Zm00001d033680) and d9 (Zm00001d013465), was not affected by loss of GRAS42 in any tissue. The levels of GIBBERELLIN-INSENSITIVE DWARF PROTEIN HOMOLOG2 (GID2, Zm00001d010308) transcripts, which encode a GA receptor homologous to Arabidopsis GID1A, were upregulated in gras42-mu1021149 relative to the wild type in stem and SAM and L3 base experiments but were unaffected in the expanding leaf sheaths (Fig. 5 and Supplementary Fig. S5). In addition, loss of gras42-mu1021149 led to increased accumulation of transcripts encoded by myb74 (Zm00001d012544) in stem and SAM tissue. The only other GA signaling gene that was differentially expressed in gras42-mu1021149 was gid5 (Zm00001d016973). The accumulation of transcripts from gid5, homolog of Arabidopsis SLEEPY1 (SLY1), was significantly reduced in gras42-mu1021149 L3 base but not affected in the other two tissues (Fig. 5, Supplementary Fig. S5, and Supplementary Table S5). Similar to the GA biosynthetic genes, our data suggest that gras42 does not affect the transcript abundances of core GA signaling genes.
gras42-mu1021149 enhances the expression of genes suppressed by BR
The three mutant alleles of gras42 (gras42-mu1021149, gras42-3, gras42-4) are all short with erect leaves. These phenotypes are similar to, but much weaker than, maize BR-deficient brd1, na1-1, and na2-1 mutant phenotypes (Hartwig et al. 2011; Makarevitch et al. 2012; Best et al. 2016). To explore the possibility that gras42 influences BR-responsive gene expression independent of altering BR biosynthesis or signaling transcript abundance, we looked at the effect of the loss of gras42 on a set of BR-responsive genes. The list of DEGs that responded to treatment with excess BR from leaf and shoot tissues were obtained from previously published work (Trevisan et al. 2020). These consisted of 1,124 genes increased and 377 genes decreased in expression in leaves when treated with BR and 30 genes increased and 192 genes decreased in shoots treated with BR (Trevisan et al. 2020). These genes were analyzed as a set to determine if gras42-mu1021149 had a non-random effect on the direction of expression via chi-squared analyses.
In stem and SAM tissue, loss of GRAS42 in gras42-mu1021149 led to non-random changes in the expression of genes that were previously identified to be differentially expressed following treatment with BR. Overlapping the genes in our RNA-seq analysis with the set of BR-induced genes in maize leaves (Trevisan et al. 2020) identified 1,080 genes in common. Of these, 488 were accumulated and 592 were decreased in abundance consistent with a weak loss of BR response in gras42-mu1021149 SAM tissue (chi-squared P-value = 0.005; Table 1). Overlapping our RNA-seq analysis with the genes suppressed by the treatment of leaves with BR (BR-repressed genes) identified 348 genes also present in our stem and SAM tissue. Of these transcripts, 231 were increased in gras42-mu1021149 and 117 were decreased in accumulation (chi-squared P-value = 2.62E-10). This non-random pattern among the BR-repressed genes was also consistent with a weak loss of BR responsiveness in gras42-mu1021149 SAM tissue. Very few genes were increased in expression in shoot tissue following treatment with BR (Trevisan et al. 2020) and no chi-squared tests were significant for this gene list (Table 2). Among the genes repressed by BR in the sheath and shoot tissue, we identified 149 genes in our RNA-seq experiment with stem and SAM tissues. Of these, a 2:1 ratio (100:49; Table 1) were increased in their accumulation in gras42-mu1021149. This, again, demonstrated a loss of BR-responsive gene expression in gras42-mu1021149 mutants. A similar pattern was observed for leaf and shoot BR-repressed genes in gras42-mu1021149 expanding leaf sheaths (Tables 1 and 2) indicating that gras42-mu1021149 fails to suppress the expression of BR-repressed genes. This indicates a role for gras42 in at least a subset of genes effected by BR treatment.
Distribution of gras42-mu1021149 effects on gene expression for the set of differentially expressed genes in leaf tissue treated with brassinosteroid (BR)
gras42-mu1021149 Tissue . | Gene set: BR effect in leaves . | Observed (gras42-mu1021149 vs WT) . | Expected (gras42-mu1021149 vs WT) . | Chi-Square P-value . | |||
---|---|---|---|---|---|---|---|
Up . | Down . | Total . | Up . | Down . | |||
Stem and SAM | BR induced | 488 | 592 | 1080 | 534.0 | 546.0 | 0.005 |
BR repressed | 231 | 117 | 348 | 172.1 | 175.9 | 2.6E-10 | |
Expanding Leaf Sheaths | BR induced | 522 | 564 | 1086 | 537.1 | 548.9 | 0.36 |
BR repressed | 238 | 110 | 348 | 172.1 | 175.9 | 1.6E-12 | |
L3 Base | BR induced | 398 | 676 | 1074 | 570.8 | 503.1 | 4.2E-26 |
BR repressed | 188 | 171 | 359 | 190.8 | 168.2 | 0.77 |
gras42-mu1021149 Tissue . | Gene set: BR effect in leaves . | Observed (gras42-mu1021149 vs WT) . | Expected (gras42-mu1021149 vs WT) . | Chi-Square P-value . | |||
---|---|---|---|---|---|---|---|
Up . | Down . | Total . | Up . | Down . | |||
Stem and SAM | BR induced | 488 | 592 | 1080 | 534.0 | 546.0 | 0.005 |
BR repressed | 231 | 117 | 348 | 172.1 | 175.9 | 2.6E-10 | |
Expanding Leaf Sheaths | BR induced | 522 | 564 | 1086 | 537.1 | 548.9 | 0.36 |
BR repressed | 238 | 110 | 348 | 172.1 | 175.9 | 1.6E-12 | |
L3 Base | BR induced | 398 | 676 | 1074 | 570.8 | 503.1 | 4.2E-26 |
BR repressed | 188 | 171 | 359 | 190.8 | 168.2 | 0.77 |
Distribution of gras42-mu1021149 effects on gene expression for the set of differentially expressed genes in leaf tissue treated with brassinosteroid (BR)
gras42-mu1021149 Tissue . | Gene set: BR effect in leaves . | Observed (gras42-mu1021149 vs WT) . | Expected (gras42-mu1021149 vs WT) . | Chi-Square P-value . | |||
---|---|---|---|---|---|---|---|
Up . | Down . | Total . | Up . | Down . | |||
Stem and SAM | BR induced | 488 | 592 | 1080 | 534.0 | 546.0 | 0.005 |
BR repressed | 231 | 117 | 348 | 172.1 | 175.9 | 2.6E-10 | |
Expanding Leaf Sheaths | BR induced | 522 | 564 | 1086 | 537.1 | 548.9 | 0.36 |
BR repressed | 238 | 110 | 348 | 172.1 | 175.9 | 1.6E-12 | |
L3 Base | BR induced | 398 | 676 | 1074 | 570.8 | 503.1 | 4.2E-26 |
BR repressed | 188 | 171 | 359 | 190.8 | 168.2 | 0.77 |
gras42-mu1021149 Tissue . | Gene set: BR effect in leaves . | Observed (gras42-mu1021149 vs WT) . | Expected (gras42-mu1021149 vs WT) . | Chi-Square P-value . | |||
---|---|---|---|---|---|---|---|
Up . | Down . | Total . | Up . | Down . | |||
Stem and SAM | BR induced | 488 | 592 | 1080 | 534.0 | 546.0 | 0.005 |
BR repressed | 231 | 117 | 348 | 172.1 | 175.9 | 2.6E-10 | |
Expanding Leaf Sheaths | BR induced | 522 | 564 | 1086 | 537.1 | 548.9 | 0.36 |
BR repressed | 238 | 110 | 348 | 172.1 | 175.9 | 1.6E-12 | |
L3 Base | BR induced | 398 | 676 | 1074 | 570.8 | 503.1 | 4.2E-26 |
BR repressed | 188 | 171 | 359 | 190.8 | 168.2 | 0.77 |
Distribution of gras42-mu1021149 effects on gene expression for the set of differentially expressed genes in stem and leaf sheath tissue treated with BR
gras42-mu1021149 Tissue . | Gene set: BR effect in shoot and leaf sheaths . | Observed (gras42-mu1021149 vs WT) . | Expected (gras42-mu1021149 vs WT) . | Chi-Square P-value . | |||
---|---|---|---|---|---|---|---|
Up . | Down . | Total . | Up . | Down . | |||
Stem and SAM | BR induced | 11 | 10 | 21 | 10.4 | 10.6 | 0.79 |
BR repressed | 100 | 49 | 149 | 73.7 | 75.3 | 1.6E-05 | |
Expanding Leaf Sheaths | BR induced | 9 | 12 | 21 | 10.4 | 10.6 | 0.55 |
BR repressed | 118 | 33 | 151 | 74.7 | 76.3 | 1.7E-12 | |
L3 Base | BR induced | 9 | 11 | 20 | 10.6 | 9.4 | 0.47 |
BR repressed | 41 | 98 | 139 | 73.9 | 65.1 | 2.3E-08 |
gras42-mu1021149 Tissue . | Gene set: BR effect in shoot and leaf sheaths . | Observed (gras42-mu1021149 vs WT) . | Expected (gras42-mu1021149 vs WT) . | Chi-Square P-value . | |||
---|---|---|---|---|---|---|---|
Up . | Down . | Total . | Up . | Down . | |||
Stem and SAM | BR induced | 11 | 10 | 21 | 10.4 | 10.6 | 0.79 |
BR repressed | 100 | 49 | 149 | 73.7 | 75.3 | 1.6E-05 | |
Expanding Leaf Sheaths | BR induced | 9 | 12 | 21 | 10.4 | 10.6 | 0.55 |
BR repressed | 118 | 33 | 151 | 74.7 | 76.3 | 1.7E-12 | |
L3 Base | BR induced | 9 | 11 | 20 | 10.6 | 9.4 | 0.47 |
BR repressed | 41 | 98 | 139 | 73.9 | 65.1 | 2.3E-08 |
Distribution of gras42-mu1021149 effects on gene expression for the set of differentially expressed genes in stem and leaf sheath tissue treated with BR
gras42-mu1021149 Tissue . | Gene set: BR effect in shoot and leaf sheaths . | Observed (gras42-mu1021149 vs WT) . | Expected (gras42-mu1021149 vs WT) . | Chi-Square P-value . | |||
---|---|---|---|---|---|---|---|
Up . | Down . | Total . | Up . | Down . | |||
Stem and SAM | BR induced | 11 | 10 | 21 | 10.4 | 10.6 | 0.79 |
BR repressed | 100 | 49 | 149 | 73.7 | 75.3 | 1.6E-05 | |
Expanding Leaf Sheaths | BR induced | 9 | 12 | 21 | 10.4 | 10.6 | 0.55 |
BR repressed | 118 | 33 | 151 | 74.7 | 76.3 | 1.7E-12 | |
L3 Base | BR induced | 9 | 11 | 20 | 10.6 | 9.4 | 0.47 |
BR repressed | 41 | 98 | 139 | 73.9 | 65.1 | 2.3E-08 |
gras42-mu1021149 Tissue . | Gene set: BR effect in shoot and leaf sheaths . | Observed (gras42-mu1021149 vs WT) . | Expected (gras42-mu1021149 vs WT) . | Chi-Square P-value . | |||
---|---|---|---|---|---|---|---|
Up . | Down . | Total . | Up . | Down . | |||
Stem and SAM | BR induced | 11 | 10 | 21 | 10.4 | 10.6 | 0.79 |
BR repressed | 100 | 49 | 149 | 73.7 | 75.3 | 1.6E-05 | |
Expanding Leaf Sheaths | BR induced | 9 | 12 | 21 | 10.4 | 10.6 | 0.55 |
BR repressed | 118 | 33 | 151 | 74.7 | 76.3 | 1.7E-12 | |
L3 Base | BR induced | 9 | 11 | 20 | 10.6 | 9.4 | 0.47 |
BR repressed | 41 | 98 | 139 | 73.9 | 65.1 | 2.3E-08 |
In L3 base, we identified 1,074 genes overlapping with the leaf BR-induced genes (Trevisan et al. 2020), 63% of which were suppressed by loss of GRAS42 consistent with weak loss of BR responsiveness. However, a divergent pattern was observed for the genes repressed by BR in shoot (Trevisan et al. 2020) and our L3 base RNA-seq experiment (Table 2). In this tissue, gras42-mu1021149 led to an effect on gene expression that resembled a gain of BR signaling. This was manifested as 41 BR-repressed genes increasing and 98 BR-repressed genes decreasing in abundance in the L3 base samples of gras42-mu1021149 relative to wild-type controls. As shown previously, gras42 is not expressed in L3 base (Fig. 4) and is predominantly expressed in dividing cells (Fig. 3). Thus, the consequences on gene expression are likely to be a secondary effect of the loss of gras42 elsewhere in the plant.
GRAS42 is a suppressor of GA-induced gene expression
Though the gras42-mu1021149 mutant did not act through transcription of genes in GA signaling or synthesis, we tested if it was a regulator of GA signal transduction. To examine the impact of loss of gras42 on GA-responsive gene expression, we obtained genes differentially expressed in wild-type maize leaf sheaths (V3 stage) upon GA treatment from a previously published study (Wang et al. 2019). This dataset comprised 254 genes induced, and 81 genes repressed upon GA treatment. Of the 254 GA-induced genes in leaf sheath (Wang et al. 2019), we identified 238 genes present in our RNA sequencing in stem and SAM as well as expanding leaf sheaths and 235 in L3 base (Table 3). GA-induced genes were non-randomly affected by loss of gras42 in stem and SAM consistent with greater GA responses in gras42-mu1021149 mutants. Of the 238 GA-induced genes, 144 transcripts were increased and 94 decreased in abundance gras42-mu1021149 as compared to wild type. On the other hand, in the data from expanding leaf sheaths, where our RNA-seq data are the tissue closest to that used in the previous GA treatment experiment (Wang et al. 2019), transcript levels of 187 GA-induced genes were higher in gras42-mu1021149 and 51 were lower as compared to wild type. The gras42-mu1021149 mutant had no effect on the direction of expression for GA-repressed genes in our stem and SAM RNA-seq data set. Expanding leaf sheath data did show a non-random distribution where 46 of the 74 GA-repressed genes were suppressed in gras42-mu1021149 as compared to wild type. These expression patterns were consistent with an excess GA response in this mutant, suggesting that gras42 encodes a negative regulator of GA signaling in these two tissue types.
Distribution of gras42-mu1021149 effects on gene expression for the set of differentially expressed genes in leaf sheath tissue treated with gibberellin (GA)
gras42-mu1021149 Tissue . | Gene set: GA effect in leaf sheath . | Observed (gras42-mu1021149 vs WT) . | Expected (gras42-mu1021149 vs WT) . | Chi-Square P-value . | |||
---|---|---|---|---|---|---|---|
Up . | Down . | Total . | Up . | Down . | |||
Stem and SAM | GA induced | 144 | 94 | 238 | 117.7 | 120.3 | 0.00064 |
GA repressed | 39 | 34 | 73 | 36.1 | 36.9 | 0.47 | |
Expanding Leaf Sheaths | GA induced | 187 | 51 | 238 | 117.7 | 120.3 | 2.6E-19 |
GA repressed | 28 | 46 | 74 | 36.6 | 37.4 | 0.046 | |
L3 Base | GA induced | 52 | 183 | 235 | 124.9 | 110.1 | 1.5E-21 |
GA repressed | 29 | 45 | 74 | 39.3 | 34.7 | 0.016 |
gras42-mu1021149 Tissue . | Gene set: GA effect in leaf sheath . | Observed (gras42-mu1021149 vs WT) . | Expected (gras42-mu1021149 vs WT) . | Chi-Square P-value . | |||
---|---|---|---|---|---|---|---|
Up . | Down . | Total . | Up . | Down . | |||
Stem and SAM | GA induced | 144 | 94 | 238 | 117.7 | 120.3 | 0.00064 |
GA repressed | 39 | 34 | 73 | 36.1 | 36.9 | 0.47 | |
Expanding Leaf Sheaths | GA induced | 187 | 51 | 238 | 117.7 | 120.3 | 2.6E-19 |
GA repressed | 28 | 46 | 74 | 36.6 | 37.4 | 0.046 | |
L3 Base | GA induced | 52 | 183 | 235 | 124.9 | 110.1 | 1.5E-21 |
GA repressed | 29 | 45 | 74 | 39.3 | 34.7 | 0.016 |
Distribution of gras42-mu1021149 effects on gene expression for the set of differentially expressed genes in leaf sheath tissue treated with gibberellin (GA)
gras42-mu1021149 Tissue . | Gene set: GA effect in leaf sheath . | Observed (gras42-mu1021149 vs WT) . | Expected (gras42-mu1021149 vs WT) . | Chi-Square P-value . | |||
---|---|---|---|---|---|---|---|
Up . | Down . | Total . | Up . | Down . | |||
Stem and SAM | GA induced | 144 | 94 | 238 | 117.7 | 120.3 | 0.00064 |
GA repressed | 39 | 34 | 73 | 36.1 | 36.9 | 0.47 | |
Expanding Leaf Sheaths | GA induced | 187 | 51 | 238 | 117.7 | 120.3 | 2.6E-19 |
GA repressed | 28 | 46 | 74 | 36.6 | 37.4 | 0.046 | |
L3 Base | GA induced | 52 | 183 | 235 | 124.9 | 110.1 | 1.5E-21 |
GA repressed | 29 | 45 | 74 | 39.3 | 34.7 | 0.016 |
gras42-mu1021149 Tissue . | Gene set: GA effect in leaf sheath . | Observed (gras42-mu1021149 vs WT) . | Expected (gras42-mu1021149 vs WT) . | Chi-Square P-value . | |||
---|---|---|---|---|---|---|---|
Up . | Down . | Total . | Up . | Down . | |||
Stem and SAM | GA induced | 144 | 94 | 238 | 117.7 | 120.3 | 0.00064 |
GA repressed | 39 | 34 | 73 | 36.1 | 36.9 | 0.47 | |
Expanding Leaf Sheaths | GA induced | 187 | 51 | 238 | 117.7 | 120.3 | 2.6E-19 |
GA repressed | 28 | 46 | 74 | 36.6 | 37.4 | 0.046 | |
L3 Base | GA induced | 52 | 183 | 235 | 124.9 | 110.1 | 1.5E-21 |
GA repressed | 29 | 45 | 74 | 39.3 | 34.7 | 0.016 |
Interestingly, the gene expression pattern affected by gras42-mu1021149 for GA-induced genes in L3 base was opposite to that observed in the other two tissues. Among the 235 GA-induced genes, transcript abundance of 183 decreased in gras42-mu1021149 indicating that the mutant gene expression pattern in this tissue resembles a loss of GA. This divergent behavior of L3 base from other two tissues is somewhat similar to our previous observations with shoot BR-repressed genes and may reflect the secondary impact of the mutant in this tissue where GRAS42 was not accumulated (Fig. 4).
Gene expression identifies leaf sheath as BR-responsive tissue and gras42 as a suppressor of BR-repressed genes
We created a parametric summary statistic to complement the gene set tests carried out by chi-squared tests (Tables 1, 2, and 3). To create a single value to report the response status of a transcriptional data set for BR or GA signaling, we calculated an average of the Z-scores for all DEGs that responded to each treatment by significantly increasing or decreasing in abundance. This turned each set of DEGs into a single value reporter of that treatment's signaling status in the tissues sampled for gene expression analysis (Fig. 6). These index values aggregate the response of maize genes to excess BRs or GA. Any significant change in these indices in gras42-mu1021149 as compared to wild-type controls will give us an indication of the activation state of the BR or GA response pathways in gras42-mu1021149 mutants.
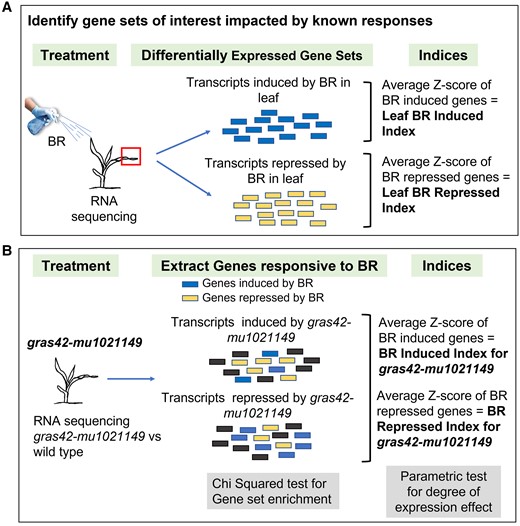
Schematic for Index calculation. A) The first step is to identify the gene sets impacted by known responses. In this example, genes differentially expressed in leaf tissue upon brassinosteroid (BR) treatment formed the two BR-responsive gene sets. Genes with increased transcripts in BR-treated samples were referred to as leaf BR-induced genes and those with decreased transcript counts after BR treatment were referred to as leaf BR-repressed genes. A z-score was calculated for each gene in the two (mock-treated and BR-treated) samples. Average of the z-scores for all the genes enhanced by BR treatment gives a single value (Leaf BR-induced index) that reports positive response to excess BR. Likewise, Leaf BR-repressed index summarizes the decreased expression in response to excess BR. B) Expression patterns of BR-responsive gene sets identified above were analyzed in gras42-mu1021149 to assess the status of BR signaling in gras42-mu1021149. The counts of BR-induced transcripts (and BR-repressed transcripts) enhanced or repressed by gras42-mu1021149 were analyzed by chi-square test. The z-scores of leaf BR-responsive genes were calculated from the normalized counts in WT and gras42-mu1021149. The average of z-scores for each gene set in gras42-mu1021149 resulted in index values that summarize BR response in gras42-mu1021149.
A summary of the positive response to BR excess in the leaf was constructed using the set of transcripts that increased in abundance upon BR treatment of wild-type maize (Trevisan et al. 2020). The Z-scores of the normalized counts for these genes were calculated using all replicates from wild type and gras42-mu1021149 across our RNA-seq experiments (Fig. 6). The value, a leaf “BR-induced index” (Fig. 6), for each genotype was generated by taking the mean Z-score of these genes. If the gene expression pattern in that sample resembles the coordinated increase in BR-induced genes, this index value will increase. If the expression pattern resembles a loss of BR signaling, this value will decrease. This value reports the similarity of any sample's gene expression pattern to BR-induced gene expression. Likewise, an index value summarizing the genes repressed by BR excess, the leaf “BR-repressed index”, was calculated from the average change in expression in our experiments for all transcripts significantly suppressed by BR treatment in the leaf tissue (Trevisan et al. 2020). Similar indices were calculated using BR-responsive genes in shoot tissue (Trevisan et al. 2020).
The comparison of BR indices in gras42-mu1021149 and wild-type siblings allowed us to predict the status of BR responsiveness in the mutant (Fig. 7). Loss of gras42 led a reduction in BR-induced genes (Leaf BR-induced index) an increase in BR-repressed genes (Leaf BR-repressed index). This weak loss of BR signaling in gras42-mu1021149 is similar to that observed by the chi-squared set enrichment analysis (Table 1). The leaf BR-repressed index also increased in gras42-mu1021149 expanding leaf sheaths consistently indicating a weak loss of BR signaling in gras42-mu1021149. In L3 base, neither of the two leaf BR indices showed any significant changes (Fig. 7) consistent with the lack of gras42 gene expression in this tissue (Fig. 4). The BR-induced index calculated from shoot RNA data (Shoot BR-induced index) was not impacted by loss of gras42 in any of the three tissues. Very few genes (n = 21) were induced by BR excess in the shoot experiment, resulting in low power to detect significant changes in gene expression across our experiments. The shoot BR-repressed index comprised 149 genes and therefore provides a better estimate of expression effects. Similar to the leaf BR-repressed index, the Shoot BR-repressed index increased in the gras42-mu1021149 mutant stem and SAM as well as in expanding leaf sheaths (Fig. 7). This once again demonstrated reduced BR responses in the gras42-mu1021149 mutants. All results were coherent with the chi-squared test observations and demonstrate that gras42 is a positive regulator of BR signaling in maize stem and expanding leaf sheaths where gras42 is expressed. The stronger effect on genes repressed by BR may indicate that gras42 acts as a negative regulator of the genes repressed by BR signal transduction.
![Brassinosteroid (BR) indices in wild type (WT) and gras42-mu1021149 across three tissues (stem and shoot apical meristem [SAM], expanding leaf sheaths and base of third leaf [L3 base]). A) Leaf BR-induced index (Solid blue line with circle) comprises 1,104 genes enhanced by BR treatment and Leaf BR-repressed index (solid yellow line with diamond) represents 374 genes repressed by BR treatment; B) Shoot BR-induced index (Solid blue line with circle) comprises 21 genes enhanced by BR treatment and Shoot BR-repressed index (dashed yellow line with diamond) represents 190 genes repressed by BR treatment. A student's t-test was performed to determine the significant differences between the three replicates of WT and gras42-mu1021149 profiled by RNA-Seq. ns, not significant; ***P < 0.001.](https://oup.silverchair-cdn.com/oup/backfile/Content_public/Journal/plphys/195/4/10.1093_plphys_kiae147/1/m_kiae147f7.jpeg?Expires=1750215284&Signature=mAVcI3cVBzhF70tZFj0wQlQ6qArR3RYebNTZPIITrsTK5xxcAcFK7s0KvZwHeML~vxW1bmvTdaikZ8Gi2vh-TxkSJHKGGD~8l0x4BPlSyGrItOenfy2CIKEs-zjgZvQUQRuUHLZOi~uM0PUgnwRbUTelakKKxyOMRMSo-YP6MnVos9Ahzar~Pgl2U3z2BSn4Jdqj7SzRf7urLO--CPr9m98lUIAffNhjN3dMVzZRpQu3-m3TlsUvACDPCGY5g1xhPDxhA4toDJLKUjHfPfkfNYm-FEe9A8SjlN9Hnwe0hyLIkZPMARq0PEsiyPhNNfwMUzgqXiNhBsIo-X9mfowyYg__&Key-Pair-Id=APKAIE5G5CRDK6RD3PGA)
Brassinosteroid (BR) indices in wild type (WT) and gras42-mu1021149 across three tissues (stem and shoot apical meristem [SAM], expanding leaf sheaths and base of third leaf [L3 base]). A) Leaf BR-induced index (Solid blue line with circle) comprises 1,104 genes enhanced by BR treatment and Leaf BR-repressed index (solid yellow line with diamond) represents 374 genes repressed by BR treatment; B) Shoot BR-induced index (Solid blue line with circle) comprises 21 genes enhanced by BR treatment and Shoot BR-repressed index (dashed yellow line with diamond) represents 190 genes repressed by BR treatment. A student's t-test was performed to determine the significant differences between the three replicates of WT and gras42-mu1021149 profiled by RNA-Seq. ns, not significant; ***P < 0.001.
The shoot BR-repressed index showed a significant reduction in gras42-mu1021149 as compared to wild type in L3 base (Fig. 7) suggesting a possible increase in BR signaling. This was similar to what was observed in the chi-squared analysis of the BR-regulated gene sets in the L3 base RNA-seq experiment (Tables 1 and 2). The absence of GRAS42 accumulation in L3 base (Fig. 4) and accumulation in actively dividing cells (Fig. 3) suggests that any effects observed in these experiments are likely indirect effects of defects in mutant growth or mobile signals from other parts of the plant.
Comparison of these indices across the wild-type control tissues can also be used to explore differences in the relative activities of BR responses as a result of maize development. Comparing the BR-induced and BR-repressed indices in wild-type samples revealed tissue-specific effects on the state of BR-responsive genes (Fig. 7). The two Leaf BR indices indicate positive BR signaling in wild-type leaf sheath and stem and SAM samples, but not L3 Base (Fig. 7). A similar expression pattern was observed using the indices calculated from the shoot BR treatments (Fig. 7). These results suggest greater BR response in these tissues either from greater BR levels or more sensitive signaling. The leaf sheath sample had index values consistent with the highest BR signaling levels among these three tissues. Remarkably, in wild-type L3 base samples the expression of BR-repressed genes (Leaf BR-repressed index) was higher than the expression of BR-induced genes (Leaf BR-induced index) (Figure 7). A similar result was evident by the distribution of shoot BR-induced and shoot BR-repressed indices in L3 base (Fig. 7). This suggests repression of BR-induced genes in the fully expanded leaf tissue and may contribute to the opposing patterns seen for these gene sets in L3 base as compared to the two actively growing tissues (Tables 1 and 2 and Fig. 7).
Gene expression identifies leaf sheath as high GA and rules out gras42 as a core GA signaling gene
Index values reporting GA-responsive transcripts were calculated using the DEGs identified following GA excess treatment of maize seedlings (Wang et al. 2019). This permitted comparison of GA pathway activation status across the three wild-type maize tissues. Gene expression in the wild-type stem and SAM sample exhibited lower expression of GA-induced genes and higher expression of GA-repressed genes than the other two samples, indicating low GA response in this tissue. The rapidly elongating tissue of the expanding leaf sheaths, by contrast, showed the highest GA signaling of the three samples (Fig. 8).
![Gibberellin (GA) indices in wild type (WT) and gras42-mu1021149 across three tissues (stem and shoot apical meristem [SAM], expanding leaf sheaths and base of third leaf [L3 base]). GA-induced index (Solid blue line with circle) comprises 242 genes enhanced by GA treatment and GA-repressed index (dashed yellow line with diamond) represents 75 genes repressed by GA treatment. A student's t-test was performed to determine the significant (P-value <0.05) differences between the three replicates of WT and gras42-mu1021149 profiled by RNA-Seq. ns, not significant; ***P < 0.001.](https://oup.silverchair-cdn.com/oup/backfile/Content_public/Journal/plphys/195/4/10.1093_plphys_kiae147/1/m_kiae147f8.jpeg?Expires=1750215284&Signature=NJUEqH~bY7FQdY5N~doV~Aea0-CfsKeKsx9zCScjuBsuboIlZBvHvrfMAZpZhyRMq11TIHHWGE9MUkLD-k3f3Xp8E-DNu9JndZ~NiXWqWhq7yTohwMCZsX5BAYd1CNQsml9J4F5cvkmum-1z8EX6IW4GZnPVJEjaW4vOaKIKr67snUmBp964tt1opBaQJsqPlMCi90EtRSA9a5dyWWrRMjNNrFr4Tr0dad6Ir60oGvt9g2J8f8i60lZZukp48rkGB3Sp9Du~XObGX-VEWNLN8KQgm44-ASQLcqSoOdgExrIxhEuMIC4QSYPdvZZDY4GzIffhSsZCnoNpFXAytxt8dg__&Key-Pair-Id=APKAIE5G5CRDK6RD3PGA)
Gibberellin (GA) indices in wild type (WT) and gras42-mu1021149 across three tissues (stem and shoot apical meristem [SAM], expanding leaf sheaths and base of third leaf [L3 base]). GA-induced index (Solid blue line with circle) comprises 242 genes enhanced by GA treatment and GA-repressed index (dashed yellow line with diamond) represents 75 genes repressed by GA treatment. A student's t-test was performed to determine the significant (P-value <0.05) differences between the three replicates of WT and gras42-mu1021149 profiled by RNA-Seq. ns, not significant; ***P < 0.001.
Loss of gras42 increased the GA-induced index value in expanding leaf sheaths. This suggests excess GA signaling in this mutant in this tissue. In the L3 base samples, however, the GA-induced index was reduced in gras42-mu1021149 indicating a reduced GA signaling by this mutant in this tissue. Given the very low expression of gras42 in our leaf samples and preferential accumulation in actively dividing cells (Fig. 4 and Fig. 3), this may be an indirect effect of the mutant. No effect was observed for either index in the stem and SAM RNA-seq experiments. Taken together with the chi-squared analysis, the inconsistency of the response across tissues and weak effects suggests that gras42 is not a core regulator of GA signaling in maize.
Loss of gras42 influences the phenotype of GA and BR biosynthetic mutants
We carried out a genetic test of whether the observed differences in hormone-regulated gene expression, were part of the mechanism of altered growth and development in the gras42-mu1021149 mutant by generating double mutants of gras42-mu1021149 with na1-1 and d1. The na1-1 mutant is a classical maize dwarf carrying a mutation in the homolog of Arabidopsis DE-ETIOLATED2 (DET2), encoding sterol 5-alpha reductase, a key enzyme in BR biosynthesis (Hartwig et al. 2011). The na1-1 mutants have short, dark green leaves, and often exhibit the presence of pistils in the tassel florets. The d1 mutant a GA-deficient dwarf due to loss of ent-kaurene synthase activity, an early step in GA biosynthesis (Fu et al. 2016). The d1 dwarfs display an increase in the number of tillers and retention of anthers in the pistillate flowers of the ear. Both na1-1 and d1 single mutants are severely reduced in height as compared to the wild type. Previous work demonstrated context-dependent genetic interactions between BR and GA deficiency (Best et al. 2016). GA and BR were additive for plant height, the increased branching of GA mutants required BR, and the tassel phenotypes in BR-deficient mutants required GA (Best et al. 2016). Based on the expression analysis, we expect gras42-mu1021149 to behave like a weak loss of BR mutant. If this is correct, gras42-mu1021149 should recapitulate the interactions described previously by enhancing the retention of pistils in the na1-1 mutant and reducing the number of tillers in the d1 mutant. If it is a GA signaling mutant, on the other hand, we should observe an increase in tillers in d1 and a suppression of pistil retention in na1-1 tassels.
Double-mutant phenotypes between gras42-mu1021149 and na1-1 or d1 were consistent with gras42-mu1021149 affecting a weak loss of BR signaling. As compared to all single mutants, plant height was reduced even further in the gras42-mu1021149/na1-1 and gras42-mu1021149/d1 double mutants indicating an additive effect of these mutations on height (Fig. 9). Previous findings have shown that BR biosynthetic mutants could suppress the tillering induced by GA mutants (Best et al. 2016). Like other BR mutants, gras42-mu1021149 completely suppressed the tillering induced by d1 in gras42-mu1021149/d1 double mutants (Fig. 9). Thus, just like BR-deficient dwarfs (Best et al. 2016) gras42 is required for loss of GA to induce tillering. Although the gras42-mu1021149 tassels did not exhibit any silks, loss of GRAS42 in the gras42-mu1021149/na1-1 double mutants enhanced the penetrance of the persistence of pistils in the tassel florets (Fig. 10). No normal tassels were observed in gras42-mu1021149/na1-1 double mutants. Ninety-two percent of the gras42-mu1021149/na1-1 tassels had silks, and the remainder were barren (Fig. 10). Some gras42-mu1021149 single mutant tassels exhibited barren branches (Fig. 10) as was observed in GA excess treatments (Best and Dilkes 2022). All gras42-mu1021149/d1 tassels were completely normal demonstrating that d1 suppresses the gras42-mu1021149 barren phenotype and that, just as was the case for the presence of pistils in the tassel for BR-deficient mutants, GA is required for the gras42-mu1021149 tassel phenotype. It has been previously shown that GA can induce male sterility in maize (Nelson and Rossman 1958; Best and Dilkes 2022). The suppression of the barren phenotype by gras42-mu1021149/d1 double mutants indicates that the male sterility in gras42-mu1021149 may be due to excess GA in this tissue. In summary, gras42-mu1021149 enhanced the height impacts of both na1-1 and d1 mutants, enhanced the retention of pistils in the tassel florets of na1-1, suppressed the increased branching of GA deficiency, and exhibited a barren tassel phenotype that required GA. Thus, gras42-mu1021149 and its interactions closely resemble a loss of BR signaling.
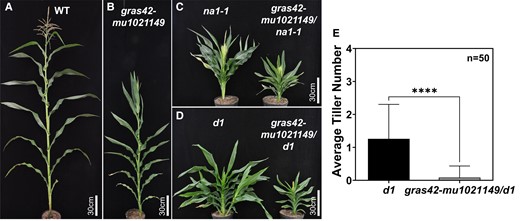
Phenotype of single and double mutants. A) Plant height of mature wild type (gras42-mu1021149/+) gras42-mu1021149 homozygote; B) Plant height of na1-1 and gras42-mu1021149/na1-1 double mutant; C) Plant height and tillers of d1 and gras42-mu1021149/d1 double mutant; D) Average number of tillers in d1 and gras42-mu1021149/d1 double mutant. Error bars are ± SD. Asterisks represents a significant difference between d1 and gras42-mu1021149/d1 determined by student's t-test. (**** = P < 0.0001).
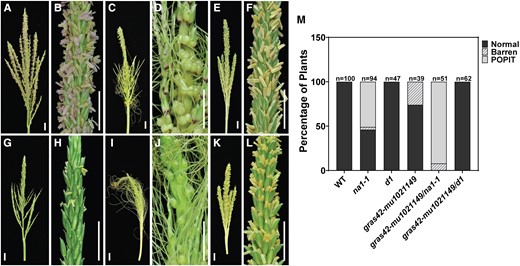
Tassel phenotype in single and double mutants. A–B) WT (gras42-mu1021149/+); C–D)na1-1; E–F)d1; G–H)gras42-mu1021149; I–J)gras42-mu1021149/na1-1; K–L)gras42-mu1021149/d1. Scale bars = 2 cm; M) Percentage of plants showing normal, barren tassels and presence of pistil in tassel (POPIT) phenotypes in WT and mutants (n = number of plants).
Expression level polymorphism discovery via genome-wide association for the GRAS42 transcript
To identify natural variants regulating the expression of the gras42 gene, we performed an expression level genome-wide association study (eGWAS) using published data from RNA-seq of seedling shoots from 296 inbred lines (Kremling et al. 2018). This identified 153 SNPs with a P-value <1E-5 for accumulation of the GRAS42 transcript (Fig. 11). Among these SNPs, 19 were located within 1 Mb of the gras42 gene and likely identified cis-acting regulatory variation (Fig. 11, Supplementary Table S6).
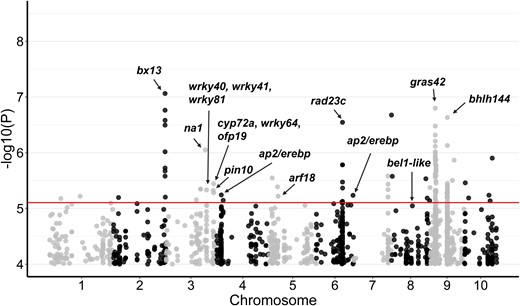
Manhattan plot for eGWAS of gras42 expression in 296 maize diverse lines. The dots represent SNPs associated with gras42 gene expression. Red line is the P-value (P) threshold selected to identify significant associations. The black arrows highlight selected potential candidate genes of interest.
In addition to the cis-eQTLs, 134 alleles had trans effects on GRAS42 accumulation (Fig. 11, Table 4, Supplementary Table S6). Among these, eight SNPs on chromosome 2 were associated with benzoxazinone synthesis13 (bx13, Zm00001d007718). One of the trans-acting SNPs was located in a region containing the previously discussed BR biosynthetic gene na1. Two SNPs were located in a locus with three potential candidate genes: cyp72a/cyp11 (Zm00001d044159), wrky64 (Zm00001d044162), and ovate family protein19 (ofp19, Zm00001d044167). The cyp72a gene is a homolog of Arabidopsis CYP72C1 that likely controls BR homeostasis (Thornton et al. 2010). Gene ofp19 is a homolog of rice OsOFP2. Ovate family proteins are transcription repressors know to interact with DLT in rice and are involved in modulating BR responses through this interaction (Xiao et al. 2017; Yang et al. 2018). The wrky64 gene encodes a homolog of AtWRKY71 that controls shoot branching in Arabidopsis by regulating the auxin pathway. Another locus on chromosome 8 was linked to bel1 like homeodomain protein 9 (Zm00001d010060) which is orthologous to the REPLUMLESS (aka PENNYWISE/BELLRINGER/VAAMANA/LARSON) gene in Arabidopsis which is known to control meristem maintenance, internode patterning, and flowering through interaction with KNOX genes (Kanrar et al. 2006). In addition to wrky64 on chromosome 3, we identified three SNPs adjacent to a cluster of WRKY transcription factors (Zm00001d043060, Zm00001d043062, Zm00001d043063, and Zm00001d043066). Among these, Zm00001d043060 is an ortholog of AtWRKY70 and AtWRKY54, both of which are positive regulators of the BR pathway in Arabidopsis (Chen et al. 2017). We also identified SNPs linked to pin-formed protein10 (pin10), a putative auxin efflux carrier encoded by Zm00001d044083, and auxin response factor18 (arf18, Zm00001d014377) as potential candidates affecting GRAS42 accumulation in our eGWAS. In addition, ubiquitin receptor rad23c (Zm00001d037326), E3 ubiquitin-protein ligase xbat33 (Zm00001d045499) and E3 ubiquitin ligase upl1 (Zm00001d023795) were identified as potential candidate genes affecting the accumulation of GRAS42. Overlap of trans-acting eGWAS loci with the gras42 co-expressed genes identified from the single-cell expression analysis (Supplementary Table S4) was worse than expected by random chance, indicating that co-expression did not enrich for regulators of gras42 expression. Together this suggests that BR, auxin, and protein turnover pathways may all influence the accumulation of GRAS42 in maize.
Candidate genes for selected trans regulators of GRAS42 accumulation from genome-wide analysis (GWA) of expression level in germinating shoots
SNP_ID . | CHR . | POS . | SNP Effect . | P-value . | Candidate genes . | Annotation . |
---|---|---|---|---|---|---|
2-238126834 | 2 | 238126834 | 0.256559263 | 2.63E-06 | Zm00001d007718 | benzoxazinone synthesis13 (bx13) |
2-238126871 | 2 | 238126871 | 0.269199382 | 3.19E-07 | ||
2-238126877 | 2 | 238126877 | 0.249651008 | 1.88E-06 | ||
2-238126885 | 2 | 238126885 | 0.261970019 | 9.63E-07 | ||
2-238126887 | 2 | 238126887 | 0.252683244 | 2.13E-06 | ||
2-238126898 | 2 | 238126898 | 0.27493553 | 1.73E-07 | ||
2-238126917 | 2 | 238126917 | 0.27870554 | 2.62E-07 | ||
2-238126921 | 2 | 238126921 | 0.287780931 | 8.64E-08 | ||
3-181905371 | 3 | 181905371 | 0.231101747 | 8.92E-07 | Zm00001d042843 | nana plant1 |
3-187852439 | 3 | 187852439 | 0.305093516 | 4.66E-06 | Zm00001d043060 | wrky81, wrky40, wrky41 |
3-187852502 | 3 | 187852502 | 0.299513231 | 8.84E-06 | ||
3-218581094 | 3 | 218581094 | −0.434818966 | 4.74E-06 | Zm00001d044083 | pin-formed protein10 |
3-220839119 | 3 | 220839119 | 0.308542162 | 3.60E-06 | Zm00001d044159, Zm00001d044162, Zm00001d044167 | cyp72a, wrky64, ovate family protein 19 |
3-220839454 | 3 | 220839454 | 0.279605606 | 5.22E-06 | ||
4-25786156 | 4 | 25786156 | 0.332474966 | 5.72E-06 | Zm00001d049309 | AP2/EREBP transcription factor family protein |
4-25793512 | 4 | 25793512 | 0.328920312 | 9.77E-06 | ||
5-43614831 | 5 | 43614831 | 0.304801044 | 6.04E-06 | Zm00001d014377 | auxin response factor 18 |
6-121088509 | 6 | 121088509 | 0.256423203 | 7.45E-06 | Zm00001d037326 | ubiquitin receptor rad23c |
6-121105526 | 6 | 121105526 | 0.308627916 | 2.85E-07 | ||
6-121109485 | 6 | 121109485 | 0.269018031 | 3.36E-06 | ||
6-169631183 | 6 | 169631183 | −0.216194592 | 5.81E-06 | Zm00001d039077 | AP2/EREBP transcription factor family protein |
8-97557162 | 8 | 97557162 | 0.460036521 | 8.99E-06 | Zm00001d010060 | bel1-like homeodomain protein 9 |
9-24602648 | 9 | 24602648 | 0.253305497 | 9.62E-07 | Zm00001d045499 | E3 ubiquitin-protein ligase XBAT33 |
9-24602809 | 9 | 24602809 | −0.219449999 | 2.74E-06 | ||
9-24602814 | 9 | 24602814 | −0.214284656 | 4.73E-06 | ||
9-80799902 | 9 | 80799902 | 0.214318727 | 4.63E-06 | Zm00001d046332 | Transcription factor bhlh144 |
9-80799911 | 9 | 80799911 | 0.215975058 | 3.90E-06 | ||
9-80800029 | 9 | 80800029 | 0.20923684 | 8.17E-06 | ||
9-80804330 | 9 | 80804330 | 0.212343734 | 5.65E-06 | ||
9-80845657 | 9 | 80845657 | 0.209586406 | 7.60E-06 | ||
9-80856131 | 9 | 80856131 | 0.230804504 | 7.42E-07 | ||
9-80872311 | 9 | 80872311 | 0.213091242 | 5.22E-06 | ||
9-80872445 | 9 | 80872445 | 0.206834285 | 1.00E-05 | ||
9-80876041 | 9 | 80876041 | 0.212724836 | 6.18E-06 | ||
9-80882352 | 9 | 80882352 | 0.210570565 | 8.17E-06 | ||
9-80887549 | 9 | 80887549 | 0.240565074 | 2.33E-07 | ||
9-80887566 | 9 | 80887566 | 0.207684343 | 9.17E-06 | ||
9-80903148 | 9 | 80903148 | 0.213727359 | 4.90E-06 | ||
9-80903157 | 9 | 80903157 | 0.215941274 | 3.87E-06 | ||
9-80903168 | 9 | 80903168 | 0.215941274 | 3.87E-06 | ||
10-21259279 | 10 | 21259279 | 0.224851277 | 6.49E-06 | Zm00001d023795 | E3 ubiquitin-protein ligase UPL1 |
SNP_ID . | CHR . | POS . | SNP Effect . | P-value . | Candidate genes . | Annotation . |
---|---|---|---|---|---|---|
2-238126834 | 2 | 238126834 | 0.256559263 | 2.63E-06 | Zm00001d007718 | benzoxazinone synthesis13 (bx13) |
2-238126871 | 2 | 238126871 | 0.269199382 | 3.19E-07 | ||
2-238126877 | 2 | 238126877 | 0.249651008 | 1.88E-06 | ||
2-238126885 | 2 | 238126885 | 0.261970019 | 9.63E-07 | ||
2-238126887 | 2 | 238126887 | 0.252683244 | 2.13E-06 | ||
2-238126898 | 2 | 238126898 | 0.27493553 | 1.73E-07 | ||
2-238126917 | 2 | 238126917 | 0.27870554 | 2.62E-07 | ||
2-238126921 | 2 | 238126921 | 0.287780931 | 8.64E-08 | ||
3-181905371 | 3 | 181905371 | 0.231101747 | 8.92E-07 | Zm00001d042843 | nana plant1 |
3-187852439 | 3 | 187852439 | 0.305093516 | 4.66E-06 | Zm00001d043060 | wrky81, wrky40, wrky41 |
3-187852502 | 3 | 187852502 | 0.299513231 | 8.84E-06 | ||
3-218581094 | 3 | 218581094 | −0.434818966 | 4.74E-06 | Zm00001d044083 | pin-formed protein10 |
3-220839119 | 3 | 220839119 | 0.308542162 | 3.60E-06 | Zm00001d044159, Zm00001d044162, Zm00001d044167 | cyp72a, wrky64, ovate family protein 19 |
3-220839454 | 3 | 220839454 | 0.279605606 | 5.22E-06 | ||
4-25786156 | 4 | 25786156 | 0.332474966 | 5.72E-06 | Zm00001d049309 | AP2/EREBP transcription factor family protein |
4-25793512 | 4 | 25793512 | 0.328920312 | 9.77E-06 | ||
5-43614831 | 5 | 43614831 | 0.304801044 | 6.04E-06 | Zm00001d014377 | auxin response factor 18 |
6-121088509 | 6 | 121088509 | 0.256423203 | 7.45E-06 | Zm00001d037326 | ubiquitin receptor rad23c |
6-121105526 | 6 | 121105526 | 0.308627916 | 2.85E-07 | ||
6-121109485 | 6 | 121109485 | 0.269018031 | 3.36E-06 | ||
6-169631183 | 6 | 169631183 | −0.216194592 | 5.81E-06 | Zm00001d039077 | AP2/EREBP transcription factor family protein |
8-97557162 | 8 | 97557162 | 0.460036521 | 8.99E-06 | Zm00001d010060 | bel1-like homeodomain protein 9 |
9-24602648 | 9 | 24602648 | 0.253305497 | 9.62E-07 | Zm00001d045499 | E3 ubiquitin-protein ligase XBAT33 |
9-24602809 | 9 | 24602809 | −0.219449999 | 2.74E-06 | ||
9-24602814 | 9 | 24602814 | −0.214284656 | 4.73E-06 | ||
9-80799902 | 9 | 80799902 | 0.214318727 | 4.63E-06 | Zm00001d046332 | Transcription factor bhlh144 |
9-80799911 | 9 | 80799911 | 0.215975058 | 3.90E-06 | ||
9-80800029 | 9 | 80800029 | 0.20923684 | 8.17E-06 | ||
9-80804330 | 9 | 80804330 | 0.212343734 | 5.65E-06 | ||
9-80845657 | 9 | 80845657 | 0.209586406 | 7.60E-06 | ||
9-80856131 | 9 | 80856131 | 0.230804504 | 7.42E-07 | ||
9-80872311 | 9 | 80872311 | 0.213091242 | 5.22E-06 | ||
9-80872445 | 9 | 80872445 | 0.206834285 | 1.00E-05 | ||
9-80876041 | 9 | 80876041 | 0.212724836 | 6.18E-06 | ||
9-80882352 | 9 | 80882352 | 0.210570565 | 8.17E-06 | ||
9-80887549 | 9 | 80887549 | 0.240565074 | 2.33E-07 | ||
9-80887566 | 9 | 80887566 | 0.207684343 | 9.17E-06 | ||
9-80903148 | 9 | 80903148 | 0.213727359 | 4.90E-06 | ||
9-80903157 | 9 | 80903157 | 0.215941274 | 3.87E-06 | ||
9-80903168 | 9 | 80903168 | 0.215941274 | 3.87E-06 | ||
10-21259279 | 10 | 21259279 | 0.224851277 | 6.49E-06 | Zm00001d023795 | E3 ubiquitin-protein ligase UPL1 |
Candidate genes for selected trans regulators of GRAS42 accumulation from genome-wide analysis (GWA) of expression level in germinating shoots
SNP_ID . | CHR . | POS . | SNP Effect . | P-value . | Candidate genes . | Annotation . |
---|---|---|---|---|---|---|
2-238126834 | 2 | 238126834 | 0.256559263 | 2.63E-06 | Zm00001d007718 | benzoxazinone synthesis13 (bx13) |
2-238126871 | 2 | 238126871 | 0.269199382 | 3.19E-07 | ||
2-238126877 | 2 | 238126877 | 0.249651008 | 1.88E-06 | ||
2-238126885 | 2 | 238126885 | 0.261970019 | 9.63E-07 | ||
2-238126887 | 2 | 238126887 | 0.252683244 | 2.13E-06 | ||
2-238126898 | 2 | 238126898 | 0.27493553 | 1.73E-07 | ||
2-238126917 | 2 | 238126917 | 0.27870554 | 2.62E-07 | ||
2-238126921 | 2 | 238126921 | 0.287780931 | 8.64E-08 | ||
3-181905371 | 3 | 181905371 | 0.231101747 | 8.92E-07 | Zm00001d042843 | nana plant1 |
3-187852439 | 3 | 187852439 | 0.305093516 | 4.66E-06 | Zm00001d043060 | wrky81, wrky40, wrky41 |
3-187852502 | 3 | 187852502 | 0.299513231 | 8.84E-06 | ||
3-218581094 | 3 | 218581094 | −0.434818966 | 4.74E-06 | Zm00001d044083 | pin-formed protein10 |
3-220839119 | 3 | 220839119 | 0.308542162 | 3.60E-06 | Zm00001d044159, Zm00001d044162, Zm00001d044167 | cyp72a, wrky64, ovate family protein 19 |
3-220839454 | 3 | 220839454 | 0.279605606 | 5.22E-06 | ||
4-25786156 | 4 | 25786156 | 0.332474966 | 5.72E-06 | Zm00001d049309 | AP2/EREBP transcription factor family protein |
4-25793512 | 4 | 25793512 | 0.328920312 | 9.77E-06 | ||
5-43614831 | 5 | 43614831 | 0.304801044 | 6.04E-06 | Zm00001d014377 | auxin response factor 18 |
6-121088509 | 6 | 121088509 | 0.256423203 | 7.45E-06 | Zm00001d037326 | ubiquitin receptor rad23c |
6-121105526 | 6 | 121105526 | 0.308627916 | 2.85E-07 | ||
6-121109485 | 6 | 121109485 | 0.269018031 | 3.36E-06 | ||
6-169631183 | 6 | 169631183 | −0.216194592 | 5.81E-06 | Zm00001d039077 | AP2/EREBP transcription factor family protein |
8-97557162 | 8 | 97557162 | 0.460036521 | 8.99E-06 | Zm00001d010060 | bel1-like homeodomain protein 9 |
9-24602648 | 9 | 24602648 | 0.253305497 | 9.62E-07 | Zm00001d045499 | E3 ubiquitin-protein ligase XBAT33 |
9-24602809 | 9 | 24602809 | −0.219449999 | 2.74E-06 | ||
9-24602814 | 9 | 24602814 | −0.214284656 | 4.73E-06 | ||
9-80799902 | 9 | 80799902 | 0.214318727 | 4.63E-06 | Zm00001d046332 | Transcription factor bhlh144 |
9-80799911 | 9 | 80799911 | 0.215975058 | 3.90E-06 | ||
9-80800029 | 9 | 80800029 | 0.20923684 | 8.17E-06 | ||
9-80804330 | 9 | 80804330 | 0.212343734 | 5.65E-06 | ||
9-80845657 | 9 | 80845657 | 0.209586406 | 7.60E-06 | ||
9-80856131 | 9 | 80856131 | 0.230804504 | 7.42E-07 | ||
9-80872311 | 9 | 80872311 | 0.213091242 | 5.22E-06 | ||
9-80872445 | 9 | 80872445 | 0.206834285 | 1.00E-05 | ||
9-80876041 | 9 | 80876041 | 0.212724836 | 6.18E-06 | ||
9-80882352 | 9 | 80882352 | 0.210570565 | 8.17E-06 | ||
9-80887549 | 9 | 80887549 | 0.240565074 | 2.33E-07 | ||
9-80887566 | 9 | 80887566 | 0.207684343 | 9.17E-06 | ||
9-80903148 | 9 | 80903148 | 0.213727359 | 4.90E-06 | ||
9-80903157 | 9 | 80903157 | 0.215941274 | 3.87E-06 | ||
9-80903168 | 9 | 80903168 | 0.215941274 | 3.87E-06 | ||
10-21259279 | 10 | 21259279 | 0.224851277 | 6.49E-06 | Zm00001d023795 | E3 ubiquitin-protein ligase UPL1 |
SNP_ID . | CHR . | POS . | SNP Effect . | P-value . | Candidate genes . | Annotation . |
---|---|---|---|---|---|---|
2-238126834 | 2 | 238126834 | 0.256559263 | 2.63E-06 | Zm00001d007718 | benzoxazinone synthesis13 (bx13) |
2-238126871 | 2 | 238126871 | 0.269199382 | 3.19E-07 | ||
2-238126877 | 2 | 238126877 | 0.249651008 | 1.88E-06 | ||
2-238126885 | 2 | 238126885 | 0.261970019 | 9.63E-07 | ||
2-238126887 | 2 | 238126887 | 0.252683244 | 2.13E-06 | ||
2-238126898 | 2 | 238126898 | 0.27493553 | 1.73E-07 | ||
2-238126917 | 2 | 238126917 | 0.27870554 | 2.62E-07 | ||
2-238126921 | 2 | 238126921 | 0.287780931 | 8.64E-08 | ||
3-181905371 | 3 | 181905371 | 0.231101747 | 8.92E-07 | Zm00001d042843 | nana plant1 |
3-187852439 | 3 | 187852439 | 0.305093516 | 4.66E-06 | Zm00001d043060 | wrky81, wrky40, wrky41 |
3-187852502 | 3 | 187852502 | 0.299513231 | 8.84E-06 | ||
3-218581094 | 3 | 218581094 | −0.434818966 | 4.74E-06 | Zm00001d044083 | pin-formed protein10 |
3-220839119 | 3 | 220839119 | 0.308542162 | 3.60E-06 | Zm00001d044159, Zm00001d044162, Zm00001d044167 | cyp72a, wrky64, ovate family protein 19 |
3-220839454 | 3 | 220839454 | 0.279605606 | 5.22E-06 | ||
4-25786156 | 4 | 25786156 | 0.332474966 | 5.72E-06 | Zm00001d049309 | AP2/EREBP transcription factor family protein |
4-25793512 | 4 | 25793512 | 0.328920312 | 9.77E-06 | ||
5-43614831 | 5 | 43614831 | 0.304801044 | 6.04E-06 | Zm00001d014377 | auxin response factor 18 |
6-121088509 | 6 | 121088509 | 0.256423203 | 7.45E-06 | Zm00001d037326 | ubiquitin receptor rad23c |
6-121105526 | 6 | 121105526 | 0.308627916 | 2.85E-07 | ||
6-121109485 | 6 | 121109485 | 0.269018031 | 3.36E-06 | ||
6-169631183 | 6 | 169631183 | −0.216194592 | 5.81E-06 | Zm00001d039077 | AP2/EREBP transcription factor family protein |
8-97557162 | 8 | 97557162 | 0.460036521 | 8.99E-06 | Zm00001d010060 | bel1-like homeodomain protein 9 |
9-24602648 | 9 | 24602648 | 0.253305497 | 9.62E-07 | Zm00001d045499 | E3 ubiquitin-protein ligase XBAT33 |
9-24602809 | 9 | 24602809 | −0.219449999 | 2.74E-06 | ||
9-24602814 | 9 | 24602814 | −0.214284656 | 4.73E-06 | ||
9-80799902 | 9 | 80799902 | 0.214318727 | 4.63E-06 | Zm00001d046332 | Transcription factor bhlh144 |
9-80799911 | 9 | 80799911 | 0.215975058 | 3.90E-06 | ||
9-80800029 | 9 | 80800029 | 0.20923684 | 8.17E-06 | ||
9-80804330 | 9 | 80804330 | 0.212343734 | 5.65E-06 | ||
9-80845657 | 9 | 80845657 | 0.209586406 | 7.60E-06 | ||
9-80856131 | 9 | 80856131 | 0.230804504 | 7.42E-07 | ||
9-80872311 | 9 | 80872311 | 0.213091242 | 5.22E-06 | ||
9-80872445 | 9 | 80872445 | 0.206834285 | 1.00E-05 | ||
9-80876041 | 9 | 80876041 | 0.212724836 | 6.18E-06 | ||
9-80882352 | 9 | 80882352 | 0.210570565 | 8.17E-06 | ||
9-80887549 | 9 | 80887549 | 0.240565074 | 2.33E-07 | ||
9-80887566 | 9 | 80887566 | 0.207684343 | 9.17E-06 | ||
9-80903148 | 9 | 80903148 | 0.213727359 | 4.90E-06 | ||
9-80903157 | 9 | 80903157 | 0.215941274 | 3.87E-06 | ||
9-80903168 | 9 | 80903168 | 0.215941274 | 3.87E-06 | ||
10-21259279 | 10 | 21259279 | 0.224851277 | 6.49E-06 | Zm00001d023795 | E3 ubiquitin-protein ligase UPL1 |
Discussion
In this study we report a semi-dwarf maize mutant, gras42-mu1021149. We identified three mutant alleles of gras42 in the maize ortholog of the rice DLT and Arabidopsis SCL28 genes. A mutator transposon insertion allele, gras42-mu1021149, was characterized in depth. The mutant has short dark green leaves, upright leaf angles, reduced tillering, and partially barren tassel branches (Fig. 1 and Fig. 10). The phenotype of loss of gras42 is consistent with that reported for dlt loss-of-function mutants in rice (Tong et al. 2009; Li et al. 2010) and resembles some of the known BR-deficient mutants of maize (Hartwig et al. 2011; Best et al. 2016). The Mu insertion in gras42-mu1021149 resulted in the accumulation of truncated transcripts corresponding to the first third of the gene up to the Mu insertion site. Reads downstream from the Mu insertion were also detected, though at much lower levels, and their accumulation was consistent with the ectopic promoter activity of Mu responding to the regulatory environment of gras42 (Supplementary Fig. S2). Prior study found a similar pattern in other Mu insertions (Ellison et al. 2023). A systematic analysis of aberrant transcripts from Mu insertions would determine whether this observation is an unusual anecdote or an aspect of Mu's biology.
Differential gene expression analysis by RNA-seq suggests that gras42 could determine leaf angle via regulation of KNOX transcription factors and auxin levels. We identified transcripts of two class I KNOX family genes, lg3 and rs1, that increased in the gras42-mu1021149 RNA-seq data from stem and SAM tissue. The likely auxin biosynthetic gene, yucca6, was also downregulated in gras42-mu1021149. In addition, an early auxin-responsive gene, aas6, was differentially expressed in gras42-mu1021149. AAS6 is a putative auxin amido synthase gene in the GH3 family which is also known to regulate leaf angle in rice (Zhao et al. 2013; Zhang et al. 2015). The accumulation of GRAS42 in cells undergoing the G2/M transition in shoot apices (Fig. 3) suggests that these regulatory events may occur during or concomitant with the modulation of cell division patterns early in organ development.
Maize GRAS42 regulates BR response
The semi-dwarf stature of the gras42-mu1021149 mutant resembled the expected phenotype from weak BR deficiency. An analysis of gene expression was consistent with a loss of BR signaling in gras42-mu1021149. Expression changes in known BR-responsive genes were used as indicators of BR pathway activation in gras42-mu1021149. Gene expression changes at BR-responsive genes in gras42-mu1021149 resembled a loss of BR response in the mutant. The expression pattern in both of our actively growing tissues: stem and SAM and expanding leaf sheaths were consistent with a weak loss of BR response in gras42-mu1021149 (Tables 1 and 2). This was also evident using a parametric index calculated from the expression effects on BR-responsive genes. Loss of gras42-mu1021149 increased the transcript levels of BR-repressed genes and decreased the transcripts of BR-induced genes (Fig. 7). The examination of gene expression consequences of loss of gras42-mu1021149 indicates that GRAS42 acts as a positive regulator of BR-responsive gene expression. During the drafting of this manuscript, Wang et al. (2022) showed that ZmBZR1 (aka BZR1, GRMZM5G812774, Zm00001d046305, Zm0001eb384820) and ZmBEH1 (aka BES1, GRMZM2G102514, Zm00001d02197, Zm00001eb325550) bind to the promoter of gras42 (Wang et al. 2022). Working with the same gras42-mu1021149 allele, Wang et al. (2022) showed that these maize mutants are semi-dwarf and have more upright leaves than wild-type siblings. In rice, the orthologous gene, DLT, interacts with OSH15 to regulate the expression of the BR receptor, BRI1, and BR catabolizing enzyme CYP734A5/6 (Niu et al. 2022). These results demonstrate that GRAS42 plays roles both upstream and downstream of BR signaling. This is consistent with our finding that GRAS acts as positive regulator of BR-responsive gene expression.
Our double-mutant interactions were also consistent with gras42 encoding a positive regulator of BR-responsive gene expression. The enhanced penetrance of the presence of pistils in the tassel florets of gras42-mu1021149/na1-1 double mutants is consistent with weak loss of BR signaling in gras42-mu1021149 (Fig. 10). The gras42 gene was required for BRs to suppress pistils in tassel florets suggesting a role of gras42 in BR signaling. Furthermore, gras42-mu1021149 suppressed tillering in gras42-mu1021149/d1 double mutants indicating that gras42 is required for loss of GA to induce tillering (Fig. 9). This is consistent with the suppression of tillering observed due to loss of BR in double mutants defective in GA and BR biosynthesis (Best et al. 2016). In addition, the previously observed requirement for GA production for pistil production in tassel florets of BR-deficient plants (Best et al. 2016, 2017), the GA-deficient mutant d1 suppressed the tassel phenotype of gras42-mu1021149 (Fig. 10). Taken together, these findings show that gras42 positively affects BR signaling which is consistent with previous reports with the role of DLT in rice (Tong et al. 2009, 2012; Yang et al. 2018). However, unlike studies in rice, we did not observe consistent alterations in the expression of BR biosynthetic or core signaling genes in maize gras42-mu1021149 mutants (Fig. 5 and Supplementary Fig. S5). The gras42-mu1021149 mutants exhibit or enhance every phenotype associated with a loss BR biosynthesis including plant height, retention of pistils in tassel florets, and suppression of tiller outgrowth in GA loss-of-function mutants (Fig. 1, Fig. 9, and Fig. 10). However, this effect is context dependent and tissues in which GRAS42 is not accumulated (e.g. mature leaf tissue, Fig. 4) were not impacted for BR-regulated gene expression while rapidly expanding tissues were (Table 1 and 2 and Fig. 7).
Does GRAS42 regulate the GA pathway?
Two opposing roles for DLT in the regulation of the GA pathway were previously proposed from work in rice. In one study, the levels of GA biosynthetic genes were increased in rice dlt mutants (Li et al. 2010). In a second study, the accumulation of transcripts encoding a GA catabolic gene was increased and transcripts encoding two GA biosynthetic genes were unaffected in rice dlt mutants (Tong et al. 2014). We did not observe consistent changes in transcript levels of GA biosynthetic genes in our three RNA-seq experiments (Fig. 5 and Supplementary Fig. S5). We also did not see consistent changes in the levels of GA-regulated gene expression in our three RNA-seq experiments (Table 3 and Fig. 8). The only GA biosynthetic gene differentially expressed in any RNA-seq comparison between gras42-mu1021149 and wild-type siblings was d3, and that was only differentially expressed in the stem and SAM tissue (Fig. 5). In addition, the expression levels of core GA signaling genes were not consistently impacted in gras42-mu1021149 (Fig. 5 and Supplementary Fig. S5). This demonstrates that GRAS42 is not a primary regulator of GA biosynthesis and signaling in maize. We did observe an increase in the transcript levels of GA catabolic enzymes (GA2OX) in gras42-mu1021149 stem and SAM tissue (Fig. 5), similar to the Tong et al. (2014) study, but this effect was not consistent across tissues. The finding of coordinated accumulation of GA-regulated genes by gras42-mu1021149 in our RNA-seq experiments, but with opposite effects depending on the tissue, is similar to the context-dependent epistatic interactions between BR and GA biosynthetic mutants in maize (Best et al. 2016) that was confirmed using hormone biosynthetic inhibitors (Best et al. 2017).
We used hormone-regulated gene sets to examine the state of signaling across our different tissues and genotypes. These composite values, indices, derived from the expression of a set of genes report on the status of hormone response. Gene expression indices calculated using the set of genes affected by BR excess demonstrate active BR signaling in actively dividing stem and SAM as well as expanding leaf sheaths of wild-type plants (Fig. 7). However, a reversal in this pattern was observed in already expanded L3 base suggesting that expanded leaves have suppressed or no BR signaling. These finding are consistent with the tissue-specific distribution of BR biosynthesis reported previously (Shimada et al. 2003). Our data reveals that this tissue specificity also extends to BR responses. Tissue dependency was also detected in GA responses using indices calculated in the three tissues using the set of genes affected by GA excess. In contrast to high BR response in stem including SAM, a low GA response was detected in this tissue in the wild-type plants. While the expanding leaf sheath showed active GA signaling, no response was detected in L3 base. Since the GA and BR responses are variable across tissues, it further suggests that mechanisms of interaction between the two hormones are tissue-specific and gras42 may influence this through interaction with other transcription factors.
The responses to loss of gras42 were also tissue-specific. Different sets of genes were differentially expressed in stem and L3 base. In addition, expression analysis of specific gene sets showed opposite responses in the actively growing and already expanded tissues. For instance, gras42-mu1021149 led to an increase in transcript levels of ga2oxidases in stem and SAM but a decrease in ga2oxidases was observed in L3 base (Fig. 5). This flipping of gene expression consequences between tissues was also evident from the transcript levels of BR- and GA-responsive genes. The distribution of the two sets of BR-repressed genes (leaf and shoot) in gras42-mu1021149 L3 base indicated a gain of BR response in this tissue which is the opposite of what we observe in the other two tissues (Tables 2 and 3). Similarly, the pattern of GA-induced genes in L3 base contrasts to that in the other two tissues indicating a tissue-specific role of gras42. These differences could be attributed to GRAS42 expression levels in the three tissues (Fig. 4) and/or tissue-specific protein interactors. Given the absence of GRAS42 transcripts in L3 base, it is likely that effects in this tissue are secondary effects mediated by variation in mobile signals produced in other parts of the plant.
GRAS42 is unlikely to be the mediator of previously reported tissue-specific epistatic interactions between hormone biosynthetic mutants
As both the wild type and gras42-mu1021149 showed a reversal in BR-responsive gene expression across tissues this suggests that gras42 is not the mediator of the reversed effects between stem, leaf sheaths, and leaf blades (Fig. 7). This opposite effect of gene regulation in a tissue-specific manner is a function of the genes influenced by BR, rather than the effect of gras42-mu1021149 on BR-regulated gene expression. This suggests the existence of a repressor or activator that drives a reversal in the transcriptional control of BR-regulated genes as maize leaf tissue moves from a rapidly expanding and dividing developmental state to maturity. This unknown factor may also be responsible for the reversions in the direction epistatic interactions between BR and GA biosynthetic mutants (Best et al. 2016). The large number of BZR paralogs in maize (Manoli et al. 2018), which may have undergone expression sub functionalization and diverged in protein function, are candidates for the mediators of the differential effects of BR on gene expression between maize tissues.
In this study, we demonstrated that maize gras42 affects both BR- and GA-responsive gene expression in a tissue-specific manner. However, many of these changes are unlikely to be direct. Analysis of previously published single-cell transcriptomic data found GRAS42 transcripts substantially enriched among dividing cells in the shoot apex (Fig. 3). Together with the hormone responsive gene expression analysis, this suggests that gras42 encodes a repressor of BR signaling that acts during cell proliferation. The role for BR in cell cycle regulated gene expression and cell division has been known for decades (Hu et al. 2000; Nakaya et al. 2002; Oakenfull et al. 2002). Previous analyses of BR consequences on cell division have been mixed, but promotion of cell division and cell cycle regulated gene expression has been demonstrated when BR-deficient cells are supplied with exogenous BR (Hu et al. 2000). Though BR excess can also inhibit cell divisions and growth, particularly in root tissues where sub-nanomolar application enhances root growth and greater concentrations inhibit growth. BR has also been implicated in the cell divisions that precede stomatal development in Arabidopsis (Gudesblat et al. 2012; Kim et al. 2012; Wang et al. 2015). The relationship between BR and stomatal development is unclear and complicated by tissue and species-specific effects (Qi and Torii 2018). Though co-expression was detected between GRAS42 and TMM1 and ZmSPCH2 in our analysis of public single-cell data (Supplementary Fig. S1), no differences in stomatal densities or index were observed in the BR-deficient mutant na2 (Best et al. 2016) suggesting that this hormone is not required for normal stomatal development in maize.
The rice ortholog of gras42, DLT has previously been reported to be co-regulated with BZR1 by GSK2 in rice, placing it downstream of GSK2 in BR signaling (Tong et al. 2012). BZR1 has also been shown to bind to the gras42 promoter in maize and rice (Tong et al. 2009; Wang et al. 2022). Our data show that gras42 does not regulate expression of BR biosynthetic genes but does affect the expression of BR-responsive genes (Fig. 5 and Fig. 7). In addition, the expression of brassinozole resistant1 (bzr1, Zm00001d046305) and other upstream BR signaling genes like Arabidopsis BAK1 homolog somatic embryogenesis receptor-like kinase2 -like1 (serk2l1, Zm00001d024430) and brassinosteroid insensitive1-associated receptor kinase like3 (bak3, Zm00001d037010) were not affected by loss of gras42 (Fig. 5 and Supplementary Fig. S5). These results are consistent with gras42 acting downstream of BZR1 in regulating the transcriptional response to BR. On the other hand, DLT has been shown to modulate the expression of GA biosynthetic genes in rice (Li et al. 2010), but its role in GA signaling has not been defined. The expression of GA biosynthetic genes was not affected in maize gras42-mu1021149, but expression of GA-induced genes was affected by loss of gras42. Considering that GA-induced gene expression changes are DELLA dependent, it may be that GRAS42 interacts with DELLAs to modulate GA signaling. We propose that gras42 might be responsible for mediating crosstalk between the BR and GA pathway by interacting with BZR1 and DELLA transcription factors.
Natural variation in GRAS42 transcript accumulation places it downstream of BR
The eGWAS analysis revealed that the expression of gras42 was associated with SNPs linked to known components of the BR pathway, including BR biosynthesis (na1), degradation (cyp72A), and signaling (WRKY transcription factor Zm00001d043060). The ofp19 gene was also identified as a putative candidate regulating the accumulation of GRAS42. OFPs regulate plant growth and development through their interactions with multiple transcription factors. Interactions between OFPs and DLT have been previously reported in rice (Xiao et al. 2017; Yang et al. 2018). OFPs are also known to interact with KNOX and BEL1-like homeodomain (BELL) proteins and suppress the BELL-KNOX heterodimers (Pagnussat et al. 2007; Liu and Douglas 2015; Zhang et al. 2016). A bell9 (Zm00001d010060) gene was identified as a potential regulator of GRAS42 expression in our eGWAS analysis. OFPs have also been shown to function in hormone signaling. OsOFP8 is phosphorylated by GSK2 and plays and role in BR signaling (Yang et al. 2016). The OFPs are also known to affect leaf angle. Gain-of-function mutant Osofp8 and OsOFP8 overexpression lines showed increased leaf angle, whereas the leaf angle was more upright in OsOFP8 RNAi transgenic lines. The finding that ofp19 regulates GRAS42 expression raises the possibility that OFPs function through interaction with GRAS42 in determining leaf inclination.
Materials and methods
Plant material
The maize (Zea mays) Robertson's Mutator (Mu) allele of gras42, gras42-mu1021149 (aka url1-1, scl28-1, bcr1-1, and UFMu-09491) (McCarty et al. 2005; Hartwig 2011; Wang et al. 2022) was identified in the Uniform-Mu population (McCarty et al. 2005). An additional allele of gras42 (gras42-3, url1-2, bcr1-2) was identified from a spontaneous mutant in the CML247 inbred line which failed to complement gras42-1 (Hartwig 2011). An EMS allele, gras42-4, was identified within a family used by the maize TILLInG project (Till et al. 2004; Weil and Monde 2007) which was segregating for a semi-dwarf that also failed to complement gras42-mu1021149. The dwarf1 (d1) allele was obtained from Carolina biological supply. The nana plant1-1 (na1-1) mutant was described previously (Hartwig et al. 2011). To generate the double mutants, pollen from homozygous na1-1 and homozygous d1 mutants was used in crosses to gras42-mu1021149 homozygotes. The resulting F1 plants were selfed and subsequent F2 families were grown and phenotyped for tillering, plant height, and persistence of pistils in the tassels.
Growth conditions
Greenhouse experiments measuring the growth and development of single and double mutants were conducted at the Purdue Horticulture Plant Growth Facility using a 16:8 hr day:night cycle provided by supplemental lighting with temperature settings of 27°C (day) and 21°C (night). Plants were grown in 100% Turface MVP (Profile Products, Inc.) and fertilized with 200 ppm Nitrogen mixed from a 21-5-20 N-P-K mix (Miracle-Gro Excel, Scotts, Marysville, OH) and adjusted to pH 6.
Morphological measurements
Plant height of 20 gras42-mu1021149 heterozygote and 20 homozygote siblings were measured every 7 d starting at 26 d after planting and continued until maturity. Height was measured as the distance from the soil surface to the topmost visible developed leaf collar.
Leaf numbers for gras42-mu1021149 heterozygote and homozygote siblings were counted at maturity. Since the lower leaves undergo senescence before plants reach maturity, every second leaf was marked counting the first developing true leaf as one. Leaf length and width were measured after leaves were fully extended. The distance from the leaf collar to the tip along the midrib was measured for the leaf length, whereas the widest point of the leaf was selected for leaf width measurements.
The leaf angles of gras42-mu1021149 mutants and heterozygous siblings were measured with a digital protractor (WR400 3-inch goniometer, Wixey, Sanibel, FL). The angle between the stem and the abaxial side of the midrib was determined after plants reached maturity (post-anthesis). The inclination angle, the acute angle between the leaf and the stem above the leaf, is reported.
Genotyping
The molecular identity of the gras42-4 allele was determined using Illumina sequencing of the overlapping PCR amplicons covering the entire gras42 locus (Supplementary Table S7). This approach, known as wideseq, has been previously described in Khangura et al. 2019. Briefly, PCR products from a single mutant individual spanning the entire gene were pooled and prepared for sequencing using the Tn5-tagmentation procedure with the Illumina Nextera DNA library preparation kit. These libraries were sequenced using a MiSeq instrument (Illumina, San Diego, CA) at the Purdue Genomics Core Facility. Paired-end reads from each sample were processed to remove adapters and poor quality bases (<Phred-20) using Trimmomatic version 0.22 (Bolger et al. 2014) with the following settings “LEADING:20 TRAILING:20 MINLEN:30”. Only the filtered paired reads were used for further processing. The filtered paired-end reads were aligned to the gras42 genomic sequence using short read aligner BWA version 0.7.12 (Li and Durbin 2009). The alignments were generated using the BWA sampe command with default parameters to generate Sequence Alignment/Map (SAM) files. The SAM files were converted into Binary Alignment Map (BAM) files, followed by sorting, indexing, and finally, variant calling using mpileup function in SAMtools suite version 1.3 (Li et al. 2009). The variants were also manually inspected by visualizing each sample in IGV version 2.8.2 (Thorvaldsdottir et al. 2013) using the sorted BAM files.
The single-nucleotide substitution (G221A) in gras42-4 was followed by PCR and cleavage of amplicons with a restriction endonuclease. This mutation created an AvrII restriction site 5′-C^CTAGG-3′, from 5′-CCTGGG-3′ creating a co-dominant Cleaved Amplified Polymorphic Sequence (CAPS) (Konieczny and Ausubel 1993). Primer pair URL-F2 and URL-R2 (Supplementary Table S7) produced a 797 bp amplicon encompassing the AvrII restriction site. The PCR products were digested with AvrII restriction endonuclease followed by electrophoresis on a 2.5% (w/v) agarose gel could distinguish the wild-type allele as an undigested amplicon of 797 bp and gras42-4 allele as two bands at 642 and 155 bp.
The genotyping of individuals segregating for the gras42-mu1021149 allele was done using gras42-specific primer pairs URL-F2 and URL-R2, along with a Mutator TIR-specific primer Eomu3 (Supplementary Table S7) to create two dominant markers. Individuals with the gras42-mu1021149 allele produced a PCR amplicon when tested with Eomu3 and URL-F2 or URL-R2 primer pairs. The wild-type allele was successfully amplified by the URL-F2 and URL-R2 primer pair.
RNA sequencing and transcriptome analysis
Three tissue types including stem, leaf sheathes from the expanding leaves, and base of the third collared leaf (L3 base) were collected from heterozygous and homozygous gras42-mu1021149 seedlings at V3 developmental stage. The one-inch stem sections comprised the stem, shoot apical meristem (SAM), and the surrounding leaf sheaths. Heterozygous and homozygous plants collected as three replicates of 12 plants each. Total RNA was extracted using TRIzol reagent. The concentration and quality of the RNA was measured using a Nanodrop 2000c spectrophotometer (Thermo Scientific, Waltham, MA). Library construction and sequencing were performed by Novogene using the NovaSeq 6000 sequencing platform (Illumina, San Diego, CA).
The reads were aligned to the maize reference genome (Zm-B73-REFERENCE-GRAMENE-4.0) using hisat2 (Kim et al. 2019). The aligned reads for each sample were individually assembled using cufflinks (Trapnell et al. 2012). Transcript assemblies generated by cufflinks were merged by cuffmerge. The count files containing number of aligned reads corresponding to each gene were generated using htseq-count (Anders et al. 2015). DEGs were called using DEseq2 version 1.26.0 (Love et al. 2014). DEseq2 results for the three tissues are available in Supplementary Table S8. Transcripts with |log2 fold change| ≥ 1 and FDR adjusted P-value ≤ 0.05 were considered as DEGs (Supplementary Table S2).
Analysis of published single-cell RNA-seq
A public dataset from Satterlee et al. (2020) comprising two B73 replicates were obtained from NCBI (SRR11943512 and SRR11943513). Data were processed in Cell Ranger v6.0 (10 × Genomics) making a custom reference genome and mapping to B73 maize genome version 5. A raw gene counts matrix was used as an input to detect empty droplets using DropletUtils v1.16 (Lun et al. 2019). After filtering out empty droplets, the gene counts matrix was imported to the Seurat v4 package, following Seurat standard pipeline (Hao et al. 2021). High quality cells were considered to have less than 5% of mitochondrial expressed genes, more than 2,500 genes detected per cell, and less than 100,000 transcripts detected per cell. Data were transformed using the SCTransform function, which was followed by dimensionality reduction and cell clustering. Different cell clusters were identified with previously published marker genes (Satterlee et al. 2020). Cell cycle scoring was performed to identify clusters undergoing cell division process. Data generated with Seurat were exported using the package SeuratWrappers to an appropriate format for Monocle. Using Monocle v4, we identified modules with co-regulated genes through a graph-autocorrelation analysis (Cao et al. 2019).
Phylogenetic analysis
The protein sequences for GRAS42 homologs in amborella (Amborella trichopoda), asparagus (Asparagus officinalis), garlic (Allium sativum), Arabidopsis (Arabidopsis thaliana), brachypodium (Brachypodium distachyon), rice (Oryza sativa ssp. japonica), setaria (Setaria italica and Setaria viridis), and sorghum (Sorghum bicolor) were obtained from the Plaza monocot 5.0 databases (Van Bel et al. 2022). The sequences were aligned using clustalW (Larkin et al. 2007) with default parameters (Gap opening penalty: 10 and gap extension cost: 0.20). A maximum-likelihood tree was estimated using IQ-TREE version 1.5.5 (Nguyen et al. 2015) with substitution model JTT + I + G4 as the best predicted model by Bayesian information criteria (Kalyaanamoorthy et al. 2017). A consensus tree was generated using ultrafast bootstrapping with 1000 replicates (Minh et al. 2013). The tree was visualized using iTOL v6 (Letunic and Bork 2021).
Gene set enrichment/chi-square test
Publicly available data were utilized to obtain BR- and GA-affected gene sets. The genes differentially expressed in leaf and shoot tissues of maize seedlings treated with 1 nM epibrassinolide were obtained from Trevisan et al. 2020. The DEG sets were divided into two subsets of upregulated and downregulated genes for each tissue. These were named BR-induced and BR-repressed genes, respectively. The DEGs in leaf sheaths of V3 maize seedlings treated with 10−4 M GA3 were obtained from a previous study (Wang et al. 2019) and grouped into GA-induced and GA-repressed gene sets. The normalized counts of genes in a set were extracted from our wild type and gras42-mu1021149 RNA sequencing data for all the three tissues (stem and SAM, expanding leaf sheaths, and L3 base). The effect of gras42-mu1021149 on the expression of a gene set was analyzed using a chi-squared test. The expected proportion of up and downregulated genes was calculated based on the distribution of induced and repressed genes among all expressed genes, not just the DEG sets, in each experiment.
Index calculation
A Z-score was calculated for each gene from the normalized counts in three replicates each of wild type and gras42-mu1021149. The Z-score of each gene for each sample was calculated as follows:
An index was then generated for each sample by taking the average of the Z-scores for the set of BR- and GA-regulated genes described above.
eGWAS
The normalized counts of GRAS42 transcripts in germinating shoot tissue from 296 maize diversity lines were obtained from RNA sequencing performed in a previous study (Kremling et al. 2018). The SNP data for these lines were obtained from maize Hapmap3.2.1 (Bukowski et al. 2018). The SNP data were filtered for minor allele frequency (MAF) ≤ 0.05, and 23.9 M SNPs were retained. For genome-wide association, we modified the R package switchgrassGWAS (Lovell et al. 2021) to adapt the bigsnpr R package (Privé et al. 2018) for maize. The results were filtered to retain SNPs below a P-value of 1E-5 as significantly associated with gras42 expression. The SNPs within 1 Mb of the gras42 translation start site or the gras42 stop codon were considered cis-acting and the rest of the SNPs were considered trans-acting. Within the candidate locus, three genes upstream and three genes downstream from the SNP position were considered as potential candidates associated with the SNP. GWAS results were viewed using an interactive browser: Zbrowse (Ziegler et al. 2015). The annotations for the candidate genes were obtained from several sources including Gramene, MaizeGDB (https://www.maizegdb.org; Portwood et al. 2019; Woodhouse et al. 2021a) and JGI genome portal (https://genome.jgi.doe.gov/portal/).
Accession numbers
The accession numbers for major genes/proteins from this article can be found in Supplementary Table S3, Table S7, and Table S9.
Acknowledgments
The authors would like to acknowledge the assistance of the staff and leadership at the Agronomy Center for Research and Education, Rosen Center for Advanced Computing, and the Horticulture Greenhouse at Purdue University. We are grateful to Dilkes lab members for comments and discussions. We would particularly like to thank everyone who puts extra effort into scientific software documentation and data dissemination that allows research such as this to determine meaning from open-source repositories. In particular, the NSF Cyverse project, Alice MacQueen, and Tom Juenger, and the labs of Ed Buckler, Yao Chen, and Serena Varotto—you are the real heroes.
Author contributions
A.K., N.B.B., T.H., R.S.K., B.S., and B.P.D. designed the research. A.K., N.B.B., T.H., J.B., R.S.K., S.M., A.A.-R., J.S., B.S., and B.P.D. performed the experiments and analyzed data. A.K., N.B.B., T.H., R.S.K., A.A.-R., and B.P.D. wrote the paper. All authors edited and approved the manuscript.
Supplementary data
The following materials are available in the online version of this article.
Supplementary Figure S1. Density plots of maize genes.
Supplementary Figure S2. BAM coverage and alignment of reads that span gras42 gene in stem and SAM tissue.
Supplementary Figure S3. BAM coverage and alignment of reads that span gras42 gene in stem and SAM tissue in expanding leaf sheaths.
Supplementary Figure S4. Phylogenetic tree for GRAS42 protein homologs
Supplementary Figure S5. Circle plot showing log2 fold change of sterol and brassinosteroid (BR) biosynthetic genes, BR signaling genes, and gibberellin (GA) biosynthetic and signaling genes.
Supplementary Table S1. Expression (FPKM) of gras42 and gras47 across different maize tissues obtained from qTeller.
Supplementary Table S2. Differentially expressed genes (DEGs) in gras42-mu1021149 stem and SAM and L3 base tissues.
Supplementary Table S3. Normalized counts of maize smr genes in WT and gras42-mu1021149 in three tissues: stem and SAM, expanding leaf sheaths and L3 base.
Supplementary Table S4. Genes co-expressed with gras42 in single-cell transcriptomic data along with their log2 fold change in gras42-mu1021149 vs WT.
Supplementary Table S5. Expression changes for genes encoding steps in brassinosteroid (BR) and gibberellin (GA) pathways in gras42-mu1021149 mutant.
Supplementary Table S6. Cis and trans variants affecting GRAS42 transcript accumulation in germinating shoot at P < 1E-5.
Supplementary Table S7. Primer pairs used for genotyping of gras42 alleles and wide seq.
Supplementary Table S8. DEseq2 output for gras42-mu1021149 vs WT comparison in three tissues.
Supplementary Table S9. Gene IDs for maize, Arabidopsis and rice genes mentioned in the manuscript.
Funding
This work was supported by United States Department of Agriculture National Institute of Food and Agriculture postdoctoral grant 2022-67012-36601 to R.S.K. and United States Department of Energy Office of Science (BER) Grant DE-SC0023305 and National Science Foundation IOS-1755401 to B.P.D. J.S. is supported by NCSU startup funds.
Data availability
Raw reads from RNA sequencing of gras42-mu1021149 homozygote and heterozygote siblings in three tissues generated and analyzed in this study are available at NCBI Sequence Read Archive (SRA) under the bioproject accession number PRJNA831826.
Dive Curated Terms
The following phenotypic, genotypic, and functional terms are of significance to the work described in this paper:
gibberellin insensitive dwarf protein2 Gramene: Zm00001d010308
gibberellin insensitive dwarf protein2 Araport: Zm00001d010308
gibberellin insensitive dwarf protein5 Gramene: Zm00001d016973
gibberellin insensitive dwarf protein5 Araport: Zm00001d016973
References
Ellison EL, Zhou P, Hermanson P, Chu YH, Read A, Hirsch CN, Grotewold E, Springer NM.
Author notes
The author responsible for distribution of materials integral to the findings presented in this article in accordance with the policy described in the Instructions for Authors (https://dbpia.nl.go.kr/plphys/pages/General-Instructions) is Brian Dilkes.
Conflict of interest statement. None declared.