-
PDF
- Split View
-
Views
-
Cite
Cite
Ke Mao, Jie Yang, Yunxia Sun, Xin Guo, Lina Qiu, Quanlin Mei, Na Li, Fengwang Ma, MdbHLH160 is stabilized via reduced MdBT2-mediated degradation to promote MdSOD1 and MdDREB2A-like expression for apple drought tolerance, Plant Physiology, Volume 194, Issue 2, February 2024, Pages 1181–1203, https://doi.org/10.1093/plphys/kiad579
- Share Icon Share
Abstract
Drought stress is a key environmental factor limiting the productivity, quality, and geographic distribution of crops worldwide. Abscisic acid (ABA) plays an important role in plant drought stress responses, but the molecular mechanisms remain unclear. Here, we report an ABA-responsive bHLH transcription factor, MdbHLH160, which promotes drought tolerance in Arabidopsis (Arabidopsis thaliana) and apple (Malus domestica). Under drought conditions, MdbHLH160 is directly bound to the MdSOD1 (superoxide dismutase 1) promoter and activated its transcription, thereby triggering reactive oxygen species (ROS) scavenging and enhancing apple drought tolerance. MdbHLH160 also promoted MdSOD1 enzyme activity and accumulation in the nucleus through direct protein interactions, thus inhibiting excessive nuclear ROS levels. Moreover, MdbHLH160 directly upregulated the expression of MdDREB2A-like, a DREB (dehydration-responsive element binding factor) family gene that promotes apple drought tolerance. Protein degradation and ubiquitination assays showed that drought and ABA treatment stabilized MdbHLH160. The BTB protein MdBT2 was identified as an MdbHLH160-interacting protein that promoted MdbHLH160 ubiquitination and degradation, and ABA treatment substantially inhibited this process. Overall, our findings provide insights into the molecular mechanisms of ABA-modulated drought tolerance at both the transcriptional and post-translational levels via the ABA–MdBT2–MdbHLH160–MdSOD1/MdDREB2A-like cascade.
Introduction
Drought stress is one of the main environmental factors affecting plant growth and development; it limits the productivity, quality, and geographic distribution of crops (Wang et al. 2003; Fujita et al. 2006; Zhu 2016). Drought stress leads to a series of physiological and morphological changes in plants, such as leaf wilting, growth retardation, increased reactive oxygen species (ROS) and malondialdehyde (MDA) accumulation, decreased root vitality and photosynthesis, and even plant death in severe cases (Shinozaki and Yamaguchi-Shinozaki 2007; Anjum et al. 2011; Dietz et al. 2016; Gupta et al. 2020). Plants have evolved complex intrinsic mechanisms to adapt to drought conditions. In response to drought, abscisic acid (ABA) accumulates in plants, and the ABA signaling pathway is activated, which induces various molecular and physiological responses, such as the expression of drought-response transcription factors (TFs), accumulation of osmotic modulators, increased antioxidant enzyme activity, stomatal closure, and enhanced growth of lateral roots; these changes alleviate the adverse effects of drought stress (Dietz et al. 2016; Sahay et al. 2019; Singh et al. 2021).
ROS, such as superoxide anion (O2−), hydrogen peroxide (H2O2), and hydroxyl radical (OH−), are constantly produced as byproducts of various metabolic processes in plants and continuously reduced/scavenged by the plant antioxidative defense system so that they are maintained at steady-state levels (Edreva 2005). Drought stress breaks the dynamic balance of ROS production and removal in plants, which leads to the excessive accumulation of intracellular ROS and induces oxidative damage to cell structures (Hunyadi 2019; Dong et al. 2022). Plants counteract ROS toxicity through enzymatic antioxidant systems that comprise a range of ROS scavengers, such as superoxide dismutase (SOD), peroxidase (POD), catalase (CAT), and nonenzymatic metabolites, such as ascorbic acid, glutathione, proline, carotenoids, and flavonoids (Suzuki et al. 2012; Huang et al. 2013). Numerous studies have shown that increased antioxidant enzyme activity helps alleviate the adverse effects of drought stress (Santos et al. 2023) in various plants such as Arabidopsis (Arabidopsis thaliana) (Liu et al. 2023a), rice (Oryza sativa) (Yang et al. 2009), tobacco (Nicotiana tabacum) (Hamid Badawi et al. 2004 ), wheat (Triticum aestivum) (Xiong et al. 2023), Populus (Populus alba × Populus glandulosa) (Cheng et al. 2019), and apple (Malus domestica) (Jia et al. 2019; Yu et al. 2022). Overexpression of genes encoding antioxidant enzymes can significantly increase the activity of antioxidant enzymes and thus enhance plant abiotic stress tolerance. For example, the positive role of SOD-encoding genes in abiotic stress response has been identified in several plant species such as Arabidopsis (Wang et al. 2016a, 2016b; Zhou et al. 2018), tobacco (Hamid Badawi et al. 2004; Faize et al. 2011; Negi et al. 2015), and wheat (Wang et al. 2016a, 2016b).
In response to drought stress, the expression of a variety of TFs, such as MYB (v-myb avian myeloblastosis viral oncogene homolog), bHLH (basic helix-loop-helix), AP2/ERF (APETALA2/ethylene-responsive factor), WRKY, and bZIP (basic leucine zipper), is rapidly induced to propagate signaling cascades via protein interactions and the transcriptional regulation of target genes (Chen et al. 2012; Lindemose et al. 2013; Zhu 2016). The bHLH family is one of the largest TF families in plants, and it is involved in regulating almost all aspects of plant growth and development as well as biotic and abiotic stress responses (Feller et al. 2011; Li et al. 2022a; Liang et al. 2023; Zhan et al. 2023; Zhang et al. 2023; Liu et al. 2023b). A variety of bHLH TFs involved in plant drought responses have been identified. Under drought conditions, bHLH TFs are activated and bind to the specific E-box (CANNTG) in the promoter of target genes, which regulates plant drought responses by regulating the expression of these genes. For example, the bHLH TF AtMYC2 (myelocytomatosis protein 2) promotes the transcription of the drought-responsive gene RD22 (responsive to dehydration 22) by binding to its promoter, which enhances the drought tolerance of Arabidopsis (Abe et al. 2003). ZmbHLH124 directly binds to the ZmDREB2A promoter and activates its transcription to enhance drought tolerance in maize (Zea mays) (Wei et al. 2021). To date, only a few bHLH TFs that regulate drought tolerance have been identified in fruit crops, including VvICE1 and VvbHLH1 in grape (Vitis vinifera) (Li et al. 2014; Wang et al. 2016a, 2016b), PtrbHLH66 in trifoliate orange (Poncirus trifoliata) (Liang et al. 2022), FvICE1 in strawberry (Fragaria vesca) (Han et al. 2023), and MdbHLH130, MdCIB1, MdSAT1, and MdPIF3 in apple (Zhao et al. 2020a; Ren et al. 2021; Zheng et al. 2021; Yang et al. 2021a), and the mechanisms underlying the drought-response mediated by these TFs remain unknown.
Dehydration-responsive element binding (DREB) proteins are members of the AP2/ERF superfamily (Han et al. 2022; Xiao et al. 2023). DREBs play important roles in regulating abiotic stress responses in plants, such as cold, drought, and salt (Cheng et al. 2023; Guo et al. 2023; Qian et al. 2023). They specifically bind to DRE cis-acting elements in the promoters of various downstream stress-response genes and regulate their expression, thereby modulating abiotic stress tolerance (Baker et al. 1994; Rehman and Mahmood 2015). In Arabidopsis, DREB family members are divided into 6 groups: A-1 to A-6. The DREB1/CBF genes in the A-1 group are mainly involved in the response to low-temperature stress, and DREB2 genes in the A-2 group are mainly involved in the regulation of drought and salt stress responses (Sakuma et al. 2002; Nakano et al. 2006; Tavakol et al. 2014). DREB2s from many plant species have been shown to play positive roles in drought responses, such as lettuce (Lactuca sativa), barley (Hordeum vulgare), maize, mungbean (Vigna radiata), and apple (Xue and Loveridge 2004; Qin et al. 2007; Kudo et al. 2014; Li et al. 2019; Vu et al. 2021; Filyushin et al. 2022; Chen et al. 2022a; Li et al. 2022b; Li et al. 2023). For example, AtDREB2A enhances drought tolerance by regulating a diverse set of genes, including COR15A/B, RD17/29A, and KIN1 (Sakuma et al. 2006a; Sakuma et al. 2006b). In apple, MdDREB2A and MsDREB2C were identified as positive regulators of drought tolerance (Zhao et al. 2013; Li et al. 2019; Lian et al. 2021; Li et al. 2022b). Several other DREB TFs from apple that positively regulate drought tolerance have also been identified, such as MsDREB6.2 and MdDREB76 (Liao et al. 2017; Sharma et al. 2019). The expression of these MdDREB genes is significantly increased in response to drought stress (Zhao et al. 2013; Liao et al. 2017; Li et al. 2019; Li et al. 2022b). However, the mechanism by which drought activates their expression remains unclear.
Ubiquitination is a post-translational modification of proteins that is required for numerous cellular processes, including protein degradation, transcriptional regulation, and signal transduction (Hua and Vierstra 2011; Morreale and Walden 2016). The ubiquitin-proteasome system (UPS) has a substantial effect on the survival of sessile plants under different types of abiotic stress, such as salinity, cold, and drought, by mediating the ubiquitination of target proteins (Song et al. 2017; Sun et al. 2020). The UPS consists of many parts: ubiquitin (Ub), ubiquitin-activating enzyme (E1), ubiquitin-conjugating enzyme (E2), ubiquitin-protein ligase (E3), 26S proteasome, and target proteins (Zheng and Shabek 2017). There are 3 types of E3 ligase: RING E3s, HECT E3s, and RBR E3s. The cullin-RING ligase (CRL) complex is assembled on a cullin scaffold, binding a RING-box protein at its N-terminus and an adaptor protein and a substrate receptor (responsible for substrate specificity) at its C-terminus (Morreale and Walden 2016). In plants, there are 3 types of cullin proteins: CUL1/2, CUL3a/b, and CUL4 (Petroski and Deshaies 2005; Hotton and Callis 2008; Hua and Vierstra 2011). BT2 is a BTB protein that exhibits ubiquitination activity by forming E3 ubiquitin ligase complexes with the cullin scaffolding protein CUL3 and the RING finger protein RBX1 (Figueroa et al. 2005; Ren et al. 2007). It participates in many biological processes in plants, such as nutrients, abiotic stress responses, and hormone signaling (Mandadi et al. 2009). In apple, MdBT2 is involved in regulating multiple aspects of growth and development, such as leaf senescence (An et al. 2019), Fe homeostasis (Zhao et al. 2016a), anthocyanin accumulation (An et al. 2018a; An et al. 202 °c), and vacuolar acidification (Zhang et al. 2020), by mediating the ubiquitination and degradation of various TFs. MdBT2 negatively regulates the low-temperature and drought tolerance of apple by promoting the ubiquitination and degradation of MdMYB23 (An et al. 2018a) and MdNAC143 (An et al. 2020c; Ji et al. 2020), respectively. However, whether MdBT2 regulates apple drought tolerance through its effects on bHLH proteins remains unclear.
Apple is one of the most widely cultivated and economically important fruit trees worldwide. Drought stress adversely affects the growth, quality, and yield of apples (Liao et al. 2017; Wang et al. 2020). Due to the long juvenile phase of apple trees, biotechnology is an effective approach for improving the drought tolerance of apple. A thorough understanding of the responses of apple to drought stress at the molecular level is necessary for the development of drought-tolerant cultivars (Chen et al. 2022b). Here, we identified the ABA-response gene MdbHLH160 from apple and demonstrated that it plays a positive role in drought tolerance in Arabidopsis and apple. Promoter binding and protein interaction analyses indicated that MdbHLH160 promoted SOD enzyme activity under drought by directly promoting MdSOD1 expression and interacting with MdSOD1 protein. MdbHLH160 was also shown to directly promote the expression of MdDREB2A-like, a DREB family gene that positively modulates apple drought tolerance. In addition, MdBT2 was identified as a MdbHLH160-interacting protein. It promoted the ubiquitination and degradation of MdbHLH160, and ABA treatment substantially inhibited this process. Our research provides a mechanistic understanding of ABA-mediated drought tolerance at both the transcriptional and post-translational levels.
Results
Gene cloning and characterization of MdbHLH160
The full-length coding sequence (CDS) of MdbHLH160 (MD10G1098900) is 801 bp. The encoded protein is 266 amino acids (aa) and contains a conserved bHLH domain (Supplemental Fig. S1). Tissue-specific expression analysis showed that MdbHLH160 is highly expressed in the leaves and roots and lowest in fruits (Fig. 1A). MdbHLH160 expression in the roots and leaves was significantly induced by polyethylene glycol (PEG) and ABA treatment (Fig. 1, B and C), suggesting that MdbHLH160 might play a role in drought response. Subcellular localization analysis in Nicotiana benthamiana leaves showed that MdbHLH160 was localized to the nucleus (Fig. 1D). MdbHLH160 showed strong transcriptional activation activity in yeast, and this activity disappeared when 30 aa at its N-terminal was truncated, indicating that the N-terminal was the transcriptional activation domain of MdbHLH160 (Fig. 1E). These results suggest that MdbHLH160 is a typical bHLH TF gene that responds to drought and ABA.
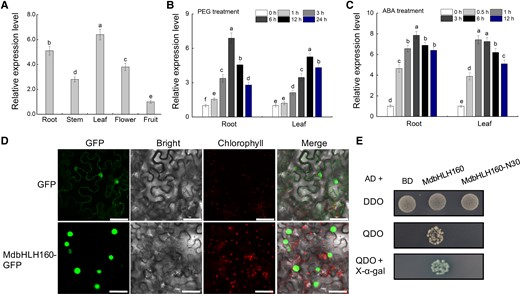
Expression analysis and protein characteristics of MdbHLH160. A) Relative expression levels of MdbHLH160 in different tissues of apple (“Golden delicious’). B to C) Expression analysis of MdbHLH160 in apple roots and leaves under PEG B) and ABA C) treatment. Total RNA was isolated using a Wolact Plant RNA Isolation Kit (Wolact, Hong Kong, China). Quantitative real-time PCR (RT-qPCR) was performed with a LightCycler 96 quantitative instrument (Roche, Basel, Switzerland). MdMDH (Malate dehydrogenase) was used as an internal reference gene (Niu et al. 2022; Chen et al. 2022b; Li et al. 2022b). The primer sequences used for RT-qPCR analysis are listed in Supplemental Table S1. Gene expression levels were calculated by the 2−ΔΔCT method. Error bars indicate the SD of 3 biological replicates. Different letters represent significant differences based on 1-way ANOVA and Duncan's test (P < 0.05). PEG, polyethylene glycol; ABA, abscisic acid. D) Subcellular localization of MdbHLH160-GFP in N. benthamiana leaves. Bars = 50 μm. E) Identification of the self-activation activity of full-length and truncated MdbHLH160 (MdbHLH160-N30) based on Y2H assays. DDO, SD medium lacking Trp and Leu; QDO, SD medium lacking Trp, Leu, His, and Ade.
Analysis of the function of MdbHLH160 in the drought response in Arabidopsis
To identify the role of MdbHLH160 in the drought response, various transgenic Arabidopsis lines were obtained (Supplemental Fig. S2A). Three lines (#1, #2, #3) with high MdbHLH160 expression (Supplemental Fig. S2B) were selected for subsequent mannitol and drought treatments. Arabidopsis seedlings were placed vertically on 1/2 MS medium supplemented with 0, 200, and 300 mM mannitol and cultured for 6 d under long-day (LD) conditions. In 1/2 MS medium, there was no noticeable difference in the growth and root length between wild type (WT; “Col”) and transgenic seedlings. Under mannitol treatment, the leaf yellowing of transgenic lines was less pronounced than that of the WT, and the roots of transgenic lines were significantly longer than the roots of the WT (Fig. 2, A and B), suggesting that MdbHLH160 expression alleviated the damage and growth inhibition of Arabidopsis caused by mannitol. Moreover, MdbHLH160-OE lines had significantly more lateral roots than WT plants under mannitol treatment (Fig. 2, A and C). The ROS fluorescent probe C-H2DCFDA was used to monitor ROS accumulation in the root tips. Under mannitol treatment, the ROS accumulation was significantly lower in OE lines than in the WT (Fig. 2, D and E).
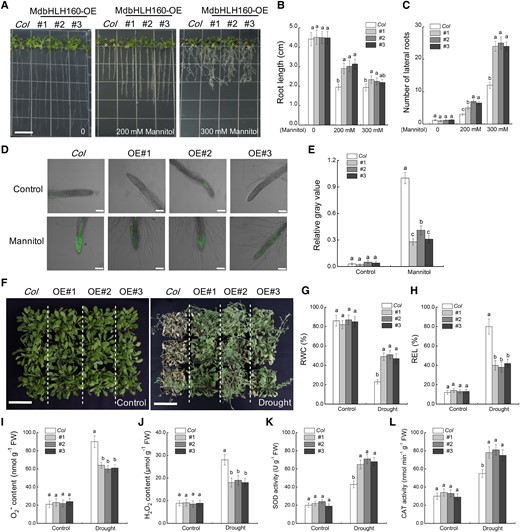
Ectopic expression of MdbHLH160 in Arabidopsis increased drought tolerance. A) Representative images of Arabidopsis seedlings. “Col” and MdbHLH160 transgenic seedlings were cultivated on 1/2 MS medium supplemented with 0, 200, or 300 mM of mannitol for 6 d. Bars = 1 cm. B) Primary root length. C) Number of lateral roots. The root images of Arabidopsis were obtained using a SNAPSCAN 310 scanner and analyzed by WinRHIZO analysis software (V4.1c; Regent Instruments, Quebec City, QC, Canada) to obtain lateral root numbers. At least 15 roots of each line were counted in C. D to E) Fluorescence observation (D) and quantification (E) of ROS accumulation in root tips. Root tips treated with 300 mM mannitol were incubated with the ROS-sensitive fluorescent probe C-H2DCFDH. Scale bars, 100 µm. The relative gray value of at least 15 roots of each line under 300 mM mannitol treatment was counted by ImageJ software. F) Representative images of Arabidopsis plants after 10 d of drought treatment. A total of 24 plants from each line (6-wk-old) were included in a biological replicate, and there were 3 replicates per treatment. Bars = 7 cm. (G to L) RWC G) REL H) O2− content I) H2O2 content J) SOD activity K), and CAT activity L) in the leaves of “Col” and transgenic lines before (control) and after drought treatment. The data are presented as means ± SD of 3 replicates. Bars labeled with different letters indicate values are significantly different at P < 0.05, based on 1-way ANOVA and Duncan's tests.
To investigate the function of MdbHLH160 in drought tolerance, 6-wk-old adult Arabidopsis plants grown in soil were treated with drought stress. Under normal conditions, there was no noticeable morphological difference between lines. After 10 days of drought treatment, phenotypic comparison and relative water content (RWC) and relative electrolyte leakage (REL) determination showed that the MdbHLH160-OE lines experienced less stress-related damage than WT plants (Fig. 2, F–H). Under drought, the ROS (O2− and H2O2) accumulation was lower in the leaves of MdbHLH160-OE lines than in WT leaves, while the activities of the antioxidant enzymes SOD and CAT were higher in MdbHLH60 lines than in WT plants (Fig. 2, I–L), demonstrating that MdbHLH160 may inhibit excessive ROS accumulation by promoting antioxidant enzyme activity. These results indicate that MdbHLH160 positively regulates the drought response in Arabidopsis.
MdbHLH160 overexpression enhances drought tolerance in apple
To characterize the function of MdbHLH160 in apple, MdbHLH160 overexpression (MdbHLH160-HA-GFP[OE]) and RNA-interference (MdbHLH160-RNAi) vectors were constructed and transformed into apple calli, respectively. Positive transgenic lines with MdbHLH160 overexpression or inhibited MdbHLH160 expression was verified by RT-qPCR and western blot (WB) analyses (Supplemental Fig. S2, C and D). WT and MdbHLH160 transgenic calli with consistent growth were used for PEG treatment. After 20 d of culture, the OE lines showed better growth and had a higher fresh weight than WT calli under PEG treatment; the opposite phenotype was observed in the RNAi lines (Fig. 3, A and B). Measurements of antioxidant enzyme activities showed that MdbHLH160 overexpression promoted the activity of SOD and CAT enzymes under PEG treatment (Fig. 3, C and D).
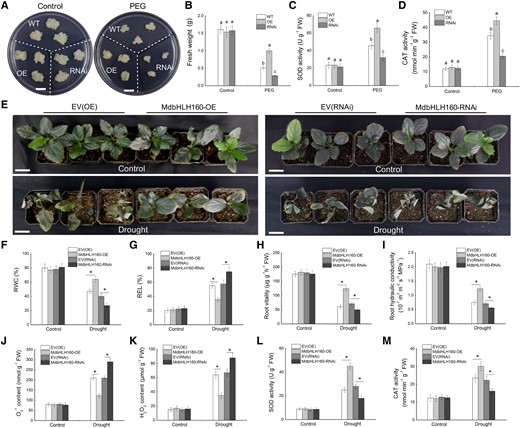
Phenotypic analysis of transgenic apple material under drought treatment. A) Representative images of calli. Bars = 1 cm. B) Fresh weight. C) SOD activity. D) CAT activity. WT, MdbHLH160-OE/RNAi transgenic calli were cultured on MS medium supplemented with 0 or 10% PEG6000 for 20 d. E) Growth phenotypes of transgenic apple plants after 20 d of drought treatment. Three replicates of 45 plants of each genotype were used for drought treatment. Scale bars represent 3 cm. F-G) RWC F) and REL G) in the leaves of apple plants. (H to M) Root vitality H), root hydraulic conductivity I), O2− content J), H2O2 content K), SOD activity L), and CAT activity M) in transgenic roots of apple plants. Samples were collected after 12 d of drought treatment to measure physiological parameters. Samples collected before drought treatment were used as controls. The data are presented as the means ± SD of 3 replicates. Different letters in B–D indicate significant differences based on 1-way ANOVA and Duncan's tests. Bars labeled with * in each panel are significantly different from the corresponding control plants (EV-OE/EV-RNAi) at P < 0.05 (Student's t-test).
The use of A. rhizogenes to obtain plants with transgenic roots facilitates the rapid study of gene functions in apples. To identify the role of MdbHLH160 in regulating apple drought tolerance, the MdbHLH160-OE and MdbHLH160-RNAi vectors were transformed into apple roots to obtain composite plants. After confirmation of the transgene by fluorescence observation and RT-qPCR analysis (Supplemental Fig. S3), 45 plants with high MdbHLH160 expression and 45 plants with significantly inhibited MdbHLH160 expression were selected for drought treatment. Plants transformed with the empty vectors (EV-OE and EV-RNAi) were used as controls. Under normal conditions, there were no significant differences among different lines. After 20 d of drought treatment, the OE lines were less damaged than the EV(OE) lines, and the RNAi lines were more severely damaged than the EV(RNAi) lines (Fig. 3E). Measurements of RWC and REL in leaves were consistent with the phenotypic differences (Fig. 3, F and G). These results suggest that MdbHLH160 overexpression in the roots enhances the drought tolerance of apple plants.
Root vitality and root hydraulic conductivity were measured to determine the effect of MdbHLH160 expression on the root system. Before the drought treatment, no difference was observed among the genotypes. Under drought treatment, root vitality, and root hydraulic conductivity were significantly higher in MdbHLH160-OE lines than in EV(OE) plants, and that in MdbHLH160-RNAi lines were significantly lower than in EV(RNAi) plants (Fig. 3, H and I), demonstrating that MdbHLH160 overexpression helps maintain the water absorption capacity of the roots under drought stress. Furthermore, the high ROS accumulation and low activity of SOD and CAT enzymes in the roots of control plants compared with MdbHLH160-OE lines suggested that MdbHLH160 might alleviate oxidative stress damage caused by drought by promoting the scavenging of excess ROS (Fig. 3, J–M).
MdbHLH160 interacts with MdSOD and promotes MdSOD activity
To explore the mechanism underlying MdbHLH160-mediated drought tolerance, MdbHLH160-N30 was used as a query to screen the apple cDNA library by a yeast 2-hybrid (Y2H) system, and MdSOD1 (MD01G1164700) was identified as a potential MdbHLH160-interacting protein. The CDS of MdSOD1 was then cloned, and the MdbHLH160–MdSOD1 interaction was confirmed through a Y2H assay (Fig. 4A). In addition, the MdbHLH160-HIS and MdSOD1-GST proteins were expressed and purified (Supplemental Fig. S4), and this interaction in vitro was further verified by pull-down assays (Fig. 4B). The MdbHLH160–MdSOD1 interaction was also identified in vivo by split-luciferase (LUC) and Co-IP assays (Fig. 4, C and D).
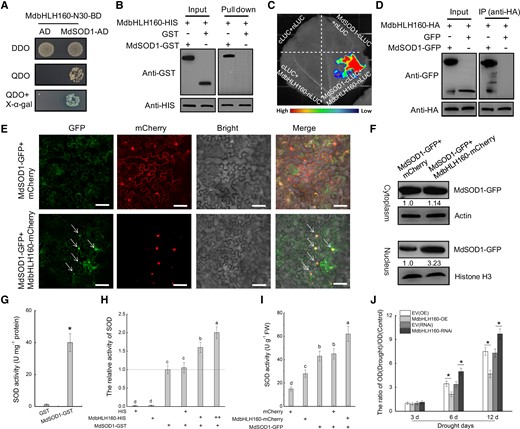
MdbHLH160 promotes the nuclear localization and activity of MdSOD1 through protein interactions. (A to D) Identification of protein interactions between MdbHLH160 and MdSOD1 through Y2H A), pull-down B) split-LUC C), and Co-IP D) assays. E) Co-localization of MdbHLH160 and MdSOD1 proteins. MdSOD1-GFP and MdbHLH160-mCherry proteins were co-expressed in N. benthamiana leaves. mCherry was used as a control. No less than 10 fields were observed in each combination. Scale bars, 75 µm. F) Immunoblot analysis of MdSOD1-GFP. The Cell Lysis and Recombinant Protein Extraction and Organelle Isolation kits (Sigma, Darmstadt, Germany) were used to isolate and collect cytosolic and nuclear fractions. Anti-actin and anti-Histone H3 were used as cytosolic and nuclear loading controls, respectively. Gray values of the bands of the MdSOD1-GFP protein in the control combination (MdSOD1-GFP + mCherry) were set to 1.00. Values are shown below the strip. G) SOD activity of purified GST and MdSOD1-GST proteins. H) Effects of MdbHLH160-HIS on MdSOD1 activity in vitro. Purified HIS protein was used as a control. “+” and “++” represent the ratio of the protein. I) Effects of MdbHLH160 co-expression on SOD activity in MdSOD1 transgenic N. benthamiana leaves. No less than 9 leaves are used. J) The ROS level in the root cell nucleus was determined using cytochrome c oxidation assays. The root tip tissue of composite apple plants was cut down, and the nucleus was separated. ROS in the root cell nucleus was measured by dropping nuclear lysate into the cytochrome c standard mother liquor (1 mg ml−1; Solarbio). OD values were measured at 520 nm with a spectrophotometer in 3 separate experiments according to a previously described method (Chen et al. 2021b). Six biological replicates and 3 technical replicates were performed for each type of sample. The data are presented as the means ± SD. Different letters in (H) and (I) indicate significant differences at P < 0.05 based on 1-way ANOVA and Duncan's tests. * in each panel indicates significantly different from the control at P < 0.05 according to a Student's t-test.
Since MdbHLH160 is located in the nucleus (Fig. 1D), how the MdbHLH160–MdSOD1 interaction affects the function of MdSOD1 merits investigation. We identified the effect of MdbHLH160 expression on the subcellular localization of MdSOD1 in Nicotiana benthamiana leaves. Fluorescence observation showed that the MdSOD-GFP protein was widely distributed in all parts of the cell. After co-expression of MdbHLH160, MdSOD-GFP accumulation in the nucleus increased (Fig. 4E). To confirm this observation, cytoplasmic and nuclear proteins were extracted and tested using anti-GFP antibody. No detectable change was observed in cytoplasm-localized MdSOD1-GFP protein. However, the accumulation of MdSOD1-GFP protein in the nucleus increased substantially after the co-expression of MdbHLH160 (Fig. 4F). These findings suggest that MdbHLH160 might promote the nuclear localization of MdSOD1 protein via protein interactions.
To clarify the effect of the MdbHLH160–MdSOD1 interaction on MdSOD1 activity, the catalytic activity of the purified MdSOD1-GST protein was measured in vitro. The activity of MdSOD1-GST was high compared with the GST control (Fig. 4G). The addition of MdbHLH160-HIS can significantly promote MdSOD1 activity, whereas HIS did not (Fig. 4H). In addition, measurements of SOD activity in Nicotiana benthamiana leaves showed that leaves expressing MdSOD1-GFP + MdbHLH160-mCherry had significantly higher SOD activity than leaves expressing MdSOD1-GFP or MdbHLH160-mCherry alone (Fig. 4I). These results suggest that the MdbHLH160–MdSOD1 interaction promoted MdSOD1 activity.
Next, the nucleus of transgenic root cells of the drought-treated apple plants was extracted, and the ROS dye (cytochrome c, as an oxidation indicator) was used to detect ROS accumulation in the nucleus. The MdbHLH160-OE lines accumulated less ROS and the MdbHLH160-RNAi lines accumulated more ROS compared with control lines after drought treatment (Fig. 4J). These results indicated that MdbHLH160 inhibited excessive ROS accumulation in the nucleus by promoting the nuclear localization and activity of MdSOD1 protein through protein interactions.
MdbHLH160 directly activates MdSOD1 expression
Studies have shown that the bHLH TFs positively regulate plant tolerance to abiotic stress by promoting the expression of antioxidant enzyme-encoding genes (Geng and Liu 2018). We investigated whether MdbHLH160 affects MdSOD1 expression in apple. MdSOD1 expression was significantly increased in MdbHLH160-OE lines but decreased in MdbHLH160-RNAi lines under stress treatments (Fig. 5, A and B). Moreover, MdbHLH160 overexpression significantly promoted MdSOD1 expression under nonstress conditions (Fig. 5, A and B). These results indicate that MdbHLH160 positively regulated MdSOD1 expression. To further verify this result, the promoter sequence of MdSOD1 was cloned, and dual-LUC assays were performed. Fluorescence observation and LUC activity determination showed that MdbHLH160 significantly enhanced the transcriptional activity of the MdSOD1 promoter (Fig. 5C).
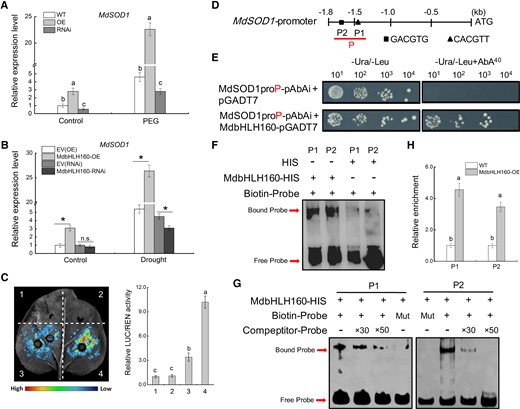
MdbHLH160 directly activates MdSOD1 transcription. A) The expression of MdSOD1 in MdbHLH160 transgenic calli. B)MdSOD1 expression in the MdbHLH160 transgenic roots of apple plants. Samples were collected from stress-treated apple calli and plants in Fig. 3. C) Dual-LUC assays show that MdbHLH160 activates the transcription of the MdSOD1 promoter. Representative images are shown. The combinations are as follows: (i), empty reporter and effector; (ii) empty reporter + 35S::MdbHLH160; (iii) MdSOD1pro::LUC + empty effector; and (iv) MdSOD1pro::LUC + 35S::MdbHLH160. D) Diagram of potential MdbHLH160-binding sites in the MdSOD1 promoter. E) Y1H assays showing the interaction between MdbHLH160 and MdSOD1 promoter (MdSOD1proP-pAbAi). The yeast strains were grown on SD/-Ura/-Leu and SD/-Ura/-Leu + 40 ng mL−1 Aureobasidin A (AbA) media for 3 d. F to G) EMSAs showing the binding of MdbHLH160 to the MdSOD1 promoter in vitro. The HIS protein served as a negative control. The unlabeled probes were used as competitors. × 30 and ×50 represent the rates of the competitor. Mut, mutated probe in which the 5′-GACGTG-3′ and 5′-CACGTT-3′ motifs were replaced by 5′-AAAAAA-3′. H) ChIP-qPCR assays showing the binding of MdbHLH160 to the MdSOD1 promoter in vivo. WT and MdbHLH160-HA transgenic calli were treated with 8% PEG6000 treatment for 10 h in the dark. Chromatin was immunoprecipitated using anti-HA antibody, and qPCR was performed using the primers listed in Supplemental Table S1. WT was used as a control. The value of WT calli was set to 1.0. Error bars indicate the SD of 3 biological replicates. Different letters in A, C, and H indicate significant differences at P < 0.05 based on 1-way ANOVA and Duncan's tests. * in B in each panel indicates significant differences from the control lines at P < 0.05 according to Student's t-test.
Sequence analysis showed that there were 2 putative bHLH TF-binding sites (P1, P2) in the MdSOD1 promoter (Fig. 5D). To identify whether MdbHLH160 directly binds to the MdSOD1 promoter, a fragment containing these 2 sites (MdSOD1pro-P) was cloned for a Y1H assay. Yeast transformed with MdbHLH160-AD and MdSOD1pro-pAbAi grew normally on media supplemented with Aureobasidin A (AbA), suggesting that MdbHLH160 binds to the MdSOD1 promoter (Fig. 5E). Electromobility shift assays (EMSAs) were conducted to verify this binding. MdbHLH160 bound to both the P1 and P2 sites in the MdSOD1 promoter (Fig. 5F). The specificity of the binding was verified by competitive EMSAs with the addition of mutant and competing probes (Fig. 5G). To identify this binding in vivo, the PEG-treated MdbHLH160-OE(HA) transgenic calli were collected for ChIP-qPCR analysis. The relative enrichment was significantly higher in transgenic calli than in WT plants, indicating that MdbHLH160 binds directly to the MdSOD1 promoter in apples (Fig. 5H). The above results revealed that MdbHLH160 activated MdSOD1 expression by directly binding to the MdSOD1 promoter, which in turn promoted SOD activity.
MdbHLH160 directly activates MdDREB2A-like expression
Many studies have shown that bHLH TFs modulate plant stress tolerance by directly regulating the expression of DREB family genes, and A-2 subgroup (DREB2) genes are key members regulating the plant drought response (Liu et al. 1998; Li et al. 2019). We speculated whether DREB2 genes were involved in MdbLHL160-mediated drought tolerance in apple. Ten MdDREB2 genes were identified in the apple genome (Malus × domestica GDDH13 v1.1) through genome-wide screening and phylogenetic analysis (Supplemental Fig. S5). Expression analysis in PEG-treated calli showed that the expression of most MdDREB2 genes was significantly upregulated in MdbLHL160-OE calli, and the expression of some of these genes was down-regulated in MdbLHL160-RNAi calli (Fig. 6A), suggesting that MdbHLH160 might modulate apple drought tolerance by regulating the expression of MdDREB2 genes.
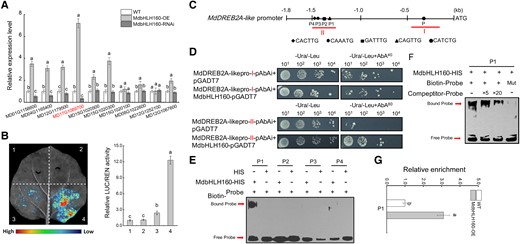
MdbHLH160 directly promotes MdDREB2A-like expression. A) The relative expression level of MdDREB2 genes in WT and MdbHLH160 transgenic calli under PEG treatment. Samples were collected from PEG-treated apple calli in Fig. 3. Primers used for RT-qPCR analyses are listed in Supplemental Table S1. The gene MD17G1089700 (MdDREB2A-like) showed the greatest variation in expression between genotypes. B) Dual-LUC assays show that MdbHLH160 activates the transcriptional activity of the MdDREB2A-like promoter. The combinations are as follows: (i) empty reporter and effector; (ii) empty reporter + 35S::MdbHLH160; (iii) MdDREB2A-likepro::LUC + empty effector; and (iv) MdDREB2A-likepro::LUC + 35S::MdbHLH160. C) Diagram of potential MdbHLH160-binding sites in the MdDREB2A-like promoter. D) Y1H assays show the interaction between MdbHLH160 and the MdDREB2A-like promoter. Red letters (I, II) indicate the fragments marked with red lines in C. E-F) EMSAs showing that MdbHLH160 binds to the P1 site in the MdDREB2A-like promoter. HIS protein served as a negative control. Mut, mutated probe in which the 5′-CAGTTG-3′ motif was replaced with 5′-AAGTAA-3′. G) ChIP-qPCR assays showing that MdbHLH160 binds to the MdDREB2A-like promoter at the P1 site in vivo. The ChIP samples of apple calli in Fig. 5H were used for qPCR analyses. Error bars indicate the SD of 3 biological replicates. Different letters represent significant differences based on 1-way ANOVA and Duncan's tests (P < 0.05).
The gene MD17G1089700 showed the greatest variation in expression between genotypes and was selected for further study. It was named MdDREB2A-like based on its ortholog in Arabidopsis, and its promoter and CDSs were cloned. Results of dual-LUC assays showed that MdbLHL160 promoted the transcriptional activity of MdDREB2A-like promoter (Fig. 6B). Sequence analysis identified 5 potential MdbHLH160-binding motifs in the MdDREB2A-like promoter (P, P1-P4). According to the aggregation characteristics of motifs, 2 segments (I, II) were cloned into pAbAi vectors for promoter binding identification through a Y1H assay (Fig. 6C). The results showed that MdbLHL160 bound to segment II in yeast (Fig. 6D). EMSAs were then performed to determine which site was the MdbHLH160-binding site in this region, and the results showed that MdbLHL160 bound to the P1 site (Fig. 6E). The binding specificity was verified through competitive EMSAs by adding competitive and mutant probes (Fig. 6F). In addition, the binding of MdbHLH160 to the MdDREB2A-like promoter was identified in vivo through Chip-qPCR assays using MdbHLH160-OE calli (Fig. 6G). These results indicate that MdbHLH160 directly promotes MdDREB2A-like expression by binding to its promoter.
MdDREB2A-like positively modulates apple drought tolerance
Several apple DREB proteins are involved in the modulation of drought tolerance (Liao et al. 2017; Li et al. 2019; Sharma et al. 2019; Li et al. 2022b). To identify the role of MdDREB2A-like in the apple drought response, transgenic apple calli overexpressing MdDERB2A-like or with MdDERB2A-like expression inhibited were obtained (Supplemental Fig. S6, A and B) and subjected to PEG treatment (Fig. 7A). The overexpression of MdDREB2A-like enhanced growth, increased the fresh weight, and decreased the MDA content in transgenic calli compared with WT, and the opposite pattern was observed in MdDREB2A-like-RNAi calli (Fig. 7, A to C). Furthermore, composite apple plants with transgenic roots were obtained (Supplemental Fig. S6, C and D) and subjected to drought for 20 d. Under drought treatment, the MdDREB2A-like-OE lines showed better growth, higher RWC, and a lower REL and MDA content compared with control plants (Fig. 7, D–G), indicating that the MdDREB2A-like-OE lines experienced less damage caused by drought. The opposite patterns were observed for MdDREB2A-like-RNAi lines. In addition, the root vitality and hydraulic conductivity were higher in MdDREB2A-like-OE lines than in control plants (Fig. 7, H and I). These results indicate that MdDREB2A-like positively regulates apple drought tolerance.
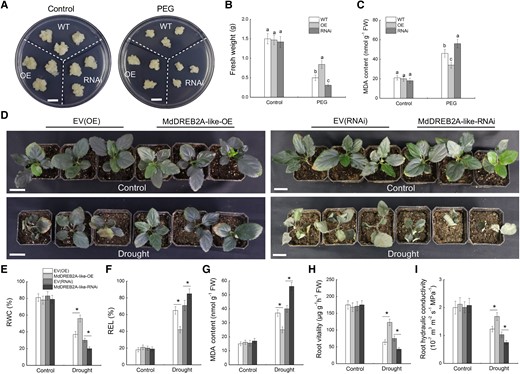
Overexpression of MdDREB2A-like enhances drought stress tolerance in apple. (A to C) Growth phenotypes A), fresh weight B), and MDA content C) of WT and MdDREB2A-like transgenic calli treated with PEG6000. Bars = 1 cm. Error bars represent the means ± SD from 3 independent experiments. Different letters represent significant differences (ANOVA, Duncan's tests, P < 0.05). D) Phenotypic comparison of transgenic apple plants after 20 d of drought treatment. Three replicates of 45 plants of each genotype were subjected to drought treatment. Scale bars represent 3 cm. (E-G) RWC E), REL F), and MDA content G) in apple leaves. (H-I) Root vitality H) and root hydraulic conductivity I) of different transgenic lines. Samples were collected after 12 d of drought treatment for measurements of physiological parameters. The data are presented as the means ± SD of 3 replicates. * in each panel indicates significant differences from the corresponding control plants (EV-OE/EV-RNAi) at P < 0.05 according to Student's t-test.
Drought and ABA treatment promote the stability of MdbHLH160
ABA plays a key role in plant drought stress responses by affecting gene expression and the stability of drought response TFs (Chen et al. 2021a). Since MdbHLH160 expression is strongly induced by PEG and ABA treatment (Fig. 1, B and C), the effect of these treatments on MdbHLH160 protein stability was investigated using MdbHLH160-HA transgenic calli. Under control conditions, MdbHLH160 degraded rapidly. By contrast, both PEG and ABA treatments inhibited the degradation of MdbHLH160, and the inhibitory effect of ABA was more noticeable compared with that of PEG (Fig. 8, A and B). These results indicate that drought and ABA promoted MdbHLH160 stability.
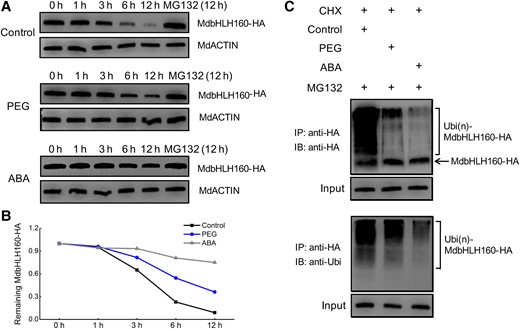
Drought and ABA treatment inhibit the ubiquitination and degradation of MdbHLH160. A) MdbHLH160 degradation was inhibited in response to PEG and ABA treatment. MdbHLH160-HA transgenic calli were treated with PEG and ABA. Samples of calli were taken at specified time points, and total proteins were extracted and then used for immunoblotting assays using the anti-HA antibody. MdActin was used as a loading control. B) Degradation curve analysis of MdbHLH160-HA using the band intensity (gray value) in (A). Band intensity was determined using ImageJ software. C) Ubiquitination assays of MdbHLH160. The protein extracts of the calli (treated with PEG or ABA for 6 h, +MG132) were used for immunoprecipitation using anti-HA magnetic beads. Immunoprecipitated samples were then detected using anti-HA (top) and anti-Ubi (bottom) antibodies.
The 26S proteasome inhibitor MG132 inhibited the degradation of MdbHLH160, suggesting that the ubiquitin-26S proteasome pathway was involved in the degradation of MdbHLH160 (Fig. 8, A and B). We thus tested and compared the ubiquitination of MdbHLH160 under different treatment conditions. The ubiquitination of MdbHLH160 was substantially reduced under PEG and ABA treatment compared with the control, and the ubiquitination level was lowest in ABA-treated calli (Fig. 8C). These results suggest that drought and ABA treatment inhibited the ubiquitination and degradation of MdbHLH160.
MdbHLH160 interacts with MdBT2
In addition to MdSOD1, MdBT2 was identified as a potential MdbHLH160-interacting protein based on Y2H screening. The CDS of MdBT2 was thus cloned and fused into the BD vector for Y2H assays; the results showed that MdbHLH160 interacted with MdBT2 in yeast (Fig. 9A). To further clarify the interaction, the MdBT2-GST fusion protein was expressed and purified. Results of pull-down assays showed that MdbHLH160 could interact with MdBT2 in vitro (Fig. 9B). Next, split-LUC assays were carried out to demonstrate the interaction in vivo. Fluorescence was only observed when MdbHLH160-nLUC and MdBT2-cLUC were co-expressed, indicating that these 2 proteins interacted (Fig. 9C). In addition, the Co-IP assay was performed. After immunoprecipitation with anti-HA antibody, the MdBT2-Flag protein could be detected when co-expressed with MdbHLH160-HA, but GFP-Flag could not be detected, indicating that MdbHLH160 interacted with MdBT2 in vivo (Fig. 9D).
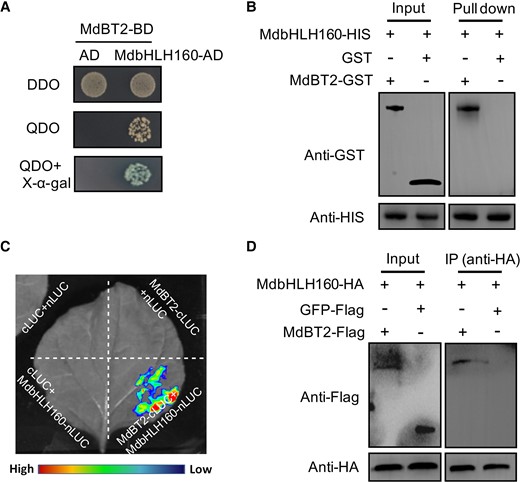
MdbHLH160 interacts with MdBT2. A) Y2H assays reveal an interaction between MdbHLH160 and MdBT2. B) Pull-down assays revealing the interaction between MdbHLH160 and MdBT2 in vitro. The GST protein was used as the control. C) Identification of the MdbHLH160-MdBT2 interaction through split-LUC assays. Combinations containing empty nLUC or cLUC vectors were used as controls. D) Co-IP assays indicate that MdbHLH160 interacts with MdBT2 in vivo. MdBT2-Flag and MdbHLH160-HA proteins were co-expressed in Nicotiana benthamiana leaves. Total protein extracts were immunoprecipitated with an anti-HA antibody and immunoblotted with an anti-Flag antibody. The GFP-Flag protein was used as a negative control.
ABA inhibits the MdBT2-mediated ubiquitination and degradation of MdbHLH160
MdBT2 is involved in plant response to abiotic stress by mediating the ubiquitination and degradation of several TFs (An et al. 2018a; An et al. 2020c; Ji et al. 2020). Here, a cell-free method was used to examine whether MdBT2 affects the degradation of MdbHLH160. Two transgenic calli with MdBT2 expression overexpressed (MdBT2-Flag [OE]) or interfered (MdBT2-RNAi) were obtained (Supplemental Fig. S7). Total proteins were then extracted from WT and transgenic calli and incubated with the purified MdbHLH160-HIS protein for different periods. The MdbHLH160-HIS protein was degraded faster when incubated with extracts of MdBT2-Flag calli than when they were incubated with extracts of WT calli. MdbHLH160-HIS protein incubated with extracts of MdBT2-RNAi calli showed the opposite pattern (Fig. 10A). These results indicate that MdBT2 promoted the degradation of MdbHLH160. To examine the negative role of MdBT2 in MdbHLH160 protein stability in vivo, MdbHLH160-HA + MdBT2-Flag and MdbHLH160-HA + MdBT2-RNAi double transgenic calli were obtained. The degradation of MdbHLH160-HA protein was substantially promoted by MdBT2 overexpression and inhibited by interference with MdBT2 expression (Fig. 10B). MG132 treatments eliminated the effect of MdBT2 on MdbHLH160 protein stability (Fig. 10, A and B), suggesting that MdBT2 promoted MdbHLH160 degradation via the ubiquitin-26S proteasome pathway.
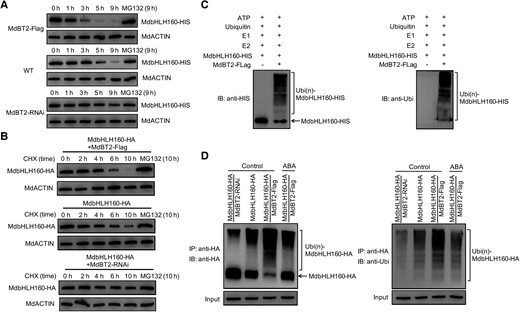
ABA inhibits the MdBT2-mediated ubiquitination and degradation of MdbHLH160. A) Cell-free degradation assays of MdbHLH160-HIS protein. Protein extracts of WT and MdBT2 transgenic calli (MdBT2-Flag, MdBT2-RNAi) were incubated with MdbHLH160-HIS protein for the indicated periods. MdbHLH160-HIS protein levels were detected by immunoblotting using the anti-HIS antibody. For MG132 treatment, MG132 was added to the 3 protein extracts for 0.5 h, followed by incubation with MdbHLH160-HIS protein for 9 h. B) MdBT2 promoted MdbHLH160 degradation in vivo. The protein levels of MdbHLH160-HA in 3 types of transgenic calli were detected at specified time points under control conditions (treated with CHX ± MG132) with the anti-HA antibody. C) Ubiquitination assays in vitro. The MdBT2-Flag active protein was immunoprecipitated from MdBT2-Flag calli using anti-Flag antibody and incubated with MdbHLH160-HIS, E1, E2, and ubi at 30 °C. The mixture was used to conduct immunoblotting assays with anti-HIS (left) and anti-Ubi (right) antibodies. D) MdBT2 promoted MdbHLH160 ubiquitination in vivo. The transgenic calli were treated with or without ABA for 8 h in MS medium supplemented with 250 μM CHX and 100 μM MG132. The protein extracts were immunoprecipitated using anti-HA magnetic beads. The eluted solution was detected with anti-HA (left) and anti-Ubi (right) antibodies.
To examine whether MdBT2 directly promotes the ubiquitination of MdbHLH160, the MdBT2-Flag active protein extracted from MdBT2-Flag calli was co-incubated with MdbHLH160-HIS protein, E1, E2, and ubiquitin in vitro. A greater number of high-molecular-mass forms of MdbHLH160 (poly ubiquitinated MdbHLH160) were detected when supplemented with MdBT2-Flag active protein (Fig. 10C), indicating that MdBT2 is essential for the ubiquitination of MdbHLH160. Subsequently, in vivo ubiquitination assays were conducted using various types of transgenic calli. The abundance of poly ubiquitinated MdbHLH160-HA was greater in MdbHLH160-HA + MdBT2-Flag calli than in MdbHLH160-HA calli but lower in MdbHLH160-HA + MdBT2-RNAi calli (Fig. 10D). Moreover, ABA treatment made the abundance of poly ubiquitinated MdbHLH160-HA in MdbHLH160-HA + MdBT2-Flag calli substantially lower than that under control conditions (Fig. 10D). These results indicate that ABA inhibits MdBT2-mediated MdbHLH160 ubiquitination and degradation.
MdBT2 attenuates MdbHLH160-mediated drought tolerance and the transcriptional activation of downstream target genes
The inhibitory effect of MdBT2 on MdbHLH160 stability suggests that MdBT2 may modulate apple drought responses through MdbHLH160. To test this hypothesis, MdbHLH160 and MdBT2 transgenic calli were exposed to PEG treatment. The growth of MdbHLH160-overexpressing calli was greater than that of WT calli, and MdBT2 overexpression inhibited calli growth under PEG treatment (Fig. 11A). Overexpression of MdBT2 under a MdbHLH160-HA calli background (MdbHLH160-HA + MdBT2-Flag) resulted in a lower fresh weight and higher O2− accumulation than in MdbHLH160-HA calli under PEG treatment (Fig. 11, A–C), suggesting that MdBT2 decreased MdbHLH160-mediated drought tolerance. The effect of MdBT2 on the MdbHLH160-mediated transcription of target genes was investigated. In the dual-LUC assays, the transcriptional activation of MdbHLH160 on MdSOD1 and MdDREB2A-like promoters was significantly inhibited by MdBT2 co-expression (Fig. 11, D and E). These results indicate that MdBT2 negatively regulates MdbHLH160-mediated MdSOD1 and MdDREB2A-like expression and drought tolerance.
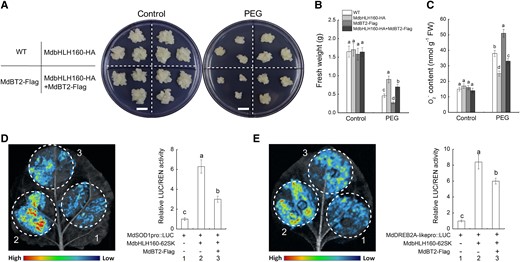
MdBT2 negatively regulates the MdbHLH160-mediated drought tolerance and the transcriptional activation of downstream target genes. (A to C) Growth phenotypes A), fresh weight B), and O2−C) content of different types of transgenic calli under PEG treatment. Bars = 1 cm. D to E) Dual-LUC assays in N. benthamiana leaves. “–” represents the corresponding EV. The data are presented as the means ± SD of 3 biological replicates. Bars with different letters are significantly different at P < 0.05, according to 1-way ANOVA and Duncan's tests.
Discussion
The growth, development, and geographic distribution of crops are greatly limited by drought stress (Kim et al. 2017; Kumar et al. 2019). Studies in many plant species have shown that bHLH TFs play important roles in mediating responses to drought (Mao et al. 2017; Zhan et al. 2023), but the underlying mechanisms remain largely unknown. Apple is an economically important woody plant and the most widely cultivated fruit crop worldwide. Apple production is significantly limited by water deficits, especially in the Loess Plateau (Zhou et al. 2015; Wang et al. 2018), the largest apple-producing region in China. However, few studies have examined the roles of bHLH TFs in regulating the drought tolerance of apples. Here, we identified a bHLH TF, MdbHLH160, which positively regulates the apple drought response. It enhanced apple drought tolerance by directly promoting MdSOD1 and MdDREB2A-like expression. Furthermore, we found that MdBT2 mediated the ubiquitination and degradation of MdbHLH160, a process that can be significantly inhibited by ABA and drought. The results of our study provide insights that will aid future studies of the mechanisms underlying drought-induced ABA signaling and bHLH TF-mediated stress tolerance in plants.
MdbHLH160 inhibits the excessive accumulation of ROS induced by drought by promoting MdSOD1 expression
Drought stress leads to excessive ROS accumulation, which induces oxidative damage in plant cells (Garcia-Caparros et al. 2021). Plants have evolved enzymatic and nonenzymatic scavenging systems to eliminate excessive ROS (Blokhina et al. 2003; Bartwal et al. 2013). The antioxidant enzymes POD, CAT, and SOD are the primary enzymes within the enzymatic scavenging system (Dionisio-Sese and Tobita 1998; Gill and Tuteja 2010). SODs act as the first line of defense against the toxic effects of ROS by dismutating O2− to H2O2; they are ubiquitous in all aerobic organisms and subcellular compartments prone to experiencing ROS-mediated oxidative stress (Apel and Hirt 2004; Gill and Tuteja 2010). Numerous studies have shown that the overexpression of SOD-encoding genes increases SOD enzyme activity, which enhances the abiotic stress tolerance of plants by reducing ROS levels (Hamid Badawi et al. 2004; Wang et al. 2004; Melchiorre et al. 2009). TFs, such as WRKY, bHLHs, and NACs, can regulate the activity of antioxidant enzymes by altering the expression of the genes that encode these enzymes, which affects plant stress tolerance (Zhao et al. 2020b; Jia et al. 2021; Meng et al. 2022; Yu et al. 2023). For example, CsbHLH18 in sweet orange positively regulates cold tolerance by directly and specifically binding to and activating the promoter of CsPOD (Zhao et al. 2020b). We found that MdbHLH160 overexpression significantly inhibited ROS accumulation in transgenic Arabidopsis and apple materials under drought (Figs. 2 and 3). The significant increase in SOD activity and MdSOD1 expression in MdbHLH160-OE plants suggests that MdbHLH160 might play a role in regulating drought tolerance by promoting ROS scavenging (Figs. 2 and 3). Promoter binding and transcriptional regulation assays revealed that MdbHLH160 directly promoted MdSOD1 expression by binding to its promoter (Fig. 5). These results indicate that MdbHLH160 promotes ROS scavenging through the MdbHLH160-MdSOD1 module in response to drought. Given that there are many SOD enzyme-encoding genes in the apple genome (Mei et al. 2023), and the activity of other antioxidant enzymes such as CAT is also significantly increased in MdbHLH160-OE transgenic materials (Figs. 2 and 3), additional studies are needed to clarify whether MdbHLH160 is involved in regulating the expression of other antioxidant enzyme-encoding genes.
MdbHLH160 promotes the enzyme activity and nuclear localization of MdSOD1 through protein interactions
The activity of catalytic enzymes can be affected by interactions with other proteins. For example, the drought stress-responsive AP2/ERF TF MeRAV5 promotes the activity of both MdPOD and MeCAD15 via direct interactions, thereby improving drought tolerance in cassava by modulating H2O2 and lignin accumulation (Yan et al. 2021). In this study, MdbHLH160 was shown to interact with MdSOD1 (Fig. 4, A–D), which prompted us to determine whether MdbHLH160 affected SOD activity via direct interactions. Consistent with this speculation, MdbHLH160 significantly promoted MdSOD1 activity both in vitro and in vivo (Fig. 4, G–I). Since MdbHLH160 is localized to the nucleus (Fig. 1D), the MdbHLH160–MdSOD1 interaction raises another question of whether MdbHLH160 affects MdSOD1 function by affecting its subcellular localization. The excessive accumulation of ROS in the nucleus induces DNA damage, which affects plant growth and stress tolerance (Stacy et al. 1999; Czarnocka and Karpinski 2018; Waszczak et al. 2018). Antioxidant enzymes as well as nonenzymatic antioxidants, such as POD, oxidoreductase, and flavonoids, are also distributed in the nucleus to inhibit excessive ROS accumulation (Saslowsky et al. 2005; Yan et al. 2021; Chen et al. 2021b). For example, the oxidoreductase GmOXR17 has an irregular mottled distribution in the whole cell under normal conditions, but it becomes more concentrated in the nucleus in response to PEG, drought, and H2O2 treatments to reduce the ROS content; the nuclear import of GmOXR17 is enhanced by its interacting protein GmNTF2B-1 (Chen et al. 2021b). In our study, MdSOD1 was widely distributed throughout the cell. However, when MdbHLH160 was co-expressed, the proportion of MdSOD1 protein accumulated in the nucleus increased obviously (Fig. 4, E and F). Moreover, ROS levels were significantly lower in the nuclei of MdbHLH160-OE transgenic apple root cells than in controls after drought treatment (Fig. 4J). These results indicate that MdbHLH160 promotes the activity of MdSOD1 and its accumulation in the nucleus through direct interactions, thus alleviating the oxidative damage caused by drought stress. At present, most of the known ROS-scavenging systems are in the cytoplasm, and the mechanism of ROS regulation in the nucleus remains unclear. Our results will aid future studies of the mechanisms of ROS homeostasis regulation in the nucleus under stress.
MdbHLH160 enhances apple drought tolerance by promoting the expression of MdDREB2 genes
DREB family members are widely present in plant species and involved in regulating the response to a variety of abiotic stresses, such as cold, salt, and drought (Liu et al. 1998; Shen et al. 2003; Bihani et al. 2011; Filyushin et al. 2022). Many studies have shown that bHLH TFs can regulate plant stress responses by regulating the expression of DREB genes, which is most typical in the cold-induced ICE1-DREB1/CBF signaling pathway. The positive role of the bHLH TF ICE1 in the cold response by directly promoting the expression of CBF genes has been identified in several plant species, such as in Arabidopsis (Miura et al. 2007; Tang et al. 2020; Zheng et al. 2023), maize (Jiang et al. 2022), pear (Huang et al. 2015), and apple (An et al. 2022; Yang et al. 2023). In contrast to DREB1/CBF genes which mainly regulate the cold response, DREB2 genes in the A-2 group are mainly involved in drought and salt stress responses (Bihani et al. 2011; Augustine et al. 2015; Chen et al. 2016; Akbudak et al. 2018). Therefore, MdDREB2 genes were screened from the apple genome (Supplemental Fig. S5), and the effect of MdbHLH160 on their expression was determined. The expression of most MdDREB2 genes was up-regulated in MdbHLH160-OE calli under PEG treatment, and the highest expression difference between WT and MdbHLH160 transgenic calli was observed for MdDREB2A-like (MD17G1089700) (Fig. 6A). The direct binding and transcriptional activation of MdbHLH160 on the MdDREB2A-like promoter were verified (Fig. 6, B–G), and the positive role of MdDREB2A-like in modulating apple drought tolerance was identified (Fig. 7). These results indicate that MdbHLH160 can enhance apple drought tolerance by activating MdDREB2A-like expression. Among the up-regulated MdDREB2 genes in MdbHLH160-OE calli (Fig. 6A), MdDREB2A (MD01G1158600) has also been shown to positively modulate drought tolerance (Lian et al. 2021), suggesting that MdbHLH160 might modulate apple drought tolerance by targeting other MdDREB2 genes. Moreover, the higher antioxidant enzyme activity and lower ROS accumulation in various transgenic plants overexpressing DREB2 genes (Zuo et al. 2020; Wu et al. 2022; Chen et al. 2022a), including MdDREB2A (Lian et al. 2021), suggest that the MdbHLH160-MdDREB2s module might also contribute to MdbHLH160-promoted ROS scavenging. Additional studies are needed to clarify these hypotheses.
ABA inhibits the MdBT2-mediated ubiquitination and degradation of MdbHLH160
Ubiquitination is a post-translational modification that plays a key role in the regulation of protein stability (Pickart 2001). The BTB protein BT2 functions as a scaffold protein that participates in the formation of the E3 ubiquitin ligase complex combined with CUL3 and RBX1 proteins (Figueroa et al. 2005; Mandadi et al. 2009). It plays diverse roles in regulating plant growth and abiotic stress responses by mediating the ubiquitination and degradation of different types of TFs (An et al. 2020b). In apple, MdBT2 negatively modulates drought tolerance and drought-induced anthocyanin biosynthesis by promoting the ubiquitination and degradation of MdNAC143 and MdERF38, respectively (An et al. 202 °c; Ji et al. 2020). Several bHLH TFs interact with MdBT2 and undergo MdBT2-mediated ubiquitination and degradation in apples, such as MdbHLH104 (Zhao et al. 2016b), MdbHLH93 (An et al. 2019), MdCIbHLH1 (Zhang et al. 2020), and MdMYC2 (Liu et al. 2023d). In this study, MdBT2 was identified as a MdbHLH160-interacting protein (Fig. 9), indicating a potential role of MdBT2 on MdbHLH160 stability. As expected, MdBT2 promoted the ubiquitination and degradation of MdbHLH160 (Fig. 10). Moreover, the MdbHLH160-promoted drought tolerance and transcription of MdSOD1 and MdDREB2A-like genes were markedly inhibited by MdBT2 co-expression (Fig. 11).
ABA plays a key role in the plant drought response and signaling (Chen et al. 2021a; Yang and Qin 2023). In Arabidopsis, AtBT2 expression is repressed by ABA treatment (Mandadi et al. 2009). In apple, MdBT2 transcription was significantly inhibited by drought and ABA treatment (An et al. 2018b; An et al. 2020c). Moreover, MdBT2 protein stability decreased in response to drought treatment (An et al. 2020c). These studies suggest that drought-induced ABA accumulation and signaling negatively regulate BT2 activity in plants. In this study, MdBT2 promoted and ABA treatment inhibited the ubiquitination and degradation of MdbHLH160 (Figs. 8 and 10). Moreover, ABA treatment inhibited the MdBT2-mediated ubiquitination of MdbHLH160 (Fig. 10D). These results indicate that ABA promotes MdbHLH160 stability by inhibiting the MdBT2-mediated MdbHLH160 ubiquitination in apple. Given that MdbHLH160 expression was significantly up-regulated by PEG and ABA treatment (Fig. 1, B and C), drought-induced ABA signaling might function at both the transcriptional and post-translational levels to enhance MdbHLH160-mediated drought tolerance.
We proposed a working model by which MdbHLH160 modulates the apple drought stress response based on our findings, as well as the findings of previous studies (Fig. 12). Following exposure to drought stress, ABA accumulates, and ABA signaling is activated. ABA signals activate the expression of MdbHLH160 to promote protein accumulation. The gene expression and protein level of MdBT2 were down-regulated, thereby inhibiting the MdBT2-mediated degradation of MdbHLH160. The accumulated MdbHLH160 binds directly to the MdSOD1 promoter to activate its expression, thereby alleviating drought-induced oxidative stress damage. MdbHLH160 also enhances drought tolerance by directly activating MdDREB2A-like expression. Our findings provide insights into the molecular mechanisms of ABA-modulated drought tolerance via the ABA–MdBT2–MdbHLH160–MdSOD1/MdDREB2A-like cascade.
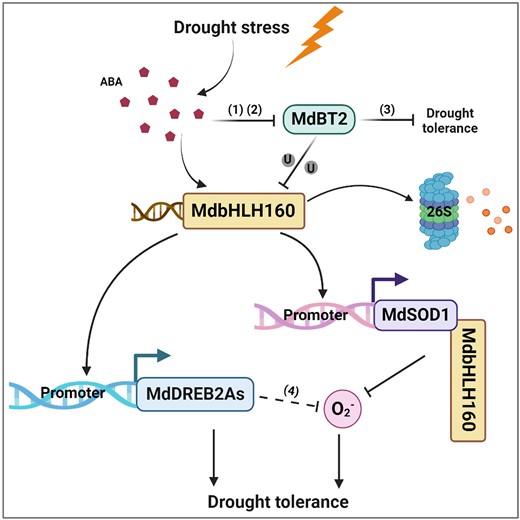
A proposed model illustrating the role of MdbHLH160 in ABA-mediated drought tolerance in apple. In response to drought stress, ABA accumulates, and ABA signaling is activated. ABA signaling activates the expression of MdbHLH160 to promote MdbHLH160 accumulation. ABA signaling also inhibits MdBT2 expression and MdBT2 protein stability, thereby inhibiting the MdBT2-mediated ubiquitination and degradation of MdbHLH160. The accumulated MdbHLH160 directly binds to MdSOD1 and MdDREB2A-like promoters to promote their expression, thus inhibiting excessive ROS accumulation and enhancing the drought tolerance of apple plants. MdbHLH160 also interacts with MdSOD1 to promote its enzyme activity and accumulation in the nucleus, thereby alleviating drought-induced oxidative stress damage in the nucleus: (i) An et al. (2018b); (ii) An et al. (2020c); (iii) Ji et al. (2020); (iv) Lian et al. (2021).
Materials and methods
Plant materials and growth conditions
Plant materials used in this study included apple (“Malus hupehensis”) plants, apple calli (“Orin”), and Arabidopsis thaliana seedlings (“Col-0”). Apple seedlings were grown at 24°C under LD (16-h light/8-h dark) conditions. Arabidopsis seedlings were grown at 23 °C under short-day (SD; 8-h light/16-h dark) conditions before drought treatment. They were planted in pots containing a mixture of nutrient soil and perlite (1:1, v/v) and grown in a growth chamber. Apple calli were cultivated on MS medium containing 1.5 mg L−1 2,4-D and 0.4 mg L−1 6-BA (6-benzyl aminopurine) in the dark at 25 °C unless stated otherwise.
Vector construction and generation of transgenic materials
The coding sequences (CDSs) of MdbHLH160, MdBT2, MdSOD1, and MdDREB2A-like were cloned into the pCambia35S-3HA-GFP, pCambia2300-GFP-Flag, and pCambia2300-GFP vectors, respectively, to construct the overexpression (OE) vectors (MdbHLH160-HA, MdbHLH160-GFP, MdBT2-Flag, MdSOD1-GFP, and MdDREB2A-like-GFP). Selected gene-specific fragments (200 to 300 bp in length) were cloned into the RNAi vector pK7GWIWG2D (with a GFP selection marker) to generate RNAi vectors. The primers are listed in Supplemental Table S1. These vectors were used for the genetic transformation of Arabidopsis thaliana using the floral dip method (Clough and Bent 1998); Agrobacterium tumefaciens (GV3101)-mediated methods were used for apple calli and apple roots as described in previous studies (Meng et al. 2019; Yang et al. 2021b).
Drought stress treatments
For gene expression analysis, 1-mo-old apple plants (“Malus hupehensis”) were treated with PEG6000 (6%; W/V) or ABA (100 μM) under hydroponic conditions (25 °C, continuous white light) (Qi et al. 2019; Tian et al. 2023). Samples of roots and leaves were collected at specified time points.
For the treatment of Arabidopsis seedlings, 5-d-old seedlings cultured in 1/2 MS medium were transferred to treatment medium (1/2 MS medium supplemented with different concentrations of mannitol, placed vertically). After 6 d of cultivation, the primary root length was measured, and root samples were collected to determine physiological indicators. Five plates were set up under each treatment for a total of 3 biological replicates.
For the drought treatment of Arabidopsis plants, 6-wk-old plantlets were subjected to 10 d without water. Three biological replicates were performed for each experiment, and each replicate comprised at least 24 plants of each line. The plantlets before treatment were used as controls.
For the drought treatment of apple plants, 10-wk-old apple plants with similar growth status were subjected to 20 d without water. The plants transformed with EV-OE and EV-RNAi were used as controls. At least 45 plants from 3 biological replicates of each genotype were used for drought treatment. Samples were collected after 12 d of drought treatment for measurements of physiological parameters.
For treatment of apple calli, WT and transgenic calli blocks (0.1 g) with similar growth status were placed on MS medium supplemented with 10% PEG6000. After 20 d of culture, samples were collected to determine physiological parameters. One replicate comprised 5 plates, and a total of 3 biological replicates were performed.
Fluorescence observation by confocal laser scanning microscopy
For subcellular localization analysis, the MdbHLH160-HA-GFP, MdbHLH160-mCherry, and MdSOD1-GFP fusion proteins were transiently expressed with specified combinations in N. benthamiana leaves following a previously described method (Yang et al. 2021b). Free GFP and mCherry proteins were used as controls. To determine the ROS accumulation level in Arabidopsis root tips, the roots were incubated in 20 µM C-H2DCFDA solution for 30 min at room temperature in the dark. After washing off the excess reagents with 0.9% NaCl solution, green fluorescence was observed (Neverisky and Abbott 2016). All fluorescence images were obtained using a confocal laser scanning microscope (TCS-SP8 SR, Leica). GFP fluorescence and C-H2DCFDA signals were detected at 500 to 535 nm after excitation at 488 nm, and mCherry was excited at 543 nm and scanned at 600 to 630 nm.
Protein interaction identification assays
Yeast-2-hybrid (Y2H) assays: The CDSs of MdbHLH160, MdSOD1, and MdBT2 were inserted into the pGBKT7 and pGADT7 vectors. These constructs were co-transformed into yeast strain Y2H-Gold with specified combinations and plated on SD medium lacking Trp and Leu (DDO) at 28 °C. The colonies were transferred to medium lacking Trp, Leu, His, and Ade (QDO) for interaction screening.
Split-LUC complementation: The CDSs of MdbHLH160, MdSOD1, and MdBT2 were cloned into the pRI101-nLUC and pRI101-cLUC vectors. The recombinant vectors were transiently expressed in N. benthamiana leaves with specified combinations by the Agrobacterium-mediated transformation method (Yang et al. 2023). Combinations containing empty nLUC or cLUC vectors were used as controls. The LUC fluorescence signal was captured using a Lumazone Pylon 2048B imaging system (Princeton, New Jersey, USA).
Pull-down: The pET32a (His tag) and pGEX4T-1 (GST tag) vectors were used. The MdbHLH160-HIS, MdSOD1-GST, and MdBT2-GST fusion proteins were expressed in Escherichia coli BL21 and purified with HIS and GST purification columns (Beyotime, Shanghai, China). The pull-down assays were conducted using anti-HIS magnetic beads (Beyotime) at 4 °C overnight. GST protein was used as a negative control. The eluents were detected by WB using anti-HIS and anti-GST antibodies (Beyotime).
Co-IP: The MdbHLH160-HA, MdBT2-Flag, MdSOD1-GFP, and GFP/GFP-Flag proteins were transiently expressed in N. benthamiana leaves with specified combinations. After 3 days of culture, total protein was extracted from leaves and incubated with anti-HA magnetic beads (Beyotime) at 4 °C overnight. The eluents were detected with anti-HA, anti-Flag, and anti-GFP antibodies (Beyotime).
Identification of promoter binding and transcriptional activation activities
Yeast 1-hybrid (Y1H): Selected fragments in MdSOD1 and MdDREB2A-like promoters were cloned into the pAbAi vector. These constructs and MdbHLH160-AD were transformed into the Y1H-Gold strain with specified combinations, with the empty pGADT7 as a negative control. Positive transformants were inoculated on the screening medium (SD base/-Ura-Leu supplemented with Aureobasidin A (AbA) (Yeasen, Shanghai, China)) to test for possible interactions.
EMSAs: MdbHLH160-HIS or HIS protein and biotin-labeled probes were mixed in the binding buffer for 20 min. The MdbHLH160 bound and free probes were separated by an acrylamide gel, and EMSAs were performed using the LightShift Chemiluminescent EMSA kit (Thermo Scientific, Waltham, Massachusetts, USA). The sequences of probes are listed in Supplemental Table S1.
ChIP-qPCR: WT and MdbHLH160-HA transgenic apple calli were treated with 8% PEG6000 for 10 h; they were then harvested and immersed in formaldehyde solution for cross-linking. After sonication, chromatin was immunoprecipitated with or without anti-HA antibody. Relative enrichment values of promoter fragments were detected by qPCR, and the enrichment of WT samples was used as the reference, which was set to 1.0. Four biological replicates were performed, with each containing 4 technical replicates. The primers are listed in Supplemental Table S1.
Dual-LUC): The promoter fragments (2000bp) were cloned into the reporter vector pGreenII 0800-LUC, and the CDS of MdbHLH160 was cloned into the effector vector pGreenII 62-SK. These constructs were transiently expressed in N. benthamiana leaves with specified combinations. After 3 d of culture under normal conditions (25 °C, LD), the plants were treated with 5% PEG6000 for 4 h. The parts surrounding the injection site were collected for luminescence detection with a detection kit (Promega, Madison, Wisconsin, USA). The LUC fluorescence was photographed using a Lumazone Pylon 2048B imaging system.
Protein degradation and ubiquitination analysis
To determine the effects of drought and ABA on the protein stability of MdbHLH160, MdbHLH160-HA transgenic calli were treated with 10% PEG6000 and 100 μM ABA in MS medium supplemented with 250 μM cycloheximide (CHX) (±100 μM MG132). Samples were taken at specified time points, and the protein level of MdbHLH160-HA was detected using anti-HA antibody. For ubiquitination detection in vivo, the protein extracts of the calli were immunoprecipitated using anti-HA magnetic beads. The eluted solution was detected with anti-HA and anti-Ubi (Cell Signaling Technology, USA) antibodies.
To determine the effects of MdBT2 on the stability of MdbHLH160 in vitro, cell-free assays were performed as previously described (An et al. 2019; An et al. 2020a). Briefly, total protein was extracted from WT and MdBT2 (OE/RNAi) transgenic calli, and they were incubated with the purified MdbHLH160-HIS protein at 22 °C. Samples were taken at specified time points, and WB was conducted using anti-HIS antibody. Samples at 0 h or treated with the proteasome inhibitor MG132 (100 μM) were used as controls. For protein degradation assays in vivo, various transgenic calli were treated in MS medium supplemented with 250 μM CHX (±100 μM MG132). Samples were taken at designated time points, and proteins were extracted and detected using anti-HA antibody.
For ubiquitination detection in vitro, the assays were performed as previously described (Zhang et al. 2020). MdBT2-Flag calli were pretreated with 100 μM MG132 for 4 h to obtain the active MdBT2-Flag protein using anti-Flag magnetic beads (Beyotime). MdbHLH160-HIS was incubated with (or without) MdBT2-Flag protein in an incubation buffer at 30 °C for 12 h. The incubation buffer contained 50 mM Tris at pH 7.5, 2 mM of dithiothreitol (DTT), 5 mM of MgCl2, 2 mM of ATP, 100 ng of rabbit E1, 100 ng of human E2, and 2 μg of ubi. The ubiquitinated MdbHLH160-HIS was detected using anti-HIS and anti-Ubi antibodies.
Determination of physiological parameters
The REL and RWC in the leaves were determined following previously described methods (Dionisio-Sese and Tobita 1998; Gaxiola et al. 2001; Hang et al. 2021). Root hydraulic conductivity was determined using a pressure chamber (Model 1505D, PMS Instrument Company, Albany, OR, USA) as described previously (Huo et al. 2020). The MDA content, activity of antioxidant enzymes (SOD, CAT), H2O2 and O2− content, and root vitality were measured using various kits (Suzhou Comin Biotechnology Co., Ltd., Suzhou, China) following the manufacturer's instructions.
Statistical analysis
Data analysis was performed using SPSS22.0 software (IBM, Chicago, IL, USA). The significance of differences among means was determined through 1-way ANOVA, Duncan's test, or Student's t-test (P < 0.05).
Accession numbers
Sequence data from this article can be found in the Genome Database for Rosaceae (GDR; https://www.rosaceae.org) data libraries under accession numbers MdbHLH160 (MD10G1098900), MdSOD1 (MD01G1164700), MdDREB2A-like (MD17G1089700), MdBT2 (MD06G1161300).
Acknowledgments
Thanks to the Horticulture Science Research Center at College of Horticulture, NWAFU for their technical support in this work.
Author contributions
K.M. and F.M. conceived and designed the experiments. K.M., J.Y., Y.S., X.G., and L.Q. performed most of the experiments. Q.M. and N.L. provided the technical assistance. K.M., J.Y., and F.M. analyzed the data and wrote the manuscript.
Supplemental data
The following materials are available in the online version of this article.
Supplemental Figure S1. Protein sequence analysis of MdbHLH160.
Supplemental Figure S2. Identification of MdbHLH160 transgenic Arabidopsis and apple calli.
Supplemental Figure S3. Identification of composite apple plants with genetically modified roots.
Supplemental Figure S4. Identification of purified MdbHLH160-HIS and MdSOD1-GST through WB assays.
Supplemental Figure S5. Phylogenetic analysis and subgroup classification of DREB family proteins in apple and Arabidopsis.
Supplemental Figure S6. Identification of MdDREB2A-like transgenic calli and composite apple plants.
Supplemental Figure S7. Identification of MdBT2 transgenic calli.
Supplemental Table S1. Primers used in this study.
Funding
This work was supported by the National Natural Science Foundation of China (31972391/31701894), the Key S&T Special Projects of Shaanxi Province (2020zdzx03-01-02), the Natural Science Basic Research Plan in Shaanxi Province of China (2023-JC-YB-156), and the China Agriculture Research System of MOF and MARA (CARS-27).
Data availability
The data that support the findings of this study are available in the Supporting Information of this article.
References
Author notes
Ke Mao and Jie Yang contributed equally.
The author responsible for distribution of materials integral to the findings presented in this article in accordance with the policy described in the Instructions for Authors (https://dbpia.nl.go.kr/plphys/pages/General-Instructions) is: Fengwang Ma ([email protected]/[email protected]).
Conflict of interest statement. None declared.