-
PDF
- Split View
-
Views
-
Cite
Cite
Yunxiao Zhao, Yicun Chen, Ming Gao, Yangdong Wang, Alcohol dehydrogenases regulated by a MYB44 transcription factor underlie Lauraceae citral biosynthesis, Plant Physiology, Volume 194, Issue 3, March 2024, Pages 1674–1691, https://doi.org/10.1093/plphys/kiad553
- Share Icon Share
Abstract
Lineage-specific terpenoids have arisen throughout the evolution of land plants and are believed to play a role in interactions between plants and the environment. Species-specific gene clusters in plants have provided insight on the evolution of secondary metabolism. Lauraceae is an ecologically important plant family whose members are also of considerable economic value given their monoterpene contents. However, the gene cluster responsible for the biosynthesis of monoterpenes remains yet to be elucidated. Here, a Lauraceae-specific citral biosynthetic gene cluster (CGC) was identified and investigated using a multifaceted approach that combined phylogenetic, collinearity, and biochemical analyses. The CGC comprises MYB44 as a regulator and 2 alcohol dehydrogenases (ADHs) as modifying enzymes, which derived from species-specific tandem and proximal duplication events. Activity and substrate divergence of the ADHs has resulted in the fruit of mountain pepper (Litsea cubeba), a core Lauraceae species, consisting of more than 80% citral. In addition, MYB44 negatively regulates citral biosynthesis by directly binding to the promoters of the ADH-encoding genes. The aggregation of citral biosynthetic pathways suggests that they may form the basis of important characteristics that enhance adaptability. The findings of this study provide insights into the evolution of and the regulatory mechanisms involved in plant terpene biosynthesis.
Introduction
Plants can synthesize more than 1 million natural small-molecule compounds with a wide diversity of structures and functions, making them excellent “chemists” (Pichersky and Raguso 2018; Fang et al. 2019; Zhan et al. 2022). Terpenoids, which are among the most abundant and widespread major classes of natural products, are synthesized by all living organisms (Chen et al. 2011; Tholl and Lee 2011; Pichersky and Raguso 2018; Zhan et al. 2022). Almost all plants produce several hundred terpenoids, such as gibberellin, brassinosteroids, carotenoids, and chlorophyll, that are necessary for their growth and development, and are classified as primary metabolites (Pichersky and Raguso 2018). However, the vast majority of terpenoids are restricted to a specific ecological niche or even a single species; consequently, they are defined as specialized terpenoid metabolites (Pichersky and Raguso 2018; Karunanithi et al. 2020; Zhou and Pichersky 2020). Lineage-specific terpenoids such as citral, linalool, and myrcene, which show enrichment during the evolution of land plants, are thought to have a role in ecological interactions, both biotic and abiotic, between the respective plants and their environments.
The biosynthesis of terpenoids has been extensively characterized in several plant lineages, and associations between terpenoids and specific plant lineages have been established (Chen et al. 2011; Dong et al. 2016; Reichardt et al. 2020; He et al. 2022). Studies have shown that specialized terpenoids are typically produced together with key enzymes in the terpenoid synthesis pathway, such as GGPPS and terpene synthase (TPS), which have evolved independently in different species (Chen et al. 2011; Dong et al. 2016; Reichardt et al. 2020; He et al. 2022). The appearance of new genes that encode enzymes capable of producing novel metabolites has facilitated the evolution of specialized terpenoid structures in plant lineages (Pichersky and Raguso 2018; Jia et al. 2022). In some cases, the genes involved in terpene biosynthesis have been shown to form clusters (Chen et al. 2020a; Reichardt et al. 2020; Zhan et al. 2022). Plant metabolic gene clusters are defined as groups of 2 or more nonhomologous but closely linked genes that encode biosynthetic enzymes, transporters, and cofactor synthases (Liu et al. 2020a; Zhan et al. 2022).
Structural genes involved in terpene precursor biosynthetic pathways include those encoding rate-limiting factors within the cytosolic mevalonate (MVA) and plastidic 2-C-methyl-D-erythritol 4-phosphate (MEP) pathways (McGarvey and Croteau 1995) and the trans/cis-prenyltransferases that convert isopentenyl diphosphate (IPP) and dimethylallyl diphosphate to polyprenyl diphosphates (Zhou and Pichersky 2020). The TPS gene family (Chen et al. 2011; Celedon and Bohlmann 2019; Booth et al. 2020; Chen et al. 2020b; Reichardt et al. 2020; Zhou and Pichersky 2020) and modifying enzymes such as cytochrome P450 oxygenases, dehydrogenases, methyltransferases, acyltransferases, and glycosyltransferases (Strommer 2011; Liu et al. 2016; Celedon and Bohlmann 2019) are also important constituents of the terpene biosynthetic pathway, and have been functionally characterized in tomato (Solanum lycopersicum), Arabidopsis (Arabidopsis thaliana), and sweet wormwood (Artemisia annua) (Liu et al. 2016; Liu et al. 2020a, 2020b; Zhan et al. 2022).
In addition to structural genes, transcription factors (TFs) are also an intrinsic component of secondary metabolism regulation. R2R3-MYBs comprise a large plant gene family involved in the regulation of several metabolic pathways, including those relating to terpenes, flavones, anthocyanins, and carotenoids (Dubos et al. 2010; Li and Zachgo 2013; Wang et al. 2019; Wei et al. 2022). While some R2R3-MYBs act specifically on a single gene of the terpene pathway, others have a broader impact on multiple genes and branches within this pathway (Karppinen et al. 2021). Furthermore, several R2R3-MYBs that regulate monoterpene-associated pathways have been identified (Reddy et al. 2017; Yang et al. 2020). Importantly, the functions of R2R3-MYBs within the same subgroup have diversified as, although the N-terminus of R2R3-MYBs is highly conserved, the C-terminal amino acids show substantial variability, which has allowed for differentiation (Li and Zachgo 2013; Karppinen et al. 2021; Lin and Rausher 2021; Ming et al. 2021).
Genes involved in plant secondary metabolism are usually randomly distributed in the plant genome, which hinders the discovery of all the genes involved in distinct biochemical pathways related to plant-specific metabolism. However, the increasing availability of plant genomes at the chromosomal level has facilitated the analysis of the evolutionary origin of and the mechanisms involved in plant-specific terpene synthesis. Lauraceae is a family of plants that includes camphor tree (Cinnamomum camphora), stout camphor tree (Stout camphor tree), mountain pepper (Litsea cubeba), and other economically and ecologically trees. These trees are highly valued owing to their abundance of monoterpenes. In particular, mountain pepper is recognized for its considerable contribution to the production of spices and pharmaceuticals through the essential oil extracted from its fruit peel. The essential oil of mountain pepper contains approximately 41 types of monoterpenes (94.4% to 98.4%). Through comparative and functional analysis of the genomes of Lauraceae species, it has been found that TPS genes play a considerable role in terpenoid biosynthesis in this family (Chaw et al. 2019; Chen et al. 2020b; Han et al. 2021; Wang et al. 2022a). Previous studies have identified the key genes in the monoterpene biosynthesis pathway in Lauraceae, including 1-deoxy-d-xylulose 5-phosphate synthase (DXS), 1-deoxy-d-xylulose-5-phosphate reductoisomerase (DXR), 3-hydroxy-3-methylglutaryl-CoA synthase (HMGS), 3-Hydroxy-3-methylglutaryl CoA Reductase (HMGR), geranyl diphosphate synthase small subunit 1 (GPPS.SSU1), and terpene synthase (TPS) (Wu et al. 2020; Zhao et al. 2020; Wang et al. 2022b; Zhao et al. 2023a). However, there are no reports to date relating to improvements in the production of citral, the most important monoterpene component of Lauraceae.
Citral is a characteristic monoterpene of the Lauraceae family and is particularly concentrated in mountain pepper, which has the highest known content of this compound. Citral exists in 2 forms, namely, α-citral (geranial) and its trans-isomer β-citral (neral), which are synthesized from geraniol or nerol, respectively, by alcohol dehydrogenases (ADHs, EC 1.1.1.1) belonging to the medium-chain oxygenase/reductase family. ADHs have been shown to function as catalysts in citral production via the removal of the hydrogen–oxygen bonds from geraniol and nerol, respectively (Iijima et al. 2006; Luddeke et al. 2012; Sato-Masumoto and Ito 2014; Gupta and Ganjewala 2015; Tan et al. 2018; Zeng et al. 2020; Ohashi et al. 2021). ADHs are present in both eukaryotes and prokaryotes, and they catalyze reciprocal transformations between alcohols and aldehydes (Strommer 2011). In 2006, Iijima et al. found that ObADH could catalyze the conversion of geraniol to α-citral in sweet basil (Ocimum basilicum). Since then, PaADH and PmADH have been reported to catalyze the synthesis of geraniol in Perilla (Sato-Masumoto and Ito 2014) and Persicaria minor (Tan et al. 2018), respectively. However, due to the high sequence similarity of ADH gene family members and the lack of high-quality genomic information, research on the involvement of ADHs in citral synthesis has been restricted to the examination of enzyme activity using recombinant proteins. Additionally, little is known about the regulatory mechanism underlying citral biosynthesis.
In this study, a Lauraceae-specific citral biosynthetic gene cluster (CGC) was identified, and was found to include genes encoding the modifying enzymes ADH28 and ADH29 as well as the regulator MYB44. The CGC was formed through species-specific tandem and proximal duplications, resulting in functional divergency in mountain pepper, camphor tree, and stout camphor tree. Functional analysis confirmed that the CGC plays a part in citral biosynthesis during the ripening of mountain pepper fruit and indicated that MYB44 contributes to these steps by directly suppressing the expression of key citral biosynthetic genes. Notably, our findings indicated that LcADH28 was primarily responsible for geranial generation in fruit, while LcADH29 preferentially catalyzed the conversion of nerol to neral. In summary, our findings indicate that the CGC is a previously uncharacterized type of terpene biosynthesis gene cluster, and thus offer insights into the evolutionary history and regulatory mechanism of citral biosynthesis in Lauraceae.
Results
Identification and characterization of LcADHs
Citral, a characteristic monoterpene, is found predominantly in Lauraceae species, particularly in L. cubeba, which has the highest known citral content. ADH enzymes play a crucial role in the biosynthesis of citral. HMM search using the ADH_N domain (PF08240) and ADH_zinc_N (PF00107) identified 36 ADH family genes in mountain pepper (Supplemental Table S1), which were classified into 3 clades based on a phylogenetic tree reconstructed using the maximum-likelihood method and gene structure analysis (Fig. 1A; Supplemental Table S2). Studies have shown that cinnamyl alcohol dehydrogenase (CAD) clade genes are involved in citral biosynthesis (Iijima et al. 2006; Sato-Masumoto and Ito 2014; Tan et al. 2018), suggesting that LcADH27-36, which was classified into Clade-III, might contribute to citral biosynthesis in mountain pepper.
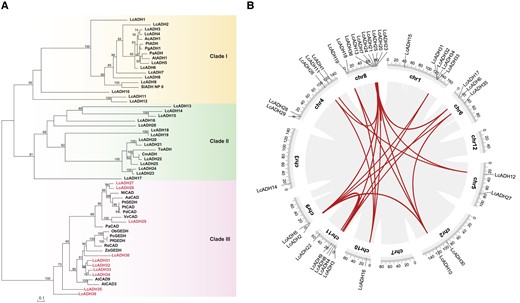
Classification of the LcADH family in Litsea cubeba. A) Maximum-likelihood tree of ADH protein sequences, including LcADHs (Supplemental Table S1) and other geraniol dehydrogenases (Supplemental Table S2). Characterized LcADHs for citral biosynthesis are indicated in clade III. B) Schematic representations of the interchromosomal relationships among LcADHs. Gray lines show synteny blocks in the L. cubeba genome; duplicated ADH gene pairs are connected by red lines.
To further investigate the mechanism underlying the expansion of ADH genes in mountain pepper despite the absence of a recent genome duplication event, we examined the chromosomal distribution of ADH genes and found that they were unevenly distributed on chromosomes (Fig. 1B). Segmental duplication events occurring in the mountain pepper ADH family were investigated by conducting a synteny analysis using BLASTp and MCScanX. Twenty-six segmental duplication events were detected involving 26 ADH genes (Fig. 1B; Supplemental Table S1). Duplicated sequences with lengths greater than 1,000 bp and identities greater than 90% were defined as segmental duplications. Recent tandem and proximal duplications were found to have led to the formation of homologous ADH gene clusters and an expansion of the ADH gene family. Specifically, the LcADH28-29 and LcADH31-34 gene clusters formed on chromosomes 4 and 1, respectively (Supplemental Fig. S1). The LcADH28-29 cluster consists of 4 genes, namely MYB44-ADH29-MRG1-ADH28 (8,510,975 to 8,584,044 bp, 73,069 bp), whereas the LcADH31-34 cluster contains 7 genes (131,420,350 to 131,757,372 bp, 337,022 bp).
In summary, these results support that duplicated ADH genes were created through tandem and proximal duplications in mountain pepper, leading to the formation of ADH gene clusters and the expansion of the ADH gene family.
Evolution and divergence of ADH gene clusters in 3 Lauraceae species
Camphor tree, stout camphor tree, and mountain pepper belong to the Cinnamomum–Litsea genus branch in Lauraceae. Our evolutionary analysis of the Lauraceae family indicated that this branch has the fastest evolution rate and is rich in monoterpenes (Chen et al. 2020b; Wang et al. 2022a). To investigate the origin and evolution of the CGC, the genomes of camphor tree, stout camphor tree, mountain pepper, maize (Zea mays), and grape (Vitis vinifera) were selected for further analysis. The syntenic regions containing the CGCs of the 5 species were shown in Fig. 2A. To identify putative orthologs of MYB44, MRG1, LcADH28, and LcADH29, we aligned the scaffolds containing the top hits to the 5 CGCs, and the matching genes in each case were plotted as a collinear line. However, no ADH28 ortholog was detected in maize or grape, although there was an ortholog of ADH29 in maize (Fig. 2B; Supplemental Fig. S2). The analysis of gene duplication patterns and phylogenetic analysis in ADHs indicated that ADH29 underwent independent evolution, while ADH28 resulted from proximal duplications within the Lauraceae family (Figs. 1B and 2B). Indeed, sequence alignment showed that the sequence similarity of LcADH28 and LcADH29 was 66.86%. In stout camphor tree, CkADH29 was annotated as a pseudogene with incomplete ADH_N domain and ADH_zinc_N (Supplemental Fig. S3). These results similarly suggest that ADH29 evolved independently, whereas ADH28 may have been retained after duplications in Lauraceae lineages.
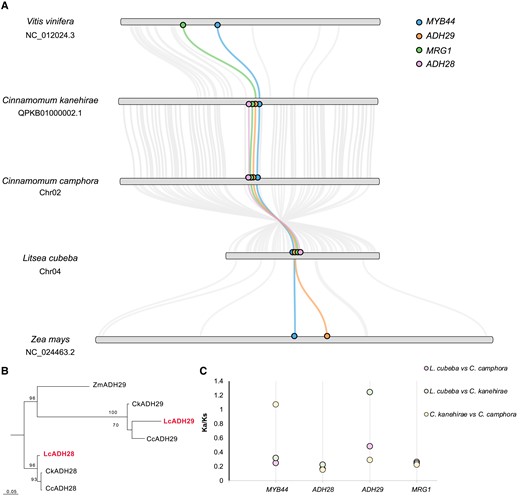
Evolutionary history of the citral biosynthetic gene cluster (CGC) in Lauraceae. A) Comparison of the CGCs from Litsea cubeba, Cinnamomum camphora, Cinnamomum kanehirae, Zea mays, and Vitis vinifera. Orthologous genes were identified by collinearity analysis and BLAST analysis between each set of 2 species. Orthologous genes are connected by gray lines. B) Maximum-likelihood tree of orthologous ADH28 and ADH29 genes in Lauraceae. C) Pairwise Ka/Ks analysis between L. cubeba, C. camphora, and C. kanehirae for the CGC.
To evaluate the strength and direction of selection pressure on the CGC during evolution, we estimated the Ks, Ka, and Ka/Ks values for each orthologous ADH gene pair in the 3 Lauraceae species (Fig. 2C; Supplemental Table S3). Purifying selection eliminates deleterious mutations while positive selection induces and fixes advantageous mutations (Hurst 2002; Navarro and Barton 2003; Yang 2007). The calculated Ka/Ks ratios for orthologous gene pairs of the CGC in each species were less than 1, indicating that purifying selection was the main driving force behind CGC evolution. However, the presence of incomplete domains in CkMYB44 and CkADH29 resulted in high Ka/Ks ratios for CcMYB44 versus CkMYB44 and CkADH29 versus LcADH29, suggesting that these genes evolved at a faster rate.
Divergence in enzymatic activities among Lauraceae ADH28 and ADH29 orthologs
Citral, a mixture of geranial and neral, is generated from the respective substrates geraniol and nerol (Iijima et al. 2006). The leaves of camphor tree and the fruits of mountain pepper have been shown to contain abundant amounts of citral (Chen et al. 2020b; Zhao et al. 2023b); however, there are no reports to date on citral in stout camphor tree. To characterize the genes involved in citral formation, the respective orthologs of ADH28 and ADH29 in the 3 Lauraceae species were cloned and expressed in Escherichia coli, and the recombinant proteins were detected by western blot (Supplemental Fig. S4). The 3 orthologs of ADH28 shared similar substrates but differed in their enzymatic activity (Fig. 3). LcADH28 could convert geraniol and nerol to geranial and neral, but had a stronger catalytic effect on geraniol. Compared with LcADH28, CcADH28 exhibited lower activity toward geraniol and nerol, while CkADH28 showed the lowest enzymatic activity among the 3 orthologs. Although it could convert geraniol to geranial, its catalytic activity against nerol was negligible. In contrast to LcADH28, LcADH29 had higher activity toward nerol than geraniol. Surprisingly, CcADH29 showed no enzymatic activity, which we speculated might be due to variation in its active site, and its substrates may be other alcohols. Consistent with the sequence analysis results, CkADH29 had no catalytic activity due to the lack of a complete structural domain.
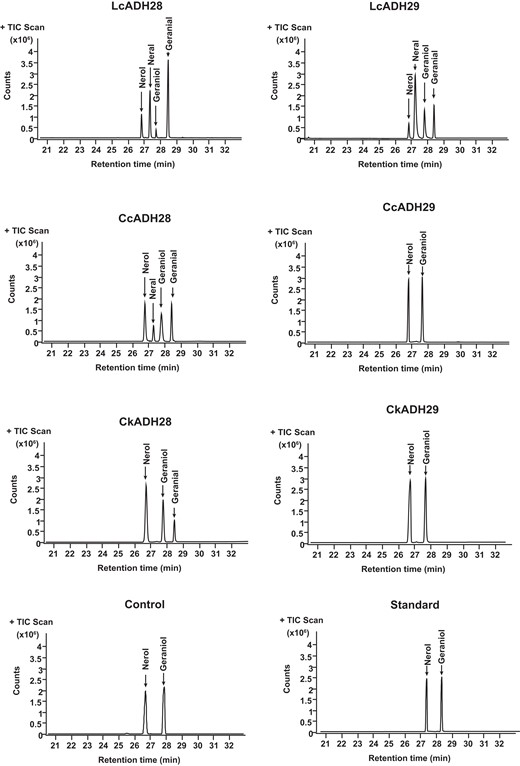
LcADHs catalyze citral biosynthesis in vitro. Identification of the enzymatic products after incubating recombinant LcADH28, LcADH29, CcADH28, CcADH29, CkADH28, and CkADH29 proteins with geraniol and nerol. Empty vector with geraniol and nerol served as controls. Recombinant enzymes were expressed in Escherichia coli (Supplemental Fig. S4). Volatile terpenes were further analyzed by gas chromatography–mass spectrometry (GC–MS) and compared with authentic standards as previously described (Si et al. 2012; Zhao et al. 2023b).
To quantify the functional differentiation of ADH28 and ADH29 in Lauraceae, we performed an enzyme kinetic analysis. As shown in Table 1, significant differences in enzyme kinetic values toward geraniol and nerol were observed between ADH28 or ADH29 in Lauraceae (P < 0.05). The Km values for LcADH28 toward geraniol and nerol were 9.01 and 24.87 μm, respectively, indicating that LcADH28 had a higher affinity for geraniol than nerol (P < 0.05). By contrast, LcADH29 showed a lower affinity for geraniol (Km = 30.60 μm) than for nerol (Km = 16.60 μm). Compared with LcADH29, LcADH28 showed markedly higher catalytic efficiency (Kcat/Km = 2.34) toward geraniol. On the other hand, the catalytic efficiency of LcADH29 toward nerol was 1.69-fold higher (Kcat/Km = 0.93) than that of LcADH28. Regarding the ADHs of camphor tree, CcADH29 could not use geraniol and nerol as substrates; however, CcADH28 exhibited catalytic activity toward geraniol (Km = 26.78 μm, Kcat/Km = 0.69) and nerol (Km = 29.25 μm, Kcat/Km = 0.27). For stout camphor tree, CkADH28 had the lowest affinity and catalytic efficiency for geraniol (Km = 45.89 μm, Kcat/Km = 0.11), while the kinetics of CkADH29 were not examined because of its incomplete catalytic domain. In addition, we found that there were significant differences in kinetic values toward geraniol and nerol between LcADH28 and CcADH28, as well as between LcADH28 and CkADH28 (P < 0.05). These results indicated that despite the high sequence conservation among orthologous ADH28 and ADH29 genes in Lauraceae, there was a prevalent divergence in either enzymatic activities or substrate specificities.
Substrate . | Geraniol . | Nerol . | ||||
---|---|---|---|---|---|---|
Km (μm) . | Kcat (S−1) . | Kcat/Km (μm−1·S−1) . | Km (μm) . | Kcat (S−1) . | Kcat/Km (μm−1·S−1) . | |
LcADH28 | 9.01 ± 1.38Bc* | 21.07 ± 0.39Aa | 2.33851276 | 24.87 ± 2.34Ab | 13.80 ± 0.32Ba | 0.5548854 |
CcADH28 | 26.78 ± 2.11Ab | 18.37 ± 0.71Ab | 0.68595967 | 29.25 ± 1.38Aa | 7.76 ± 0.61Bb | 0.26529915 |
CkADH28 | 45.89 ± 1.17a | 4.91 ± 0.42c | 0.10699499 | … | … | … |
LcADH29 | 30.60 ± 3.49A | 9.20 ± 0.19B | 0.30065359 | 16.60 ± 0.70B | 15.46 ± 0.68A | 0.9313253 |
CcADH29 | … | … | … | … | … | … |
CkADH29 | … | … | … | … | … | … |
Substrate . | Geraniol . | Nerol . | ||||
---|---|---|---|---|---|---|
Km (μm) . | Kcat (S−1) . | Kcat/Km (μm−1·S−1) . | Km (μm) . | Kcat (S−1) . | Kcat/Km (μm−1·S−1) . | |
LcADH28 | 9.01 ± 1.38Bc* | 21.07 ± 0.39Aa | 2.33851276 | 24.87 ± 2.34Ab | 13.80 ± 0.32Ba | 0.5548854 |
CcADH28 | 26.78 ± 2.11Ab | 18.37 ± 0.71Ab | 0.68595967 | 29.25 ± 1.38Aa | 7.76 ± 0.61Bb | 0.26529915 |
CkADH28 | 45.89 ± 1.17a | 4.91 ± 0.42c | 0.10699499 | … | … | … |
LcADH29 | 30.60 ± 3.49A | 9.20 ± 0.19B | 0.30065359 | 16.60 ± 0.70B | 15.46 ± 0.68A | 0.9313253 |
CcADH29 | … | … | … | … | … | … |
CkADH29 | … | … | … | … | … | … |
*Data are presented as means ± Sd of 3 replicates. Student's t-test was used to test whether there are significant differences among each ADH proteins for each substrate. Different lowercase letters indicate significantly differences among the 3 proteins of ADH orthologous for same substrate (P < 0.05). Different capital letters indicate significantly differences among the 2 substrates for same protein (P < 0.05).
Substrate . | Geraniol . | Nerol . | ||||
---|---|---|---|---|---|---|
Km (μm) . | Kcat (S−1) . | Kcat/Km (μm−1·S−1) . | Km (μm) . | Kcat (S−1) . | Kcat/Km (μm−1·S−1) . | |
LcADH28 | 9.01 ± 1.38Bc* | 21.07 ± 0.39Aa | 2.33851276 | 24.87 ± 2.34Ab | 13.80 ± 0.32Ba | 0.5548854 |
CcADH28 | 26.78 ± 2.11Ab | 18.37 ± 0.71Ab | 0.68595967 | 29.25 ± 1.38Aa | 7.76 ± 0.61Bb | 0.26529915 |
CkADH28 | 45.89 ± 1.17a | 4.91 ± 0.42c | 0.10699499 | … | … | … |
LcADH29 | 30.60 ± 3.49A | 9.20 ± 0.19B | 0.30065359 | 16.60 ± 0.70B | 15.46 ± 0.68A | 0.9313253 |
CcADH29 | … | … | … | … | … | … |
CkADH29 | … | … | … | … | … | … |
Substrate . | Geraniol . | Nerol . | ||||
---|---|---|---|---|---|---|
Km (μm) . | Kcat (S−1) . | Kcat/Km (μm−1·S−1) . | Km (μm) . | Kcat (S−1) . | Kcat/Km (μm−1·S−1) . | |
LcADH28 | 9.01 ± 1.38Bc* | 21.07 ± 0.39Aa | 2.33851276 | 24.87 ± 2.34Ab | 13.80 ± 0.32Ba | 0.5548854 |
CcADH28 | 26.78 ± 2.11Ab | 18.37 ± 0.71Ab | 0.68595967 | 29.25 ± 1.38Aa | 7.76 ± 0.61Bb | 0.26529915 |
CkADH28 | 45.89 ± 1.17a | 4.91 ± 0.42c | 0.10699499 | … | … | … |
LcADH29 | 30.60 ± 3.49A | 9.20 ± 0.19B | 0.30065359 | 16.60 ± 0.70B | 15.46 ± 0.68A | 0.9313253 |
CcADH29 | … | … | … | … | … | … |
CkADH29 | … | … | … | … | … | … |
*Data are presented as means ± Sd of 3 replicates. Student's t-test was used to test whether there are significant differences among each ADH proteins for each substrate. Different lowercase letters indicate significantly differences among the 3 proteins of ADH orthologous for same substrate (P < 0.05). Different capital letters indicate significantly differences among the 2 substrates for same protein (P < 0.05).
The CGC shows an associated expression profile and is associated with citral spatio-temporal accumulations in mountain pepper fruits
To further investigate the role of the CGC in citral synthesis in mountain pepper, the spatial expression patterns of CGC genes were analyzed using RT-qPCR. The results showed that the CGC genes were widely expressed at different fruit developmental stages. Among them, LcMYB44 and LcMRG1 exhibited the highest expression at 30 d after full bloom (DAF30) and DAF150 of the pericarp, and the lowest expression at DAF60 and DAF120 of the pericarp; additionally, there was a significant positive correlation between the expression of LcMYB44 and LcMRG1 (R = 0.89) (Fig. 4A). By contrast, the highest expression of both LcADH28 and LcADH29 was observed at DAF60 and DAF120 (Fig. 4A). LcMYB44 had a significant negative correlation with both LcADH28 and LcADH29. These results were consistent with previous studies, which also identified the peak period of citral biosynthesis in mountain pepper fruits at DAF60 and DAF120 (Chen et al. 2020b; Zhao et al. 2020; Gao et al. 2016).
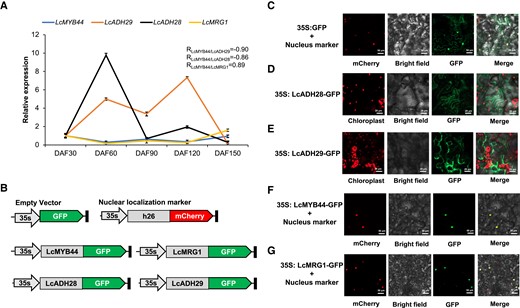
Gene expression patterns and potential roles of the citral biosynthetic gene cluster (CGC). A) The expression profiles of LcMYB44, LcADH28, LcMRG1, and LcADH29. Data are presented as means ± Sd of 3 replicates. The Litsea cubeba ubiquitin-conjugating enzyme (UBC) gene served as the internal control (Lin et al. 2013). DAF, days after full bloom. B) to G) Subcellular localization of LcMYB44, LcADH28, LcMRG1, and LcADH29 in Nicotiana benthamiana leaf cells.
The subcellular localization of LcMYB44, LcADH28, LcMRG1, and LcADH29 was examined via the transient expression of each as a fusion protein in Nicotiana benthamiana leaves (Fig. 4B). The results showed that LcMYB44 was localized to the nucleus (Fig. 4C), which is consistent with its role as a TF. MRG1, which is encoded by the MORF-RELATED GENE and functions as a reader of histone H3 lysine 36 trimethylation, was also localized to the nucleus. LcADH28 and LcADH29 were detected in the cytoplasm, in accordance with their roles as geraniol or nerol dehydrogenases (Fig. 4C).
Overexpression of LcADH28 and LcADH29 enhance citral biosynthesis in mountain pepper
The roles of LcADH28 and LcADH29 in citral biosynthesis were further validated through their transient overexpression in mountain pepper leaves, which resulted in a significant increase in the expression levels of both genes (approximately 5.4- and 7.3-fold, respectively) compared with that in control leaves (Fig. 5A). The content of geranial and neral in leaves increased significantly after LcADH28 infiltration, with 11.54 and 4.11 μg g−1 respectively, compared to leaves infiltrated with empty vector, which had 5.50 and 2.74 μg g−1 respectively (Fig. 5B). Additionally, the overexpression of LcADH29 resulted in significant 1.8-fold and 1.7-fold increases in geranial (9.56 μg g−1) and neral (4.90 μg g−1) production (Fig. 5B). Congruent with the results of the enzymatic activity analyses, LcADH28 showed a preference for geraniol as a substrate, while LcADH29 displayed a stronger preference for nerol. These findings suggested that the introduction of LcADH28 or LcADH29 can lead to an increase in citral biosynthesis using geraniol and nerol as substrates, both in vivo and in vitro.
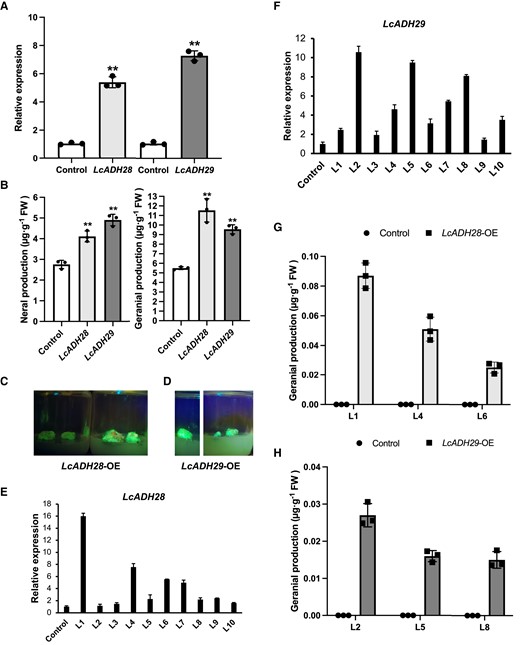
LcADHs catalyze citral biosynthesis in vivo. A) Transient overexpression of LcADH28 and LcADH29 in Litsea cubeba leaves (Wang et al. 2022a). After infiltration, the plants were grown for 2 d and the expression levels of LcADH28 and LcADH29 were confirmed. B) The overexpression of LcADH28 and LcADH29 significantly increased the concentration of citral. Gas chromatography–mass spectrometry (GC–MS) analysis was performed as previously described (Si et al. 2012; Zhao et al. 2023a). C) Phenotypes of LcADH28 in transgenic mountain pepper lines. D) Phenotypes of LcADH29 in transgenic mountain pepper lines. E) The expression levels of LcADH28 in transgenic L. cubeba lines. F) The expression levels of LcADH29 in transgenic L. cubeba lines. G) and H) Citral contents in transgenic L. cubeba calluses. All data are presented as means ± Sd of 3 replicates (**P < 0.01).
Transgenic mountain pepper lines were created that stably overexpressed LcADH28 or LcADH29, respectively. Positive transgenic lines were screened using GFP fluorescence, PCR, and Southern blotting (Fig. 5, C and D; Supplemental Fig. S5), with LcADH28-OE-1, 4, and 6 and LcADH29-OE-2, 5, and 8 being selected for subsequent experiments as they showed the highest expression of the respective genes (Fig. 5, E and F). As shown in Fig. 5, G and H, there was a substantial increase in geranial concentrations in transgenic mountain pepper calluses. The LcADH28-OE lines displayed geranial concentrations of 0.087, 0.051, and 0.025 μg g−1, and no geranial was detected in the control line. In the LcADH29-OE lines, the geranial concentrations were 0.027, 0.016, and 0.015 μg g−1. The overexpression of LcADH28 and LcADH29 did not influence neral biosynthesis. Collectively, these results indicated that LcADH28 and LcADH29 encode ADH enzymes responsible for citral biosynthesis in mountain pepper.
LcMYB44 suppresses the transcription of LcADHs by directly binding to their promoter regions
As previously reported, several MYB regulators are involved in plant monoterpene biosynthesis (Reddy et al. 2017; Yang et al. 2020). Sequence alignment analyses revealed that LcMYB44 has an N-terminal R2R3-MYB domain and 2 signature motifs and was thus categorized into subgroup 22 (Fig. 6A). In plants, MYB is instrumental in controlling secondary metabolism by binding to the MYB core, MBS and other MYB recognition elements (MREs) in the promoters of genes involved in secondary metabolite biosynthesis (Stracke et al. 2001). To identify the presence of MREs, we used the PlantCARE database (http://bioinformatics.psb.ugent.be/webtools/plantcare/html/) to screen the promoter sequences of CGC genes. As shown in Supplemental Fig. S6, the promoters of CGC had several MREs, including C/TAACT/AG, AACCTAA, and CCGTGG elements.
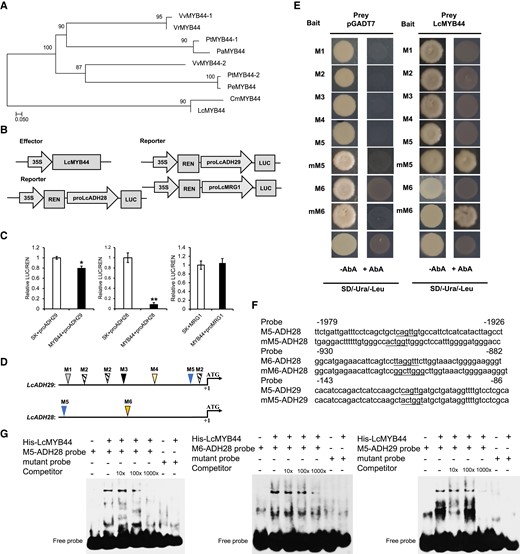
LcMYB44 binds to the promoters of LcADH28 and LcADH29 and represses their expression. A) Maximum-likelihood tree of MYB44 protein sequences, including LcMYB44 (Supplemental Table S2). B) The MYB recognition elements (MREs) in the citral biosynthetic gene cluster (CGC). C) Dual-luciferase (dual-LUC) reporter assay. Schematic diagrams of the effector (pGreenII 62-SK-LcMYB44) and reporter (35S:REN-proLcADH28-LUC, 35S:REN-proLcADH29-LUC, 35S:REN-proLcMRG1-LUC) constructs used in the assay. Relative luciferase (LUC) activity was normalized to the activity of the reference Renilla (REN) luciferase. LcMYB44 significantly inhibited LcADH28 and LcADH29 expression compared with the control. Data are presented as means ± Sd of 3 replicates (*P < 0.05, **P < 0.01). D) and E) LcMYB44 binds to the M5 (CAGTTG) and M6 (TTAGGTTT) regions of the LcADH28 and LcADH29 promoters, based on the yeast one-hybrid (Y1H) assays. Three repeats of MREs were used as baits (pAbAi-3 × MRE). Yeast cells co-expressing pGADT7-LcMYB44 and pAbAi-3 × MRE were cultured for 2 d at 30 °C in selective medium (SD/-Trp/-Ura) containing 400 ng mL−1 AbA. F) Probes: M5 and M6 LcADH28 and LcADH29 promoter regions. G) Interactions between LcMYB44 and the promoters of LcADH28 and LcADH29. The His-LcMYB44 fusion protein and the labeled probe were used. Lanes 1 to 5 contained M5 or M6 probes, and lanes 6 and 7 contained mutant probes. Unlabeled probe was used as a competitor.
To ascertain the mechanism by which LcMYB44 regulates LcADH28 and LcADH29 expression, a dual-luciferase assay was performed using proLcADH28-LUC, proLcADH29-LUC, and proLcMRG1-LUC reporters, respectively, with LcMYB44 serving as the effector (Fig. 6B). The results revealed that LcMYB44 repressed the expression of both LcADH28 and LcADH29 (Fig. 6C). Moreover, LcMYB44 inhibited the expression of LcADH28, which contained only 2 MREs, to a substantially greater extent than that seen for LcADH29, which contained 6 MREs. In addition, LcMYB44 could not activate the promoter of LcMRG1. Further research is needed to reveal the function of LcMRG1 in the CGC.
The binding of LcMYB44 to MREs on the promoters of both LcADH28 and LcADH29 was investigated through yeast one-hybrid (Y1H) assays in which triple-repeated MREs were integrated into the genome of yeast cells (Fig. 6D). The results demonstrated that LcMYB44 could bind to 3 tandem repeats of the CAGTTG and AACCTAA elements, but not to TAACTG, CAACAG, CCGTGG, or mutant elements (Fig. 6E). The interaction between LcMYB44 and the CAGTTG or AACCTAA element strongly activated the expression of the aureobasidin A (AbA) resistance gene, allowing the yeast to grow normally on the selective SD/-Ura/-Leu medium.
To further examine LcMYB44 binding activity, we conducted an electrophoretic mobility shift assay (EMSA) using a recombinant LcMYB44 protein and the 48-bp promoter fragments of LcADH28 and LcADH29 that contained the MREs (Fig. 6F; Supplemental Fig. S7). As shown in Fig. 6G, the CAGTTG and AACCTAA elements of the LcADH28 promoter and the CAGTTG element of the LcADH29 promoter were all recognized by LcMYB44. These results demonstrated that LcMYB44 suppresses the transcription of LcADH28 and LcADH29 by directly binding to the CAGTTG and AACCTAA in their promoter regions.
Suppression of LcMYB44 promotes citral biosynthesis in mountain pepper
To clarify the regulatory role of LcMYB44 in citral biosynthesis, the virus-induced gene silencing (VIGS) method was employed to suppress its expression in mountain pepper. Fifteen days after injection of the TRV-LcMYB44 vector, the growth status and terpene accumulation were examined. The mountain pepper seedlings grew normally, and the expression of LcMYB44 was confirmed to be decreased in these seedlings compared with that in control plants. This decrease in LcMYB44 expression was accompanied by a significant upregulation of LcADH28 and LcADH29 expression (Fig. 7, A and B). Furthermore, the accumulation of citral and other terpenes was significantly enhanced in the seedlings (Fig. 7, C and D), suggesting that LcMYB44 is an important regulator of citral biosynthesis. The expression of LcDXS4, LcDXR, LcHMGS1, LcGPPS.SSU1, and LcTPS42, which encode key enzymes in terpene biosynthesis that contain MREs in their promoters, was also upregulated (Fig. 7E). The increase in terpene production in the TRV-LcMYB44 lines was accompanied by 3.9-, 1.7-, 1.9-, 3.3-, and 1.8-fold increases in the expression levels of LcDXS4, LcDXR, LcHMGS1, LcGPPS.SSU1, and LcTPS42, respectively.
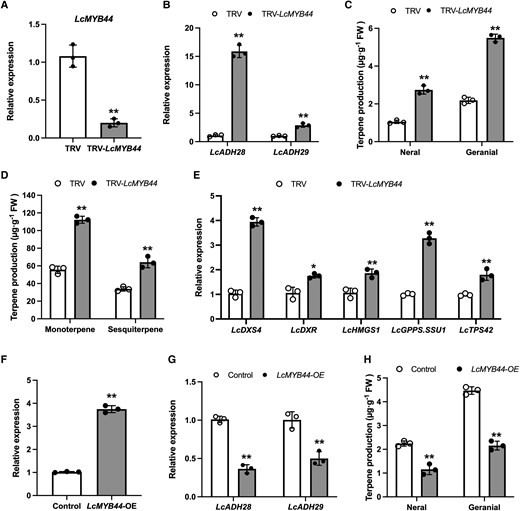
Silencing LcMYB44 using virus-induced gene silencing (VIGS) increases the contents of citral and those of other terpenes in Litsea cubeba. A) The relative expression levels of LcMYB44 as determined by RT-qPCR. B) The relative expression levels of LcADH28 and LcADH29.C) The relative contents of citral in control plants (TRV) and VIGS plants (TRV-LcMYB44). D) The relative contents of terpenes in control plants (TRV) and VIGS plants (TRV-LcMYB44). E) The relative expression levels of LcDXR, LcDXS4, LcHMGS1, LcGPPS.SSU1, and LcTPS42 as determined by RT-qPCR. F) Transient overexpression of LcMYB44 in L. cubeba leaves. The relative expression levels of LcMYB44 as determined by RT-qPCR. G) The relative expression levels of LcADH28 and LcADH29 as determined by RT-qPCR. H) The overexpression of LcMYB44 significantly decreased the concentration of citral. Data are presented as means ± Sd from 3 biological replicates. Asterisks indicate significant differences between the compared lines under the same growth conditions (*P < 0.01; **P < 0.01).
The expression level of LcMYB44 was increased by approximately 3.7-fold after its transient expression, concomitant with a significant decrease in LcADH28 and LcADH29 expression (Fig. 7, F and G). The overexpression of LcMYB44 also led to a significant decrease in geranial and neral production. The geranial and neral content of leaves after LcMYB44 infiltration was 2.15 and 1.14 μg g−1, respectively, representing a significant (P < 0.01) decrease compared with that in leaves (4.49 and 2.25 μg g−1) infiltrated with the empty vector (Fig. 7H). These results suggested that LcMYB44 may have a negative impact on both the CGC and the specific terpene biosynthesis pathway genes in mountain pepper.
Discussion
This study identified a Lauraceae-specific citral biosynthesis gene cluster, containing MYB44 as the regulator and 2 ADHs that function as modifying enzymes, and found that the CGC exhibits divergence in Lauraceae. LcADH28 and LcADH29 are responsible for catalyzing the conversion of geraniol and nerol to geranial (α-citral) and neral (β-citral). Notably, LcADH28 showed a significant preference for geraniol as the substrate (P < 0.05), while LcADH29 prioritized the catalysis of nerol. LcMYB44 suppressed the expression of LcADH28 and LcADH29 by binding to the CAGTTG and CTTAGGTTT sites on their respective promoter. During citral biosynthesis in mountain pepper fruits, a decrease in LcMYB44 expression resulted in the upregulation of LcADH28 and LcADH29, as well as other genes involved in the production of this monoterpene biosynthesis, leading finally to enhanced citral accumulation. These findings highlight the crucial role of CGC in the regulation of citral biosynthesis (Fig. 8).
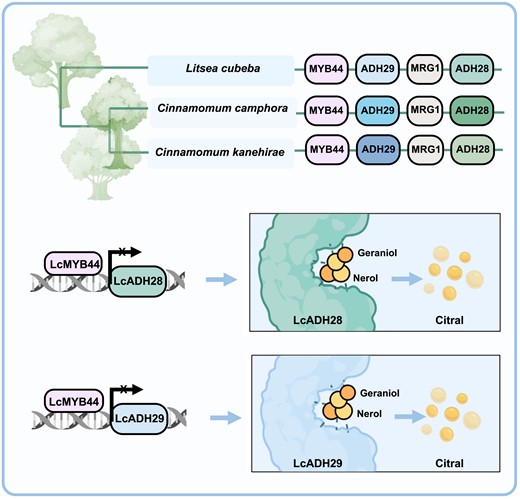
Proposed model for the evolution and the regulatory mechanisms involved in citral biosynthesis in Litsea cubeba. The Lauraceae-specific citral gene cluster comprises MYB44 as a regulator and 2 alcohol dehydrogenases (ADHs) as modifying enzymes. The divergence of ADH28 and ADH29 enzyme activity and substrate affinity has led to differences in the accumulation of citral in Lauraceae. LcMYB44 suppresses the LcADH28 and LcADH29 genes by directly binding to their promoters (created with BioRender.com).
Plants synthesize more than 1 million small-molecule metabolites (Fang et al. 2019). Terpenes, which comprise one of the most important and largest classes of natural products, participate in the exchange of information between plants and their environment, pollination, and resistance to both biotic and abiotic stresses throughout the lifetime of plants. Simultaneously, terpenes constitute an indispensable source of flavor, fragrance, and medicinal materials for human use (McGarvey and Croteau 1995; Tholl and Lee 2011; Pichersky and Raguso 2018; Fang et al. 2019).
Plant genomes exhibit considerable plasticity. Over recent years, various natural-product biosynthesis gene clusters, such as those of momillactones in Calohypnum plumiforme, zealexin in maize, and tomatine in tomato, have been discovered and found to involve terpenoids, alkaloids, fatty acids, and other natural products; these gene clusters are summarized in detail in recent reviews (Liu et al. 2020b; Zhan et al. 2022). These clusters, which contain at least 1 functional gene, offer a unique perspective on metabolic diversification and innovation. They may have formed following gene duplication (segmental or tandem) events, resulting in gene neofunctionalization, subfunctionalization, and nonfunctionalization (pseudogenes). However, these gene clusters predominantly consist of biosynthetic enzymes and modifying enzymes; the presence of a regulator occurs less frequently (Forman et al. 2022; Gerin et al. 2021). Like LcMYB44, the AcOTAbZIP gene directly regulates the OTA biosynthesis gene in the gene cluster in Aspergillus.
Our previous study presented a chromosome-level assembly of the genome of mountain pepper—the core-member of the Lauraceae—and verified the function of monoterpene synthases responsible for the production of specific volatile compounds in Lauraceae (Chen et al. 2020b). The clustering of specialized biosynthetic pathways suggests that these pathways are subject to specific selective pressures and likely underlie important traits that enhance fitness (Chen et al. 2020b). Based on our study, ADH genes that dynamically participate in the synthesis of specific compounds such as citral can combine with MYB in various Lauraceae lineages, resulting in the creation of monoterpene biosynthesis pathways (Fig. 2, A and C). Furthermore, this suggests that the CGC must have been assembled in relatively recent evolutionary periods.
Plant biosynthetic gene clusters usually consist of genes that encode biosynthetic enzymes, modifying enzymes, and transporters, among others (Itkin et al. 2013; Liu et al. 2016; Liu et al. 2020a; Reichardt et al. 2020; Kitaoka et al. 2021; Gain et al. 2022). Numerous studies have investigated the evolution of individual enzymes, which has led to metabolic diversity (Chen et al. 2011; Saladié et al. 2014; Yang et al. 2019). The evolution of ADHs occurred through gene subfunctionalization after recent duplication events or the neofunctionalization of ancient duplicated genes. Our findings suggested that recent tandem and proximal duplications resulted in the generation of duplicated LcADH genes, which have contributed to the formation of homologous ADH gene clusters and the expansion of the ADH gene family (Figs. 1B and 2C). The LcADH28 gene may have originated through proximal replication, whereas LcADH29 likely evolved independently. The higher fruit-specific expression of LcADH28 could be linked to its subfunctionalization, which suggests that its high expression determines the specific synthesis of citral in the fruit of mountain pepper.
In addition, the functional divergence of orthologous ADH genes has not been previously investigated in Lauraceae. In this study, despite the high sequence conservation among orthologous ADH28 and ADH29 genes in Lauraceae, we found that there was prevalent divergence in either enzymatic activities or substrate specificities among the encoded products. Differences in the ecological niches of mountain pepper, camphor tree, and stout camphor tree may have led to the divergence of enzymatic functions among the orthologous ADH genes in the Lauraceae family and led directly to citral enrichment in mountain pepper (Yang et al. 2019).
The nature of the interaction between TFs and enzyme-coding gene targets varies across species (Zhang et al. 2015; Li et al. 2017; Ding et al. 2020; Jing et al. 2020; Fu et al. 2021; Zheng et al. 2021). In the case of terpene synthetic pathways, these genes are regulated by 1 or more MYB genes. Although MYBs have been predicted to regulate monoterpene synthesis in numerous plants, including mint and camphor, our understanding of the underlying mechanism remains limited (Reddy et al. 2017; Yang et al. 2020). Nonetheless, in spearmint (Mentha spicata), MsMYB was identified as a negative regulator of monoterpene biosynthesis. When expressed in sweet basil (O. basilicum) or N. benthamiana, MsMYB disrupted the production of sesquiterpene and diterpene-derived metabolites (Reddy et al. 2017). MYB44 and its homologs belong to subgroup 22 of the R2R3-MYB family and are involved in biotic and abiotic stress responses (Li et al. 2013; Persak and Pitzschke 2014; Qiu et al. 2019; Wei et al. 2020). IbMYB44 of sweet potato (Ipomoea batatas), which functions as a repressor, inhibits anthocyanin biosynthesis by blocking the formation of IbMYB340-IbbHLH2-IbNAC56a regulatory complexes (Wei et al. 2020). Here, we identified a regulatory network based on the formation of a Lauraceae-specific gene cluster, in which genes that make up a biosynthetic pathway are typically co-expressed. We found that MYB44 can suppress the expression of ADHs and that the expression of MYB44 and that of the ADH genes was negatively associated at different stages of mountain pepper fruit development. Gene clusters related to secondary metabolite synthesis can enhance the cooperative expression of specific members in particular tissues and periods, leading to improved efficiency in metabolite synthesis and the stable inheritance of beneficial genetic traits (Liu et al. 2020b). From these patterns, the target specificity of the R2R3-MYB TF usually evolves rapidly, which means that LcMYB44 is unable to recognize all MYB binding elements of LcADHs despite the formation of a gene cluster.
In summary, in this study, we identified a Lauraceae-specific citral biosynthesis gene cluster in which MYB44 acts as a regulator and 2 ADHs act as modifying enzymes. This gene cluster exhibits divergence in Lauraceae. LcADH28 and LcADH29 catalyze the conversion of geraniol and nerol to geranial and neral, respectively. Notably, LcADH28 prefers geraniol as the substrate, while LcADH29 prioritizes nerol. LcMYB44 inhibits the expression of LcADH28 and LcADH29 by binding to the MRE sites in their respective promoters. The specific pathways involved in citral biosynthesis may contribute to important characteristics that enhance adaptability. The findings from this study relating to the CGC provide insights into the evolution of and the regulatory mechanisms underlying plant terpene biosynthesis.
Materials and methods
Plant material and treatment
Mountain pepper (L. cubeba) samples were collected from farming fields in the Fuyang district of HangZhou City, China (30°27′94″N, 119°58′43″E). Different tissues including roots, stems, leaves, and flowers, as well as fruit (at different stages of development: DAF30, 60, 90, 120, and 150) of 5-yr-old mountain pepper plants were gathered in 3 replicates. The samples were rapidly frozen and stored at −80 °C for subsequent experiments. N. benthamiana plantlets were grown in a greenhouse under a 16-h light/8-h dark photoperiod at 24 °C.
Gene identification and phylogenetic analysis
Full-length deduced amino acid sequences of LcADHs were identified in mountain pepper, camphor tree (C. camphora), and stout camphor tree (Cinnamomum kanehirae) genomes by Pfamscan based on the HMMER suite (http://hmmer.janelia.org/) using the Pfam profiles of PF08240 and PF00107 as queries (E value < 10−5) (Chaw et al. 2019; Chen et al. 2020b; Shen et al. 2022). Sequences were aligned using CLUSTAL OMEGA (https://www.ebi.ac.uk/Tools/msa/clustalo/). Maximum-likelihood phylogenetic trees were reconstructed using CIPRES (https://www.phylo.org) with the JTT model using 1,000 bootstrap replicates. To investigate the evolutionary history of the citral gene cluster, synteny analysis was performed with all BLASTp results as input for blocks with homology (70% sequence similarity) using the Multiple Collinearity Scan toolkit (MCScanX). The genomes used in synteny analysis include camphor tree (C. camphora) (Shen et al. 2022), stout camphor tree (GCA_003546025.1), maize (Z. mays) (v.2.1), and grape (V. vinifera) (V12X). The Ka/Ks of the ADH28, ADH29, and MYB44 coding sequences were evaluated by PAML (codeml) (http://abacus.gene.ucl.ac.uk/software/paml.html).
RNA extraction and gene expression analysis
Total RNA was extracted from fruits at different development stages of Mountain pepper. RNA extraction and reverse transcription were performed as described by Chen et al. (2020b). Mountain pepper ubiquitin-conjugating enzyme (UBC) gene served as the internal control (Zhao et al. 2020). Three biological and 3 technical replicates were implemented for each gene. The primers used in this study are shown in Supplemental Table S4.
Protein subcellular localization
To determine the subcellular localization of the LcADHs and LcMYB44 proteins, recombinant pCambia1300S-GFP plasmids or empty vector control were transferred into Agrobacterium tumefaciens GV3101 cells, and then transformed into N. benthamiana leaves. GFP signals were observed at an excitation wavelength of 488 nm using a ZEISS LSM-700 confocal laser scanning microscope after 48 h of incubation (Wang et al. 2022c).
Expression of recombinant LcADHs in E. coli and enzymatic assays
The expression of recombinant protein and enzymatic assays were carried out as previously described (Iijima et al. 2006). To determine the enzymatic activity of LcADHs, CcADHs, and CKADHs, the full-length CDS of these genes was cloned and separately inserted into the pET32a vector. The recombinant plasmids were transferred into E. coli ER2566 (Weidi, China), and recombinant proteins induction and purification were performed as previously described. The recombinant proteins were detected by SDS-PAGE and western blot analysis with an anti-His-Tag antibody as previously described (Wu et al. 2016).
For in vitro enzymatic assays, recombinant protein was incubated with 50 mm Tris buffer (pH 7.0), 1 mm NADP+, and 0.1 mm geraniol or 0.1 mm nerol at pH 9.0 for 1 h at 30 °C (Tan et al. 2018). Analysis of volatiles was carried out by gas chromatography–mass spectrometry (GC–MS) as previously described with some optimizations (Si et al. 2012; Zhao et al. 2023b). The details of the GC–MS analysis are provided in Supplemental Note S1 in supplemental data. To identify geranial and neral, the retention times were compared with authentic standards, electron ionization mass spectra, and the Kovats retention index (RI). The standards included nerol (97%), geraniol (98%), neral (98%), and geranial (98%) were purchased from Sigma-Aldrich Co. (St. Louis, Missouri, USA) and Supelco (Bellefonte, Pennsylvania, USA). Three biological replicates were performed for enzyme activity analysis.
Analysis of enzyme kinetic
The steady-state kinetic parameters of recombinant ADH proteins were determined by the methods described by Iijima et al. (2006). Geraniol and nerol concentrations ranging from 1 to 100 μm were used to determine the Km values for the ADHs. The kinetic parameters (Michaelis–Menten constant, Km) for the substrates were determined.
Transient overexpression assays
Five-wk-old mountain pepper tissue culture seedlings were employed for transient overexpression. To create the overexpression constructs, the full-length CDSs of LcADH28 and LcADH29 were amplified by PCR and inserted into the pCambia1300S vector. The recombinant plasmids or empty vector were transformed into A. tumefaciens LBA4404 cells, subsequently resuspended in infiltration buffer (10 mm MES, 10 mm MgCl2, 100 mm acetosyringone, pH 5.7), and allowed to grow to an OD600 of 0.6. After incubation at room temperature for 2 to 3 h, sterile seedling leaves were vacuum-infiltrated for 30 min, and then cultured on MS medium at 25 °C under a 16-h light/8-h dark photoperiod for 48 to 60 h. Three independent lines were generated for both ADH28 and ADH29. Leaves were collected for GC–MS and RT-qPCR analysis.
Construction and transformation of mountain pepper
To further study the gene function in Lauraceae, rather than using the model plant, a stable mountain pepper transformation system was generated. The details of the system are provided in Supplemental Note S2 in supplemental data. The overexpression constructs or the empty vector were transferred into A. tumefaciens LBA4404 for mountain pepper transformation. After selection with hygromycin, 10 independent transformed lines of LcADH28, LcADH29, and control were obtained and validated by genomic DNA PCR using gene-specific primers (Supplemental Table S4). Subsequently, the calluses of transgenic mountain pepper were collected for RNA isolation and GC–MS analysis.
Dual-luciferase (dual-LUC) assay
Dual-LUC analysis experiments were used to identify MYB interaction with promoters of LcADHs as previously described (Hellens et al. 2005). The full-length CDS of LcMYB44 was amplified by PCR using gene-specific primers (Supplemental Table S4) and inserted into the pGreenII62-SK vector. The 2 promoter fragments for LcADH28 and LcADH29 were amplified and inserted into the pGreenII 0800-Luc vector.
Y1H assay
Y1H assays were performed using the Matchmaker Gold Yeast One-Hybrid System (Clontech, Shiga, Japan). pGADT7-prey plasmids containing LcMYB44 and the pGADT7 vector containing 3 tandem copies of the MREs or the mutated element were generated. pAbAi-bait and pGADT7-prey plasmids were co-transformed and selected on SD/-Leu/-Ura plates with 400 ng/mL aureobasidin A (AbA) (TaKaRa, Japan).
Electrophoretic mobility shift assay (EMSA)
EMSA was performed according to the EMSA kit manufacturer's instructions (Beyotime, China). In competitive EMSA experiments, biotin-labeled probes of MREs in each ADH promoter, unlabeled wild-type and mutant probes were synthesized, and then incubated with LcMYB44 protein in binding buffer at room temperature for 30 min. The entire reaction mixtures were run on a non-denaturing 0.5× TBE 6% (v/v) polyacrylamide gel for 1 h (60 V, 4 °C), transferred onto Biodyne B nylon membranes (Pall Corporation) and finally cross-linked using UV-light.
Virus-induced gene silencing (VIGS)
To analyze the function of LcMYB44 in mountain pepper, a VIGS experiment was conducted as previously documented (Karppinen et al. 2021). The details of the VIGS system are provided in Supplemental Note S3 in supplemental data (Supplemental Fig. S8). The pTRV-LcMYB44 vector or the empty pTRV-vector was injected into mountain pepper. Three independent lines were constructed for LcMYB44 and the control, respectively. The samples were collected after 21 d and stored at −80 °C until used for RNA extraction and GC–MS analysis.
Statistical analysis
A minimum of 3 biological replicates was utilized for all experimental conduct. The experimental data was tested with Student's t-test as well as 1-way or 2-way ANOVA employing GraphPad Prism 10.0 software. Significant differences are denoted by asterisks (*) (*P < 0.05, **P < 0.01); different letters indicate significant differences between groups (P < 0.05).
Accession numbers
Completed CDS and promoter region of LcMYB44, LcADH28, LcMRG1, and LcADH29 were uploaded into GenBank (https://www.ncbi.nlm.nih.gov/WebSub/) (Accession No. OR130223-OR130230). The accession numbers of sequences used for phylogenetic analysis were listed in Supplemental Table S2.
Acknowledgments
We are highly thankful to Professor Zhilin Yuan, Hengfu Yin and Zhenhua Liu, Dr. Minyan Wang, and Bin Liu for their helpful suggestions.
Author contributions
Y.W., Y.Z., and Y.C. planned and designed the research. Y.Z., Y.C., and M.G. performed experiments, and Y.Z. conducted fieldwork, analyzed data, and etc. Y.Z. wrote the manuscript.
Supplemental data
The following materials are available in the online version of this article.
Supplemental Note S1. GC–MS analysis.
Supplemental Note S2. Establishment of Agrobacterium-mediated genetic transformation system of L. cubeba.
Supplemental Note S3. Virus-induced gene silencing (VIGS) in L. cubeba.
Supplemental Figure S1. The LcADH28-29 and LcADH31-34 gene clusters located on chromosomes 4 and 1.
Supplemental Figure S2. Maximum-likelihood tree of ADH protein sequences.
Supplemental Figure S3. Amino acid alignments of ADH28 and ADH29 from Lauraceae generated by ClustalX.
Supplemental Figure S4. Purification and western blot of ADHs.
Supplemental Figure S5. Southern blot of genomic DNA from transgenic LcADH28-OE and LcADH29-OE L. cubeba calluses.
Supplemental Figure S6. MYB recognition elements in promoters of CGC cluster.
Supplemental Figure S7. Purification and western blot of LcMYB44.
Supplemental Figure S8. Silencing of LcPDS by virus-induced gene silencing (VIGS) in L. cubeba.
Supplemental Table S1.LcADHs gene family list.
Supplemental Table S2. Sequences used for phylogenetic analysis.
Supplemental Table S3. The rate of Ka/Ks between CGC orthologs.
Supplemental Table S4. Primers used in the study.
Funding
This work was supported by the National Natural Science Foundation of China (32101561, 32071804) and the National Key R&D Program of China (2022YFD2200601).
Data availability
All data generated during this study are included in this article and the Supplemental data.
Dive Curated Terms
The following phenotypic, genotypic, and functional terms are of significance to the work described in this paper:
References
Gao M, Lin LY, Chen YC, Wang YD. Digital gene expression profiling to explore differentially expressed genes associated with Terpenoid Biosynthesis during fruit development in Litsea cubeba. Molecules. 2016:21(9):1251. https://doi.org/10.3390/molecules21091251
Gerin D, Garrapa F, Ballester AR, González-Candelas L, De Miccolis Angelini RM, Faretra F, Pollastro S. Functional role of Aspergillus carbonarius AcOTAbZIP Gene, a bZIP transcription factor within the OTA gene cluster. Toxins (Basel). 2021:13(2):111. https://doi.org/10.3390/toxins13020111
Author notes
The author responsible for distribution of materials integral to the findings presented in this article in accordance with the policy described in the Instructions for Authors (https://dbpia.nl.go.kr/plphys/pages/General-Instructions) is Yangdong Wang ([email protected]).
Conflict of interest statement. None declared.