-
PDF
- Split View
-
Views
-
Cite
Cite
Changjiang Cui, Hui Wan, Zhu Li, Nijiang Ai, Baoliang Zhou, Long noncoding RNA TRABA suppresses β-glucosidase-encoding BGLU24 to promote salt tolerance in cotton, Plant Physiology, Volume 194, Issue 2, February 2024, Pages 1120–1138, https://doi.org/10.1093/plphys/kiad530
- Share Icon Share
Abstract
Salt stress severely damages the growth and yield of crops. Recently, long noncoding RNAs (lncRNAs) were demonstrated to regulate various biological processes and responses to environmental stresses. However, the regulatory mechanisms of lncRNAs in cotton (Gossypium hirsutum) response to salt stress are still poorly understood. Here, we observed that a lncRNA, trans acting of BGLU24 by lncRNA (TRABA), was highly expressed while GhBGLU24-A was weakly expressed in a salt-tolerant cotton accession (DM37) compared to a salt-sensitive accession (TM-1). Using TRABA as an effector and proGhBGLU24-A-driven GUS as a reporter, we showed that TRABA suppressed GhBGLU24-A promoter activity in double transgenic Arabidopsis (Arabidopsis thaliana), which explained why GhBGLU24-A was weakly expressed in the salt-tolerant accession compared to the salt-sensitive accession. GhBGLU24-A encodes an endoplasmic reticulum (ER)–localized β-glucosidase that responds to salt stress. Further investigation revealed that GhBGLU24-A interacted with RING-type E3 ubiquitin ligase (GhRUBL). Virus-induced gene silencing (VIGS) and transgenic Arabidopsis studies revealed that both GhBGLU24-A and GhRUBL diminish plant tolerance to salt stress and ER stress. Based on its substantial effect on ER-related degradation (ERAD)–associated gene expression, GhBGLU24-A mediates ER stress likely through the ERAD pathway. These findings provide insights into the regulatory role of the lncRNA TRABA in modulating salt and ER stresses in cotton and have potential implications for developing more resilient crops.
Introduction
RNAs play a wide range of structural, informational, and regulatory roles in cells. Recent studies reveal that the transcription of noncoding RNAs (ncRNAs) is widely present in various cell types (Ariel et al. 2015). ncRNAs are functional RNA molecules with low protein-coding potential. Among them, lncRNAs are RNA transcripts longer than 200 nucleotides (nt), which are abundant in eukaryotes. LncRNAs play a role in regulating gene expression, either in a cis or trans manner, through interactions with histone modification complexes, transcription factors (TFs), Mediator (MED) complexes, RNA polymerase II, etc. (Lam et al. 2014; Vance and Ponting 2014; Marchese et al. 2017). For example, Arabidopsis (Arabidopsis thaliana) elongation factor Tu (ELF18)-INDUCED LONG NONCODING RNA1 (ELENA1) interacts with the fibrillarin 2 (FIB2)/MED subunit 19a (MED19a) complex to enhance target gene transcription (Seo et al. 2019).
With advances in sequencing technology, a large number of lncRNAs that play regulatory components in plants are identified, such as in Arabidopsis (Ben Amor et al. 2009; Di et al. 2014), rice (Oryza sativa; Yuan et al. 2018), tomato (Solanum lycopersicum; Cui et al. 2017), maize (Zea mays; Li et al. 2014), and cotton (Gossypium hirsutum; Zhao et al. 2018). Substantial changes in the expression of lncRNA under stress conditions suggest that lncRNAs are also involved in dynamic regulation of stress responses (Lv et al. 2016; Xu et al. 2016). For instance, under salt stress, 1,661 and 172 lncRNAs were differentially expressed in grape (Vitis amurensis) and wild tea tree (Camellia sinensis) roots (Jin et al. 2020; Wan et al. 2020), and 44 differentially expressed lncRNAs were found in cotton (Zhang et al. 2019a, 2019b). These findings suggest that lncRNAs have potentially important functions in plant responses to salt stress. However, the complexity of types and regulations in lncRNAs makes the molecular mechanisms of plant lncRNA functions still poorly understood. In addition, the regulations of salt stress in cotton by lncRNAs are still elusive.
Endoplasmic reticulum (ER) is the primary site of protein processing in cell. Newly synthesized glycoprotein requires N-glycosylation modifications that transfer mannose-rich oligosaccharides to a motif containing the Asn-X-Ser/Thr (where X is any amino acid other than Pro). The presence of molecular chaperones and folding catalase in ER enables folding and assembling various newly synthesized proteins. However, salt stress often disrupts the folding or assembly of proteins. ER stress is caused by excessive accumulation of unfolded or misfolded proteins in ER (Merksamer et al. 2008; Howell 2013). To prevent errors in protein synthesis and processing, eukaryotes have an ER quality control (ERQC) system that constantly monitors and removes abnormal proteins by either refolding or degradation (Liu and Howell 2016). The ERQC system intersects with N-glycosylation during the processing of glycoproteins by glucosidases I and II (Helenius 2001; Vitale 2001). The final trimming of the Glc residue by glucosidase II results in a quality check by UDP glucose:glycoprotein glucosyltransferase (UGGT), and the protein is either reglycosylated and refolded or directed to the ER-associated degradation (ERAD) pathway to maintain ER homeostasis (Gardner et al. 2000; Pearse et al. 2008; Berner et al. 2018).
ERAD substrates are typically ubiquitinated before degradation by proteasome. This process requires the action of ubiquitin-activating enzyme (E1), ubiquitin-conjugating enzyme (E2), and ubiquitin ligase (E3) (Hoseki et al. 2010). The yeast (Saccharomyces cerevisiae) E3 ligases, Doa10 and Hrd1, play a crucial role in ERAD (Hampton 2002). In mammals, the homologs HRD1 and gp78 have been identified as being involved in the degradation of ERAD-related proteins (Fang et al. 2001; Kikkert et al. 2004; Zhong et al. 2004). The ERAD system also exists in plants, as demonstrated in Arabidopsis (Su et al. 2011). HRD3A, as a part of the HRD1/HRD3 complex, was also the first found ERAD component linked to abiotic stress. The hrd3a mutant enhances Arabidopsis sensitivity to salt stress and reactive oxygen species (ROS; Liu et al. 2011). In Arabidopsis, ubiquitin conjugases UBC32 (a negative regulator of salt tolerance) or UBC33 and UBC34 negatively regulate plant tolerance to drought stress, which belong to the E2 enzyme (Cui et al. 2012; Ahn et al. 2018). Overall, ERAD is shown to have important roles in plant responses to abiotic stresses.
Cotton (Gossypium spp.) is a crucial source of fibers in the global textile industry, representing a substantial portion (35%) of the worldwide fiber production (Meyer 2017). However, the increasing prevalence of environmental stressors, such as climate change and land salinization, is expected to have a profound impact on cotton production. By 2050, it is projected that over 50% of arable land worldwide will experience the detrimental effects of salt damage (Wang et al. 2003; Jamil et al. 2011). Despite cotton plant’s relatively moderate tolerance to salt stress, exposure to high salinity levels disrupts cellular homeostasis, leading to cellular redox imbalance, impeded cellular metabolism, reduced photosynthesis, and ultimately abnormal plant growth (Zhang et al. 2013). Given the potential magnitude of salt damage, research into the underlying mechanisms of salinity tolerance in cotton is crucial (Ma 2011).
In this study, we used a transcriptomic approach and found a salt stress response gene, β-glucosidase-encoding GhBGLU24-A, in cotton plants. Our further studies indicate that GhBGLU24-A interacts with the RING-type E3 ubiquitin ligase (GhRUBL) in vivo. In addition, the effects of GhBGLU24-A and GhRUBL on tolerance to salt and ER stresses were explored by virus-induced gene silencing (VIGS) and transgenic Arabidopsis experiments. Furthermore, we discovered that the lncRNA, trans acting of BGLU24 by lncRNA (TRABA), negatively regulates the expression of GhBGLU24-A. Our results show that the expression of TRABA positively impacts the tolerance of cotton to both salt and ER stresses.
Results
GhBGLU24-A negatively regulates salt tolerance in plants
In our prior transcriptome analysis, we investigated the association between salt stress and the expression of GH_A13G1676 (GhBGLU24-A) in cotton (Cui et al. 2023). Our results showed that the expression of GH_A13G1676 was substantially increased in TM-1 root in response to salt stress; however, no such elevation was noted in the salt-tolerant variety DM37 (Supplemental Fig. S1A). During the developmental stage, GH_A13G1676 was highly expressed in root, fiber, and stamen tissues (Supplemental Fig. S1, B and C). GH_A13G1676 is located on Chr. A13 in G. hirsutum and has an ORF of 3,118 base pairs (bp), comprising 12 exons and a 1,542-bp coding sequence (CDS). Its encoded protein contains a conserved Glyco_hydro_1 domain, a characteristic of the beta-glucosidase (BGLU) family (Fig. 1A). Furthermore, an N-terminal signal peptide encoded by the first 33 nt of the gene was predicted using the tool SignalP 3.0 (https://services.healthtech.dtu.dk/service.php?SignalP-3.0). Subsequently, the protein was found to colocalize with an ER marker protein, being consistent with the prediction by the Predotar online tool (Fig. 1B; https://urgi.versailles.inra.fr/predotar/). Based on its position among BGLU family genes in G. hirsutum, GH_A13G1676 was designated as GhBGLU24-A (Supplemental Table S1 and Fig. S2). Additionally, its homolog in the D subgenome of tetraploid cotton was named GhBGLU24-D (GH_D13G1629).
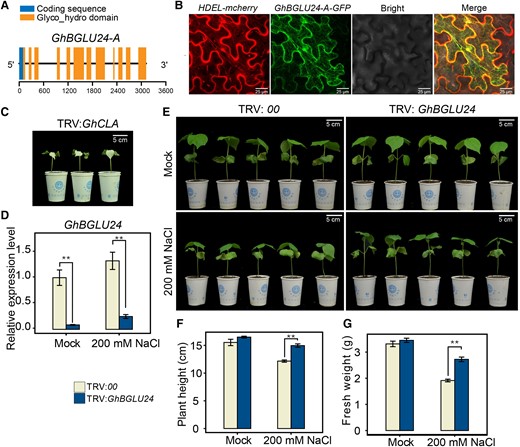
Characterization and functional analysis of the GhBGLU24-A gene. A) The schematic diagram illustrates the structural features of the GhBGLU24-A gene, highlighting the motif encoding the Glyco_hydro_1 domain and the gene intron (represented by a straight line). B) Subcellular localization of the GhBGLU24-A protein was analyzed through a colocalization study with HDEL-mCherry. C) A substantial albino phenotype was observed in the TRV:GhCLA-positive control plants approximately 2 wk after injection. Images were digitally extracted for comparison. D) The silencing efficiency of the GhBGLU24 gene was evaluated using RT-qPCR in TRV:00 and TRV:GhBGLU24 plants. The results indicated that the GhBGLU24 silencing was efficient, as reflected by a statistically significant decrease in transcript levels. E to G) The phenotypic effects of GhBGLU24 silencing on plant height F), fresh weight G), and salt stress tolerance. The indicators were determined after 1 wk of treatment with 200 mM NaCl. Images were digitally extracted for comparison. All data presented are based on an average of 3 independent experiments (n = 15 for each), and the error bars represent the standard error of the mean (SEM). Statistical significance was determined using a t test, with **P < 0.01 denoting significance.
To investigate the regulatory role in plant salt tolerance, the expression of GhBGLU24-A was suppressed through VIGS in cotton and overexpressed in Arabidopsis. The VIGS assay effectively silenced the expression of GhBGLU24-A/D (or GhBGLU24) in TM-1 roots (Fig. 1D). After 1-wk treatment by 200 mM NaCl, the silenced plants showed a salt-tolerant phenotype, including an increased plant height and fresh weight when compared to the control plants (Fig. 1, E to G). Meanwhile, 5 transgenic GhBGLU24-A Arabidopsis lines (OE) and wild-type control plants (WT) were subjected to salt treatment. Reverse transcription quantitative PCR (RT-qPCR) results showed that the expression of GhBGLU24-A gene was substantially increased in transgenic lines (Supplemental Fig. S3A). Under normal conditions (mock), the transgenic seedlings grown on 1/2 MS medium had a more developed root system, as shown by longer main root length and more numbers of lateral roots (NLRs) than WT (Supplemental Fig. S3, B, C, and E). However, under 125 mM NaCl treatment, transgenic lines showed increased sensitivity to salt, with a reduced lateral root number and shorter roots, and showed chlorosis or death (Supplemental Fig. S3, B to F). In addition, when treated with 150 mM NaCl for 1 wk, the growth of transgenic lines was severely inhibited compared to WT, as evidenced by the inhibition of bolting (Supplemental Fig. S3C).
Salt stress causes oxidative stress and ion toxicity, provoking damage to plants. Therefore, we measured superoxide dismutase (SOD), malondialdehyde (MDA), and Na+ levels in VIGS plants and transgenic lines, which are physiological indicators related to the response to salt damage. In cotton, silencing the GhBGLU24 gene increased the SOD activity and reduced the MDA content under salt stress (Supplemental Fig. S4, A and B). The accumulation of Na+ was also decreased and caused a lower Na+/K+ ratio in cotton seedling leaves (Supplemental Fig. S4, C and E). In Arabidopsis, transgenic lines had lower SOD activity and higher MDA content than WT under salt stress (Supplemental Fig. S4, F and G). In addition, more Na+ was significantly accumulated and caused a higher Na+/K+ ratio in OE lines than in WT (Supplemental Fig. S4, H and J).
The GhBGLU24-A promoters of DM37 and TM-1 respond to salt stress at a similar level
In response to salt stress, the GhBGLU24-A gene was differentially expressed in DM37 and TM-1. The promoter sequence plays a crucial role in determining gene expression, particularly variations in cis-regulatory elements. To explore this, we compared the sequences of 2 promoters, proGhBGLU24-ATM-1 from TM-1 and proGhBGLU24-ADM37 from DM37. The result demonstrated there were 23 single nucleotide polymorphisms (SNPs) and 3 insertion/deletions (InDels) in the 3,000 bp promoter region upstream of the start codon (Supplemental Fig. S5).
To further verify whether proGhBGLU24-ATM-1/DM37 promoter variants caused differential gene expression between DM37 and TM-1, we performed the dual luciferase assay to test their activities, in which proGhBGLU24-ATM-1 and proGhBGLU24-ADM37 were employed to drive the expression of the firefly luciferase (LUC) reporter gene (Bian et al. 2020; Lu et al. 2021). Our results showed that there was no significant difference in the relative luciferase activity governed by the 2 promoters under both normal conditions and salt stress (Fig. 2, A and B).
![Analysis of the impact of lncRNA TRABA on GhBGLU24-A transcription. A) Dual luciferase assay was performed to analyze the activity of the proGhBGLU24-A. proGhBGLU24-ATM-1 and proGhBGLU24-ADM37 are promoters of GhBGLU24-A from TM-1 and DM37, respectively. The bioluminescence intensity (counts per second [cps]) generated by the LUC enzyme was measured under normal and salt stress (100 mM NaCl) conditions. The 35S::LUC served as a positive control, and the pGreenII-0800 empty vector served as a negative control. Images were digitally extracted for comparison. B) The relative luciferase enzyme activity was measured using Renilla luciferase (REN) activity as an internal reference. Error bars represent the SEM of 3 independent experiments (n = 3 for each), and asterisks indicate the significance of t tests between mock and salt treatment (**P < 0.01). C) The relative expression level (R.E.L) of TRABA was analyzed by RT-qPCR. Error bars represent the SEM of 3 independent experiments (n = 3 for each), and asterisks indicate the significance of t tests between DM37 and TM-1 (*P < 0.05, **P < 0.01). D) The effect of VIGS-TRABA on the expression of GhBGLU24-A. The R.E.L of GhBGLU24 genes in the roots of VIGS-TRABA-silenced plants (TRV:TRABA) and negative control plants (TRV:00) are presented. The error bars depict the SEM of 3 independent experiments (n = 3 for each). Asterisks indicate the presence of significance between silenced and control plants (**P < 0.01, t tests). E) Presents a schematic diagram of the effector and reporter vector construction utilized in the TRABA transcriptional activation assay, conducted on the proGhBGLU24-A promoter. F) Depicts the detection of bioluminescence intensity (counts per second [cps]) in the transcriptional activation assay, where a, b, c, d, e and f denote experimental designs in which effectors and reporters were coinjected into N. benthamiana. Images were digitally extracted for comparison. G) The relative luciferase activity is presented, using REN activity as an internal reference. The error lines depict the SEM of 3 independent experiments (n = 3 for each). Asterisks indicate the presence of significance between test and control groups (**P < 0.01, t tests).](https://oup.silverchair-cdn.com/oup/backfile/Content_public/Journal/plphys/194/2/10.1093_plphys_kiad530/1/m_kiad530f2.jpeg?Expires=1747895769&Signature=Z7UPd2y3Xn~MI6nwz1cnK4jOE6MMMJWOYQIWF8aOot0ubidZr6H4~I4tv2f121BDIS8u8E640PiIrDSNVwsSghCRxOcjub59ZE6OSVqlvzMBVRLVharYY2GpdGVC5IjLv-ABBTpjQLo8NXx56zWXFyM6C~TCrdrd5HbYLp0bjBwDeW9oRAjfY-5ggxBAMB1AzA-LuWxWjO9qT-4ags-BlRDkcInmLyqYg07iN8dpN9lkS8KHNaCazjiJyBJgyYAYkjnPpYWiDFMokMeyWeMuCTTLMyjZWtJ9a9TbN4KHuva4ejFhagAW0HXw~riBPuTZd~n4BnUokGT7llX6wvoaCg__&Key-Pair-Id=APKAIE5G5CRDK6RD3PGA)
Analysis of the impact of lncRNA TRABA on GhBGLU24-A transcription. A) Dual luciferase assay was performed to analyze the activity of the proGhBGLU24-A. proGhBGLU24-ATM-1 and proGhBGLU24-ADM37 are promoters of GhBGLU24-A from TM-1 and DM37, respectively. The bioluminescence intensity (counts per second [cps]) generated by the LUC enzyme was measured under normal and salt stress (100 mM NaCl) conditions. The 35S::LUC served as a positive control, and the pGreenII-0800 empty vector served as a negative control. Images were digitally extracted for comparison. B) The relative luciferase enzyme activity was measured using Renilla luciferase (REN) activity as an internal reference. Error bars represent the SEM of 3 independent experiments (n = 3 for each), and asterisks indicate the significance of t tests between mock and salt treatment (**P < 0.01). C) The relative expression level (R.E.L) of TRABA was analyzed by RT-qPCR. Error bars represent the SEM of 3 independent experiments (n = 3 for each), and asterisks indicate the significance of t tests between DM37 and TM-1 (*P < 0.05, **P < 0.01). D) The effect of VIGS-TRABA on the expression of GhBGLU24-A. The R.E.L of GhBGLU24 genes in the roots of VIGS-TRABA-silenced plants (TRV:TRABA) and negative control plants (TRV:00) are presented. The error bars depict the SEM of 3 independent experiments (n = 3 for each). Asterisks indicate the presence of significance between silenced and control plants (**P < 0.01, t tests). E) Presents a schematic diagram of the effector and reporter vector construction utilized in the TRABA transcriptional activation assay, conducted on the proGhBGLU24-A promoter. F) Depicts the detection of bioluminescence intensity (counts per second [cps]) in the transcriptional activation assay, where a, b, c, d, e and f denote experimental designs in which effectors and reporters were coinjected into N. benthamiana. Images were digitally extracted for comparison. G) The relative luciferase activity is presented, using REN activity as an internal reference. The error lines depict the SEM of 3 independent experiments (n = 3 for each). Asterisks indicate the presence of significance between test and control groups (**P < 0.01, t tests).
LncRNA TRABA responds stronger to salt stress in DM37 than in TM-1
A transcriptome analysis was conducted to identify the transcription of an unknown RNA located at 835 to 2,131 bp upstream of the start codon of the GhBGLU24-A gene (Supplemental Table S2 and Fig. S6). Results showed that these transcripts accumulated gradually in the DM37 root responding to salinity induction (Supplemental Fig. S7A). RT-qPCR assays also validated the RNA-seq data and showed that the unknown RNA was highly transcribed in response to salt induction in DM37 compared to TM-1 (Fig. 2C). Its coding potential with a length of 1,296 nt (>200 nt) was evaluated as being low to 0.028, implying the RNA was a lncRNA.
Based on a subsequent study of its biological function, the lncRNA was designated as TRABA. Evidence from Pol II ChIP-seq data suggests that TRABA may be transcribed by the Pol II RNA polymerase and underwent processing modification of its poly(A) tail (Supplemental Fig. S7, A and B). A collinearity analysis was performed and revealed that the TRABA gene sequence was present in A genome (A2 in Gossypium arboreum or At in G. hirsutum) but not in D genome (D5 in Gossypium raimondii or Dt in G. hirsutum; Supplemental Fig. S7C).
LncRNA TRABA acts on the promoter of GhBGLU24-A to suppress its expression
LncRNAs are known to regulate the expression of neighboring genes (Engreitz et al. 2016). Our analysis revealed an apparent negative association between the expression of TRABA and GhBGLU24-A. To further investigate the possible regulatory relationship between them and simultaneously rule out the influence of the DM37-specific genetic background, we selected 3 recombinant inbred lines (RILs) (2921-2, 2961-9, and 2994-2) that contain both the TRABA and GhBGLU24-A genes, which were introgressed from the F2:3 population by crossing DM37 and TM-1. These RILs were used to silence TRABA expression. RT-qPCR results showed that TRABA-silenced plants significantly elevated the GhBGLU24 expression under salt stress (Supplemental Fig. S8; Fig. 2D).
LncRNAs have diverse and complex mechanisms for regulating gene expression. To gain deeper insights into the potential regulation of downstream genes by TRABA, transcriptional activation experiments were performed (Fig. 2E). Results showed that the bioluminescence intensity detected in the experimental group (+35S::TRABA) was significantly reduced compared to the control group (+35S::mCherry; Fig. 2F). Similarly, the relative luciferase activity was significantly lower in the presence of the effector vectors (Fig. 2G). These results indicate that the activity of the proGhBGLU24-A promoter is inhibited by TRABA. Furthermore, the comparison was performed for the bioluminescence intensity (Fig. 2F) and the relative luciferase activity (Fig. 2G) driven by proGhBGLU24-As or suppressed by TRABAs between DM37 and TM-1. No differences were found between them, suggesting that the mode of TRABA regulating proGhBGLU24-A in DM37 or TM-1 is not influenced by their sequence variation.
LncRNA TRABA trans-regulates the salt-responsive region of the GhBGLU24-A promoter under salt conditions
To further ascertain the function of the TRABA gene, homozygotes of 35S::TRABA and proGhBGLU24-A::GUS transgenic lines were separately constructed in Arabidopsis, respectively. Using Arabidopsis crosses, double-transformed F1 plants were obtained. Overexpression of TRABA acted as an effector, while the proGhBGLU24-A-driven GUS acted as a reporter (Fig. 3A). Line #0 (proGhBGLU24-A::GUS × WT) was obtained as a negative control. Lines #1, #2, and #3 were derived from the hybridizations of TRABATM-1 and proGhBGLU24-A::GUS, while line #4 was obtained from a cross between TRABADM37 and proGhBGLU24-A::GUS (Fig. 3B). RT-qPCR results in F1 plants indicated that the TRABA expression was substantially higher in lines #1, #2, #3, and #4 than in line #0 (Fig. 3C), while GUS transcription was simultaneously significantly suppressed in these plants and its protein levels were eventually reduced (Fig. 3, D and E).
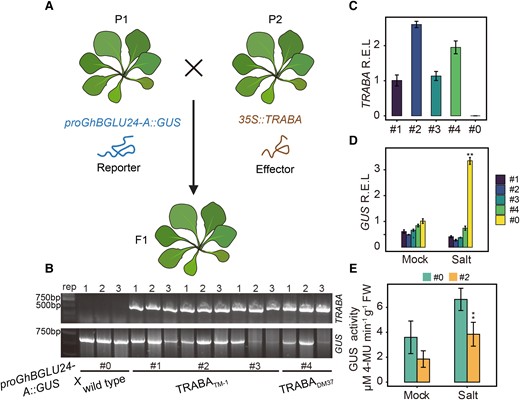
Impact of TRABA on the GhBGLU24-A promoter in transgenic Arabidopsis. A) Schematic illustration of the generation of TRABA/proBGLU24-A::GUS plants. P1 was utilized as the female parent and P2 as the male parent in crosses, yielding F1 offspring with cotransformation of TRABA/proBGLU24-A::GUS. B) Identification of transformed genetic fragments in F1 Arabidopsis. TRABA and proGhBGLU24-A::GUS transformations in Arabidopsis were confirmed using species-specific primers. Lines #1, #2, #3, and #4 were designated as the experimental group and were cotransformed with TRABA/proBGLU24-A::GUS, while line #0 was used as the control group. C) The relative expression levels (R.E.L) of TRABA genes in transgenic Arabidopsis were assessed by RT-qPCR. D) Examination of the relative expression levels of GUS reporter genes under normal and salt stress in different lines. E) Analysis of GUS enzyme activity in lines #0 and #2 under normal and salt stress conditions. Error bars indicate SEM of 3 independent experiments (n = 3 for each), and asterisks denote statistically significant comparison with #0 (**P < 0.01, t tests).
To further investigate the regulatory elements of the GhBGLU24-A promoter, it was fragmented into several lengths (1,825, 1,535, 1,263, 735, and 403 bp) (Supplemental Fig. S9). The dual luciferase assay indicated that all the promoter fragments, regardless of their length, displayed similar activity under normal conditions and upon salt treatment. Additionally, all the promoter fragments were induced by salt (Fig. 4, A and B), demonstrating that the 403 bp region upstream of the start codon plays a crucial role in promoting the transcription of the GhBGLU24-A gene under salt stress. Next, the transcriptional activation assay was used to analyze whether TRABA affects the activity of the u403 promoter. The results showed that TRABA significantly inhibits the activity of the u403 region promoter compared to the control (+35S::mCherry; Fig. 4, C and D). Additionally, the 35S promoter was used as a target for TRABA regulation. However, no effect on 35S promoter activity was observed upon TRABA treatment (Fig. 4, E and F). This suggests that TRABA is specifically involved in regulating the activity of the u403 promoter.
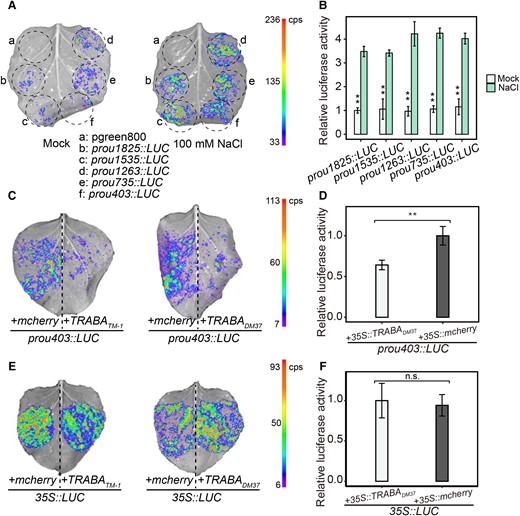
Effect of TRABA on activity of the salt-responsive region of GhBGLU24-A promoter. A, B) Detection of salt response region of GhBGLU24-A promoter using dual luciferase assay. Images were digitally extracted for comparison. The visual presentation A) and relative luciferase activity B) driven by promoter fragments of different lengths under mock and 100 mM NaCl treatment. Error bars represent Se of 3 independent experiments (n = 3 for each), and asterisks indicate the presence of significance between mock and salt stress (*P < 0.05, **P < 0.01, t tests). C, D) Effect of TRABA on u403 promoter activity in response to salt stress as revealed by transcriptional activation assays. The changes in relative luciferase activity after coinjection of effector vector 35S::TRABA and prou403::LUC reporter vector. Images were digitally extracted for comparison. E, F)TRABA-specific regulatory assays. The effect of TRABA on 35S promoter activity was analyzed by transcriptional activation assay. Images were digitally extracted for comparison. Error bars represent Se of 3 independent experiments (n = 3 for each), and asterisks indicate the presence of significance (**P < 0.01; n.s. denotes not significant, t tests).
GhBGLU24-A interacts with GhRUBL in vivo
To search for factors interacting with GhBGLU24-A in cotton, a yeast 2-hybrid (Y2H) assay was performed, and 24 positive clones were obtained. Among them, only 1 protein (encoded by LOC107890770; Supplemental Table S3) was yielded up to 13 times of interactions (at the highest rate). This protein is characterized by 2 conserved structural domains, RING-type and SIAH-type, arranged in an N-terminal to C-terminal manner (Supplemental Fig. S10A), which was deduced to the RING-type E3 ubiquitin ligase, also known as the SEVEN IN ABSENTIA (SINA) protein and named GhRUBL. GhRUBL is also highly expressed in other tissues besides cotton roots (Supplemental Fig. S10B). Further, the interaction between GhBGLU24-A and GhRUBL was confirmed through repeated yeast 2-hybrid assays and firefly luciferase complementation imaging (LCI) assay (Fig. 5, A and B). These results indicated that GhBGLU24-A binds to GhRUBL both in yeast and plant cells. Next, we performed bimolecular fluorescence complementation (BiFC) assays, which enable the detection of protein–protein interactions with subcellular localization information. Confocal observation revealed the GhBGLU24-A interacted with GhRUBL on ER, as seen by ER marker proteins (Fig. 5C). Taken together, these results indicate that GhBGLU24-A interacts with GhRUBL in vivo.
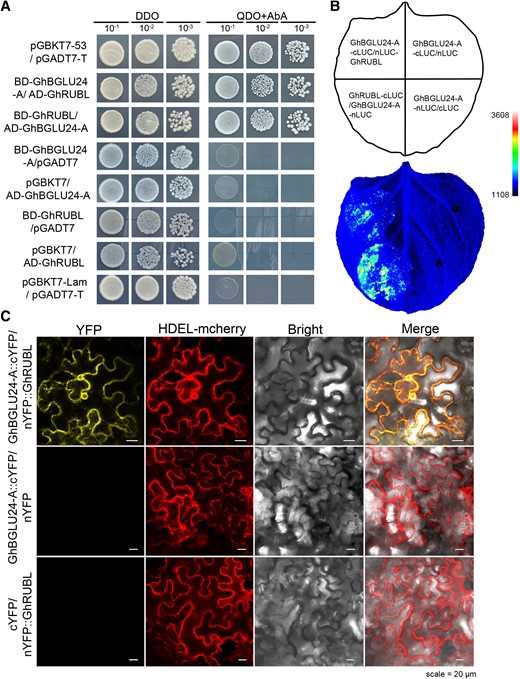
Investigation of the interaction between GhBGLU24-A and GhRUBL in vivo. A) To validate interaction between GhBGLU24-A and GhRUBL proteins, they were fused with the binding domain (BD) and activation domain (AD) of the galactose-responsive TF GAL4. The yeast strain transformed with the positive control (pGBKT7-53 + pGADT7-T) exhibited protein interactions, and the negative control (pGBKT7-Lam + pGADT7-T) did not. The yeast strain transformed with the vector (BD-GhBGLU24-A/pGADT7, pGBKT7/AD-GhBGLU24-A, BD-GhRUBL/pGADT7, and pGBKT7/AD-GhRUBL) served controls. Yeast cells were grown on synthetic defined (SD)/-Leu/-Trp (DDO) and SD/-Ade/-His/-Leu/-Trp + 125 ng/mL Aureobasidin A (AbA) (QDO + AbA) synthetic dropout medium, respectively. B) Firefly LCI analysis of the interaction between GhBGLU24-A and GhRUBL in N. benthamiana. C) BiFC assays showed interactions between GhBGLU24-A and GhRUBL, where HDEL serves as a marker for ER localization.
Silencing GhRUBL enhances salinity tolerance in cotton
To verify the GhRUBL gene role in regulating salt stress response, we silenced the expression of GhBGLU24 or GhRUBL in TM-1 plants via a VIGS experiment. TRV:00 plants in DM37 or TM-1 were used as control plants, respectively. Under normal growth conditions, we observed that TRV:GhRUBL seedlings were smaller in appearance compared to TRV:00 plants (Supplemental Fig. S11D), including plant fresh weight, height, and leaf area (Supplemental Fig. S11, E to G). This suggests that GhRUBL may play a role in regulating cotton growth and development.
To assess the plant sensitivity to salt stress, we subjected the VIGS plants to a 200-mM NaCl solution for 1 wk. To ensure the comparability of salt tolerance among silenced plants, we used relative growth indexes to eliminate growth variations of GhRUBL-silenced plants under normal conditions. The results showed higher relative growth indexes of TRV:GhRUBL and TRV:GhBGLU24 in TM-1, or TRV:00 in DM37 than TRV:00 plants in TM-1, even though TRV:GhRUBL exhibited smaller size under salt stress (Fig. 6, A and B). This indicates that silencing the GhRUBL gene, as well as TRV:GhBGLU24 and DM37 plants, resulted in comparable phenotypic salt tolerance under salt stress conditions.
![Effects of GhRUBL and GhBGLU24 on salt or ER stress, respectively. A) Phenotypes of VIGS plants (TRV:00, TRV:GhBGLU24, and TRV:GhRUBL) after 1 wk of treatment with 200 mM NaCl. Images were digitally extracted for comparison. Scale bar = 3 cm. B) Relative plant weight (fresh weight [FW]), relative shoot weight, relative root weight, and relative plant height of VIGS plants. Calculation of relative growth indices = growth indices under salt stress/growth indices under normal conditions. The data are presented as the mean ± SEM of 3 independent experiments (n = 15 for each). A 1-way ANOVA was conducted to determine the significance of the differences in means (*P < 0.05, **P < 0.01). C) Phenotypes of ER stress–treated GhBGLU24-A transgenic Arabidopsis. The figure depicts the impact of ER stress, induced by 1.0 and 2.5 mM DTT treatments, on the phenotypes of both OE and WT plants for a period of 3 and 2 wk, respectively. Scale bar = 2 mm. D) Statistical analysis of phenotypes. The phenotypes under 1.0 mM DTT treatment were categorized into 3 levels of severity, i.e. RN, GB, and YB, In addition, G, Y, and D indicate normal, etiolated, and dead phenotypes under 2.5 mM DTT treatment. The Se of 3 experimental replicates (n ≥ 20 for each) are indicated by error bars, and asterisks represent significant t test results between WT and OE plants (**P < 0.01). E) Phenotypic observations of WT and OE plants. The figure displays the phenotypes of 4-wk-old transgenic plants after 10 d of exposure to 5.0 mM DTT. Images were digitally extracted for comparison. Scale bar = 2 cm.](https://oup.silverchair-cdn.com/oup/backfile/Content_public/Journal/plphys/194/2/10.1093_plphys_kiad530/1/m_kiad530f6.jpeg?Expires=1747895769&Signature=sq4zdO0jPJnc3b1pKoRWDiWt3wzZMSRzhj2o3T9vnJBHe6j0VnEgEn4sTV~xCiymDZkZS8PqLR44k8nEdJVhkg1CUmVQIVImdVT9fNEQAVbnlnNrwP4O-di1imnNuOu0yLiVP67Yj0RtSicBXQ6Pr5njyrcoFJrD3lKQPeqzFhL1SFewGB9-qSNDc~7gHaQqWAU2s8q6HZD8GoIKgxUFD7tz3Z7X~eIKxn-U1Vle~rj5yzYL6I7JRP0G3eOFyyMcu7SGt9m2sfnG8VMWZCKn3Wvsod4adBXTHAPAFCNOD3X5~UoX~X5v8xLftvIAVPydB3-ljKzyCw4oaFD1By9S~w__&Key-Pair-Id=APKAIE5G5CRDK6RD3PGA)
Effects of GhRUBL and GhBGLU24 on salt or ER stress, respectively. A) Phenotypes of VIGS plants (TRV:00, TRV:GhBGLU24, and TRV:GhRUBL) after 1 wk of treatment with 200 mM NaCl. Images were digitally extracted for comparison. Scale bar = 3 cm. B) Relative plant weight (fresh weight [FW]), relative shoot weight, relative root weight, and relative plant height of VIGS plants. Calculation of relative growth indices = growth indices under salt stress/growth indices under normal conditions. The data are presented as the mean ± SEM of 3 independent experiments (n = 15 for each). A 1-way ANOVA was conducted to determine the significance of the differences in means (*P < 0.05, **P < 0.01). C) Phenotypes of ER stress–treated GhBGLU24-A transgenic Arabidopsis. The figure depicts the impact of ER stress, induced by 1.0 and 2.5 mM DTT treatments, on the phenotypes of both OE and WT plants for a period of 3 and 2 wk, respectively. Scale bar = 2 mm. D) Statistical analysis of phenotypes. The phenotypes under 1.0 mM DTT treatment were categorized into 3 levels of severity, i.e. RN, GB, and YB, In addition, G, Y, and D indicate normal, etiolated, and dead phenotypes under 2.5 mM DTT treatment. The Se of 3 experimental replicates (n ≥ 20 for each) are indicated by error bars, and asterisks represent significant t test results between WT and OE plants (**P < 0.01). E) Phenotypic observations of WT and OE plants. The figure displays the phenotypes of 4-wk-old transgenic plants after 10 d of exposure to 5.0 mM DTT. Images were digitally extracted for comparison. Scale bar = 2 cm.
GhBGLU24-A and GhRUBL are involved in ER stress
GhBGLU24-A-GhRUBL interacts on ER organelles. In addition, E3 ubiquitin ligase was shown to be involved in ER stress (Deng et al. 2013). Therefore, it is likely that GhBGLU24-A-GhRUBL is involved in ER stress response. To verify this conjecture, VIGS was carried out to knock down the above gene expression in cotton. DTT is usually employed as an ER stress reagent. The silenced plants grown to 4 wk old were treated with 5-mM DTT solution for 1 wk. The results showed that both GhBGLU24-VIGS and GhRUBL-VIGS plants exhibited a reduced response to ER stress compared to the control plants (TRV:00), as indicated by a lower incidence of necrosis or abscission in the seedling leaves (Supplemental Fig. S12). These results suggest that GhBGLU24-A and GhRUBL may be involved in the ER stress response. Additionally, the DM37 plants also displayed remarkably high tolerance to ER stress, as evidenced by fewer incidences of necrosis or abscission in the seedling leaves (Supplemental Fig. S12).
The involvement of GhBGLU24-A in ER stress was further investigated by subjecting GhBGLU24-A-OE Arabidopsis lines to ER stress caused by DTT. Under 1.0-mM DTT treatment, the GhBGLU24-A-OE lines exhibited distinct sensitive phenotypes described as Relatively Normal (RN), Greenish-Big (GB), and Yellowish-Big (YB). The proportion of RN type was higher in WT than in OE lines, whereas that of GB and YB types was significantly higher in OE lines than in WT (Fig. 6, C and D). Under 2.5-mM DTT treatment, the resultant phenotypes were classified into 3 categories: green (G), yellow (Y), and death (D). The results showed that an increased concentration of DTT (2.5-mM) resulted in a higher death rate in the GhBGLU24-A transgenic plants than in WT, and its proportion of the milder G and Y phenotypes was significantly lower in OE lines than in WT (Fig. 6, C and D). Furthermore, when Arabidopsis seedlings were treated with 5 mM DTT, leaf chlorosis was more severe in OE plants than WT based on chlorosis leaf areas and leaf numbers, and leaf withering (Fig. 6E). These results indicated that OE lines were more sensitive to ER stress than WT.
To investigate the potential effect of GhBGLU24-A on ERQC system, the expression of ERQC system genes coding key chaperones and catalase for protein folding and assembly was analyzed in silenced plants. The results showed that silencing GhBGLU24 genes significantly enhanced the expressions of ER type α-mannosidase I (MNS3), MNS5, Heat shock 70 kDa protein 4 (HSP70-4), and Calnexin 1 (CNX1) in salt stress compared to the control (Supplemental Fig. S13A). Conversely, the expression levels of their homologs were significantly lower in the overexpressing GhBGLU24 Arabidopsis lines compared with WT plants under salt stress (Supplemental Fig. S13B). Similarly, the expression levels of MNS3, MNS5, HSP70-4, and CNX1 genes were significantly reduced in silenced TRABA plants of 2921-2, 1961-9, and 2994-2 compared to control plants under salt stress (Supplemental Fig. S13C). Notably, the Arabidopsis homologs, AtMNS3 and AtMNS5, were reported to be involved in processing N-glycans and be involved in the ERAD of misfolded glycoproteins (Liebminger et al. 2009; Huttner et al. 2014); Chaperones AtHSP70-4 were able to assist in folding unfolded or misfolded proteins or degrading damaged proteins under stress conditions (Lee et al. 2009). Calnexin is a lectin chaperone in ER that promotes the folding of glycoproteins and retains folded intermediates to participate in the ERQC system (Montpetit et al. 2022). Taken together, these results indicated that GhBGLU24 may be involved in ER stress pathway.
LncRNA TRABA regulates salt and ER stresses in cotton
To evaluate the impact of TRABA on salt and ER stress tolerance in cotton, 2 RILs 2921-2 and 2994-2 were randomly selected for VIGS experiments. Results showed that TRABA-silenced plants in both RILs were more severely inhibited by salt stress than the control plants after being treated with 200 mM NaCl for 1 wk, as indicated by a reduction in fresh weight and plant height (Fig. 7, A and B). In addition, TRABA-silenced plants also exhibited increased sensitivity to DTT (Fig. 7, C and D). This suggests that TRABA, as an upstream regulatory gene of GhBGLU24-A, plays a role in modulating cotton response to both salt and ER stresses.
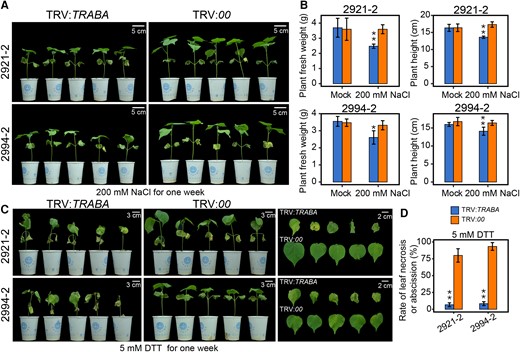
Salt or ER stress on VIGS-TRABA plants. A) Illustrative comparison of the growth phenotypes of VIGS plants after 1 wk of treatment with 200 mM NaCl. Images were digitally extracted for comparison. B) Statistical analysis of plant fresh weight and height of VIGS plants. The data presented are the average of 3 independent experiments (n = 15 for each), and the error bars indicate the SEM. Statistical significance between silenced and control plants is indicated by asterisks (*P < 0.05, **P < 0.01, t test). C) Visual comparison of the appearance of VIGS plants after 1 wk of treatment with 5 mM DTT. Images were digitally extracted for comparison. D) Statistical analysis of leaf necrosis or abscission in VIGS plants. The data presented are the average of 3 independent experiments (n = 10 for each), and the error bars indicate the SEM. Statistical significance between silenced and control plants is indicated by asterisks (**P < 0.01, t test).
Discussion
GhBGLU24-A and GhRUBL may negatively regulate salt tolerance through the ERAD pathway
Previous research showed that BGLU genes were involved in salt stress pathways, such as in alfalfa with genes like MtBGLU21, MtBGLU22, MtBGLU28, and MtBGLU30, which were rapidly activated by salt induced (Yang et al. 2021). Similarly, most tomato SlBGLU genes were salt-induced expressed (Wei et al. 2022). However, in spite of these findings, there is limited evidence for the involvement of BGLU genes in regulating salt tolerance in plants. In this study, we found that silencing GhBGLU24-A improved the cotton tolerance to salt stress (Fig. 1, D to F; Supplemental Fig. S4, A to E). Conversely, ectopic expression of the GhBGLU24-A gene reduced the Arabidopsis tolerance to salt stress (Supplemental Figs. S3 and S4, F to J). Moreover, knockout mutants of atbglu1 and atbglu19 were less sensitive to NaCl treatment, indicating that these genes negatively regulate plant salt tolerance (Cao et al. 2017), consistent with GhBGLU24-A. Our results revealed that both GhBGLU24-A and its interacting protein GhRUBL act as negative regulators of salt tolerance in cotton. Compared to BGLU genes, RING-type E3 ligase is well documented in salt tolerance. For example, the loss-of-function mutant of the RING-type E3 ligase, SALT TOLERANCE RING FINGER 1 (strf1) in Arabidopsis, exhibited enhanced salt tolerance and reduced ROS accumulation (Tian et al. 2015). Overexpression of the RING-type E3 genes, OsSIRP1, OsSIRP3, and OsSIRP4 (SALT-INDUCED RING PROTEIN) in rice, resulted in increased sensitivity to salt stress (Hwang et al. 2016; Park et al. 2018; Kim et al. 2021). Similarly, the RING-type E3, GhSARP1 (SALT-ASSOCIATED RING FINGER PROTEIN) in cotton, was identified as a negative regulator of salt stress (Liu et al. 2016). Thus, these studies suggest that GhBGLU24 and GhRUBL play an important role in regulation of salt stress responses.
The proper redox environment in ER is necessary for protein folding and processing that includes the formation of protein disulfide bridges (Deng et al. 2013). DTT, a reducing agent, can penetrate ER membrane and inhibit the formation of disulfide bonds, leading to accumulating misfolded proteins in ER and inducing acute ER stress (Altuntaş and Terzi 2020). Several responsive genes to DTT-induced ER stress are well documented in Arabidopsis (Mishiba et al. 2019; Guo et al. 2021; Fuchs et al. 2022; Kim et al. 2022). There is still a lack of research on BGLU genes involved in ER stress. Our experimental results showed that tolerance to DTT was enhanced in GhBGLU24-A-silenced cotton plants and reduced in transgenic GhBGLU24-A Arabidopsis lines, respectively (Fig. 6, C to E; Supplemental Fig. S12), suggesting that GhBGLU24-A is involved in ER stress response pathway. The ERAD in plants is responsible for maintaining ER stress homeostasis. The RING-type E3 ligase modifies target proteins through ubiquitination and subsequently directs them to 26S proteasome for degradation (Al-Saharin et al. 2022). For example, the ubiquitination and proteasomal degradation of ATG13, FREE1, and VPS23A are regulated by SEVEN IN ABSENTIA OF ARABIDOPSIS THALIANA (SINATs) (Qi et al. 2020; Xia et al. 2020). Additionally, the Arabidopsis SINAT5, putative ortholog of GhRUBL, facilitates ubiquitination and degradation of NAC1 and LHY (Xie et al. 2002; Park et al. 2010). The ubiquitin–proteasome system (UPS) is widely considered to be involved in the ERAD pathway, such as the ERAD-mediating RING finger protein (EMR) in Arabidopsis, which plays a role in the ERAD and affects brassinosteroid (BR) signaling under ER stress conditions (Park et al. 2018). GhBGLU24-A was shown to interact with the RING-type E3 ligase GhRUBL on ER possibly through the C-terminal SINA domain (Fig. 5C; Yang et al. 2017; Hu et al. 2021). Silencing GhBGLU24 and GhRUBL genes showed increased tolerance to DTT (Supplemental Fig. S12). In addition, silencing GhBGLU24 increased the expression of MNS3 and MNS5 genes on the ERAD pathway (Supplemental Fig. S13; Lee et al. 2009; Liebminger et al. 2009; Huttner et al. 2014), which indicated that DTT-induced ER stress cloud be alleviated through ERAD process. Tian et al. (2019) demonstrated that pretreatment of plants with tunicamycin or DTT resulted in low plant mortality under high salt stress conditions, implying that protein homeostasis in ER is related to the salt tolerance of plants. Furthermore, Reyes-Impellizzeri and Moreno (2021) suggested that ERAD was crucial for plant salt stress response. Based on these findings, it is inferred that GhBGLU24-A and GhRUBL influence plant salt tolerance by mediating the ERAD pathway under salt stress.
The possible role of GhBGLU24-A in the glycoprotein degradation under ER stress
The glycosylation of N-terminal asparagine residues in membrane and secretory proteins on ER is a crucial posttranslational modification. It is estimated that 70% of eukaryotic proteins require this modification to achieve proper folding and function (Mueller and Meador-Woodruff 2020). In the context of the ERQC, N-glycosylation plays a vital role by serving as a signal for displaying the folding state of proteins. This system monitors protein folding by utilizing N-glycosylation signals to identify incompletely folded or misfolded glycoproteins, which are then either refolded in ER or degraded through ERAD pathway (Xu and Ng 2015). Therefore, glycosidases that potentially act on protein N-oligosaccharides are important for the recognition and transmission of ERQC signals. For example, glucosidases I (GI) and II (GII) sequentially remove the terminal and intermediate glucose residues. The exposed sugar signal is then recognized by the ER lectin, such as calnexin, to initiate protein folding (D’Alessio et al. 2010). If refolding is unsuccessful, the glycoprotein is sent for degradation through ERAD pathway (Vembar and Brodsky 2008). N-Glycan is then demannosylated by mannosidases Mns1/ERManI (α1,2-mannosidase 1, ER class I α-mannosidase) and Htm1/EDEM (homologous to mannosidase 1, ER degradation–enhancing α-mannosidase-like protein) and carries the signal for misfolded proteins to ERAD complex, which includes the ER membrane anchor of ubiquitin ligases (e.g. E3) and accessory factors, before ultimately being degraded by proteasome (Zhang et al. 2020). BGLUs play an important role in various physiological processes in plants by hydrolyzing glycosidic bonds. For example, AtBG1 (AtBGLU18) and AtBG2 (AtBGLU33) in Arabidopsis hydrolyze ABA-GE to rapidly replenish plant ABA levels during osmotic stress (Lee et al. 2006; Xu et al. 2012). In addition, some BGLUs in rice were shown to hydrolyze salicylate glucoside and gibberellin glucosylate (Himeno et al. 2013; Hua et al. 2013). The GhBGLU24-A protein in cotton is a member of the glucosidase I family and possesses potential glycolytic functions. In addition, lectin and mannosidase genes that recognize and trim N-glycans are modulated in expression by GhBGLU24 (Supplemental Fig. S13). Based on these, it is hypothesized that the GhBGLU24-A protein acts on N-glycosylation signals in the ERQC system, thereby influencing the recognition and degradation of target proteins through the E3 protein GhRUBL. Further investigation is necessary to confirm this hypothesis in our next study.
LncRNA TRABA trans-inhibits GhBGLU24-A promoter activity under salt stress
LncRNA regulations in gene expression are a complex and diverse process. LncRNAs are reported to regulate gene expression at the transcriptional and posttranscriptional levels (Chen 2016; Quinn and Chang 2016). Previous studies indicated that lncRNAs either activate or repress the expression of regulated genes, making them versatile regulatory molecules in gene expression control (Zhang et al. 2019a, 2019b). In this context, our studies demonstrated that lncRNA TRABA repressed the GhBGLU24-A expression (Figs. 2 to 4). Further analysis of our transcriptional activation and transgenic experiments indicated that TRABA regulated the expression of reporter genes (LUC, GUS) by acting on the proBGLU24-A promoter, suggesting that TRABA regulates gene transcription by acting on the promoter rather than posttranscriptional regulation of transcripts. In terms of gene transcription, lncRNAs regulate gene expression either in a cis or trans manner, by interacting with proteins, RNA, and DNA (Rai et al. 2019). In double-transformed Arabidopsis, TRABA was able to repress the proGhBGLU24-A promoter activity even though the TRABA and proGhBGLU24-A genetic fragments were randomly inserted into the Arabidopsis genome (Fig. 3). This finding highlights the potential for TRABA to regulate gene expression through distance-independent, trans-acting mechanisms, as previously observed with the FLAIL lncRNA in Arabidopsis flail mutants (Jin et al. 2021). However, it should be noted that in inhibition of GhBGLU24-A gene expression by TRABA, the induction of expression in response to salt was not completely removed, including in DM37 and especially in 2921-2 (Fig. 2D; Supplemental Fig. S1A). This suggests that the expression of GhBGLU24-A is influenced by additional factors. In addition, the salt-induced upregulation of GhBGLU24-D in DM37 may be attributed to a specific sequence variation in the GhBGLU24-D promoter, leading to dysregulation by TRABA (Supplemental Fig. S14). Further investigation is required to understand the mechanisms that TRABA regulates GhBGLU24-A and differentially expresses in the 2 accessions. For example, it remains unclear whether TRABA recruits TFs, chromatin-modifying protein complexes, and RNA-binding proteins or directly interacts with promoter DNA sequences through direct base pairing (Thomson and Dinger 2016; Statello et al. 2021; Zhao et al. 2022). Based on our results, we can hypothesize that TRABA may repress gene expression by affecting the recruitment of transcription-associated proteins at promoters. Previous studies have shown that lncRNAs can recruit transcriptional repressors to target gene promoters, inhibiting their expression (Negishi et al. 2014). Alternatively, lncRNAs can hinder gene expression by forming RNA–DNA triplets directly with promoter sequences to block TF binding sites (Tao et al. 2021). These mechanisms could be involved in the regulation of TRABA. In addition, while we found that SNPs or InDels in the TRABA promoter led to variants of cis-acting elements, it is still uncertain whether these variants affect TRABA expression (Supplemental Fig. S15). It is also important to consider the potential influence of other factors, such as epigenetic modification (Zhao et al. 2018; Tao et al. 2021).
In conclusion, our study provides genetic evidence that TRABA plays an essential role in controlling salt tolerance and ER stress in cotton, specifically in the promoter activity of GhBGLU24-A. Furthermore, we identified GhRUBL, a RING-type E3 ubiquitin ligase, as a key negative regulator of salt tolerance and ER stress that interacts with GhBGLU24-A. Notably, a distinct regulator, TRABA, repressed salt-inducible genes between cotton accessions, suggesting that this mechanism is involved in the complex fine-tuning of cotton responses to salt tolerance during domestication and improvement. This study sheds light on the complex interplay between various regulatory mechanisms that govern cotton response to salt stress.
Materials and methods
Plant materials
Cotton (G. hirsutum) acc. TM-1 (a salt-sensitive line), G. hirsutum acc. DM37 (a salt-tolerant line developed from the introgression of G. hirsutum race yucatanense acc. TX-1046; Zhang et al. 2016), and RILs 2921-2, 2961-9, and 2994-2 (derived from TM-1 × DM37) were employed in this study. Cotton seedlings were grown in a greenhouse under controlled conditions, with a 16-h photoperiod and a day/night temperature of 25 °C/23 °C. Nicotiana benthamiana for transient expression tests and Arabidopsis (A. thaliana; Col-0) for genetic transformation were grown in a growth chamber with a daytime temperature of 22 °C and nighttime temperature of 18 °C, under a 16-h photoperiod.
VIGS experiment
VIGS experiments were performed as previously described (Burch-Smith et al. 2004; Pang et al. 2013). The conserved gene segments (GhBGLU24-A/D, GhRUBL-A/D, and TRABA) were cloned into the TRV2 vector to generate the silencing vectors. The chimeric vectors are denoted as TRV:GhBGLU24, TRV:GhRUBL, and TRV:TRABA, respectively. To validate the effectiveness of the silencing system, the empty vector TRV:00 was used as a negative control, and TRV:GhCLA served as a positive control. Agrobacterium tumefaciens strain GV3101 carrying the silencing vector was injected into 10-d-old cotton cotyledons. Based on gene expression patterns, GhBGLU24 and GhRUBL were selected for silencing in G. hirsutum acc. TM-1, while TRABA was silenced in 2921-2, 2961-9, and 2994-2. The efficacy of the silencing was further evaluated using RT-qPCR.
Genetic transformation of A. thaliana
To construct the gene overexpression vector, the full-length cDNA of GhBGLU24-A (XM_016820471), GhRUBL, and TRABA were cloned into the PK2GW7.0 vector and driven by 35S promoter. The full length of TRABA sequence was obtained by identifying the longest transcript in RNA-seq assembly, which was served as the template for primer design to amplify and clone the complete TRABA gene. Then the full length of TRABA from the first nucleotide to the last was confirmed in DM37 by the alignment to TM-1. Specific details of the primers used can be found in Supplemental Table S4. To evaluate the activity of the GhBGLU24-A promoter, the 1,825 bp promoter sequence isolated from upstream of the start codon was inserted into the vector pBI121 to construct the proBGLU24-A::GUS vector. These vectors were transformed into A. thaliana (Col-0) using the flower dipping method previously described (Zhang et al. 2006). Homozygous transgenic plants were obtained and used for subsequent experiments or hybridization. To examine the effect of TRABA on the activity of the GhBGLU24-A promoter in a stable expression context, double-transformed plants (proBGLU24-A::GUS and 35S::TRABA) were generated by crossing the corresponding single-transformed plants.
Subcellular localization of GhBGLU24-A
To detect the subcellular localization of GhBGLU24-A, the full-length CDS of GhBGLU24-A was inserted into the pBinGFP4 vector, and the HDEL-mCherry was used as a marker for ER localization. The recombinant vectors were transformed into N. benthamiana leaves as previously described (Yang et al. 2000). The localization of the protein was determined by observing the fluorescence signal under a laser confocal microscope Leica SP8 (Leica, Wetzlar, Germany). Confocal images of fluorescent signals were collected as described below.
Y2H screening and assays
Y2H assays were performed using the Matchmaker GAL4 vector system according to the manufacturer's protocol (Clontech, USA). The CDS of GhBGLU24-A were fused with the bait vector pGBKT7 and transformed into yeast strain Y2H Gold. A cDNA library from cotton was generated by fusing ds-cDNA with vector the pGADT7-Rec and transforming it into the yeast strain AH109. The Y2H bait strain was mated with the host yeast strain. And the mixture was incubated at 30 °C for 4 to 5 d on a synthetic dropout medium that lacked adenine, histidine, leucine, and tryptophan supplemented with 125 ng/mL Aureobasidin A (shortly SD/-Ade/-His/-Leu/-Trp/AbA). Further small-scale Y2H assays were performed to confirm the yeast library screening results. The full-length CDS of putative interaction partners (GhRUBL) were inserted into pGADT7 and cotransformed into the Y2H Gold cell, along with universal positive (pGBKT7-p53/pGADT7) and negative (pGBKT7-Lam/pGADT7) controls.
LCI assays
For the interaction assay between GhBGLU24-A and GhRUBL, the CDS of GhBGLU24-A and GhRUBL was fused to a fragment of luciferase (nLUC or cLUC) in the pCAMBIA-nLUC and pCAMBIA-cLUC vector, respectively. The recombinants were then transformed into A. tumefaciens strain GV3101 and transiently expressed in N. benthamiana leaf epidermal cells. After 2 to 3 d of incubation at 23 °C, the relative luciferase activity was determined as previously described (Zhou et al. 2018) using 3 biological replicates for each experiment.
BiFC assays
The pSPYNE (VN) and pSPYCE (VC) plasmids were used for this experiment as previously described (Qian et al. 2021). Full-length GhBGLU24-A and GhRUBL were fused with the C- or N-terminal of yellow fluorescent protein (cYFP or nYFP), to construct the GhBGLU24-A::cYFP and nYFP::GhRUBL plasmids. Negative controls consisted of combinations of GhBGLU24-A::cYFP with VN and nYFP::GhRUBL with VC. These constructs were transformed into A. tumefaciens strain GV3101 and incubated overnight. The cultures were then resuspended with infiltration buffer (10-mM MES, 10-mM MgCl2, and 0.2-mM acetosyringone) to an optical density (OD600) of approximately 0.6 to 0.8. Equal volumes of each culture were mixed and coinfiltrated into N. benthamiana leaves, which were then incubated in the dark for periods of 2 to 3 d.
Fluorescence was observed using a Leica SP8 confocal microscope (Leica, Wetzlar, Germany). The GFP, YFP, and mCherry fluorophores were excited with lasers of 488, 514, and 543-nm lasers, respectively, at 20% intensity. Light emission of GFP was detected at wavelengths of 510 to 540 nm, YFP at 530 to 550 nm, and mCherry at 575 to 630 nm, respectively. Pinhole (0.7 airy unit), gain, and detector offset were kept consistent throughout the experiment. Three independent experiments were performed to ensure the reliability of the results.
Salt and DTT stress treatment
To assess the expression levels of GhBGLU24-A and TRABA in response to salt stress, 3-leaf stage seedlings of acc. DM37 and acc. TM-1 were transplanted into Hoagland solution containing 200-mM NaCl, as described in previous studies (Yan et al. 2014). Three biological replicates of roots and leaves were collected at 0, 6, 12, 24, and 48 h after treatment, flash frozen, and stored at −80 °C for RNA extraction.
The growth of gene-silenced cotton plants was also evaluated in response to salt stress and DTT stress. Soil-grown control (TRV:00) and gene-silenced seedlings at the 3-leaf stage, grown in pots containing an organic substrate and vermiculite (1:1 vol/vol), were irrigated with 200 mM NaCl or 5 mM DTT solution, respectively, for 1 wk. The phenotypes of the seedlings were observed and evaluated, and parallel samples were taken at 0 and 48 h of treatment for the determination of physiological markers or RNA extraction.
The growth of transgenic Arabidopsis plants under salt stress and ER stress was evaluated. Arabidopsis (WT and OE) were grown in 1/2 MS medium containing either NaCl (125 mM) or DTT (2.5 mM) for 2 wk or DTT (1.0 mM) for 3 wk, and their root system architecture was analyzed using the EZ-Rhizo software (Armengaud et al. 2009). For seedling stage tests, 4-wk-old Arabidopsis grown in pots containing an organic substrate and vermiculite (1:1 vol/vol) were treated with salt stress (150 mM NaCl solution) or DTT stress (5 mM DTT solution). Treated samples were taken at 0 and 48 h for subsequent physiological index measurements and RNA extraction.
Transient expression assay
The 1,825-bp promoter of the GhBGLU24-A gene from TM-1 and DM37 was cloned into the pGreenII 0800 vector, resulting in the plasmids proBGLU24-ATM-1::LUC and proBGLU24-ADM37::LUC, respectively. In addition, promoter fragments of various lengths (ranging from the start codon to −1,535, −1,263, −735, and −403 bp upstream) were inserted into the pGreenII 0800 vector and designated as prou1535::LUC, prou1263::LUC, prou735::LUC, and prou403::LUC, respectively. The full-length cDNA of TRABA was cloned into the PK2GW7.0 vector. These constructs were introduced into A. tumefaciens (strain GV3101), and the agroinfiltration of N. benthamiana leaves was performed using a combination of the transformed Agrobacterium strains. The relative luciferase activities were measured 2 to 3 d after agroinfiltration, using a dual luciferase assay system. Each experiment was performed with 3 independent biological replicates.
Histochemical GUS staining and fluorimetric GUS assays
Histochemical analysis of GUS-stained Arabidopsis tissues was performed using previously described methods (Dedow et al. 2022). The fresh tissues carefully excised from the transformed Arabidopsis plant (proGhBGLU24-A::GUS) were washed with 0.1 M phosphate buffer (pH 7.0). And then, the tissues were immersed in a GUS staining buffer (1-mM X-gluc, 10-mM Na2EDTA, 2-mM potassium ferricyanide/ferrocyanide, and 0.1% [v/v] Triton X-100 in 0.1-M Na2HPO4, pH 7.0) at 37 °C for approximately 12 h. Chlorophyll was then removed from the tissues using 75% (v/v) alcohol, and the tissues were observed under a stereo microscope.
Fluorimetric GUS assays were carried out according to GUS gene quantitative detection kit (Coolaber, Beijing, China) instruction. The double transformed and control Arabidopsis root tissues were used to determine the enzymatic GUS activity on the SpectraMax iD5 (Molecular Devices, San Jose, USA). Standard curves were prepared with a range of increasing concentrations of 4-methylumbelliferone. Mean values and Se were calculated based on data from 3 independent experiments.
RNA extraction and RT-qPCR assays
Total RNA was isolated from the cotton and Arabidopsis roots as described in the manufacturer instructions of MolPure Plant RNA Kit (YEASEN, Shanghai, China). The cDNA synthesis was performed using the Hifair III 1st Strand cDNA Synthesis Kit (YEASEN, Shanghai, China). As reference genes, GhHis3 was used for cotton and AtUBQ5 for Arabidopsis. The RT-qPCR experiments were conducted using Hieff qPCR SYBR Green Master Mix (YEASEN, Shanghai, China) and LightCycler 480 (Roche Diagnostics GmbH, Mannheim, Germany). Primer information can be found in Supplemental Table S4. In this study, all designed primers were assessed for specificity of amplification using e-PCR software. Additionally, the amplification products were subjected to blast analysis to ensure their specificity in cotton. The expression profiling of the genes was evaluated by calculating the mean values and Se of 3 independent experiments, using the comparative cycle threshold method described by Livak and Schmittgen (2001).
Measurements of SOD, MDA, Na+, and K+
According to the instructions for the Superoxide Dismutase assay kit (Jiancheng, Nanjing, China) and the Malondialdehyde assay kit (Jiancheng, Nanjing, China), approximately 0.1 g of fresh cotton and Arabidopsis leaves tissue was collected and rinsed with distilled water for the measurement of SOD activity and MDA content. The absorbance values were determined using the SpectraMax iD5 spectrophotometer (Molecular Devices, San Jose, CA, USA).
The cotton and Arabidopsis leaves were thoroughly rinsed with distilled water before being dried in an oven set at 80 °C. The dried tissue was subjected to nitric acid digestion and microwave treatment for 0.5 h at 180 °C using a microwave digestion machine (Milestone, Sorisole, Italy). The presence of sodium and potassium ions was determined using the PinAAcle 900 atomic absorption spectrophotometer (PerkinElmer, Waltham, MA, USA). Each experiment was performed with 3 biological replicates.
In this study, TM-1 assembly was used as a reference genetic coordinate (Hu et al. 2019).
Statistical analyses
Statistical analyses were performed using R software (version 4.2.1). The specific statistical tests and corresponding P-values are detailed in the respective figure legends.
Accession numbers
Sequence data from this article can be found in the GenBank/EMBL data libraries or TM-1 genome version of ZJU (GH, Hu et al. 2019) and NAU-NBI (Gh, Zhang et al. 2015) under accession numbers GhBGLU24-A, LOC107895207 (GH_A13G1676, Gh_A13G1209); GhRUBL, LOC107890770 (GH_D09G1943, Gh_D09G1747); GhMNS3, LOC107905885 (GH_D05G1518, Gh_D05G1412); GhMNS5, LOC107890903 (GH_D09G1818, Gh_D09G1614); GhHSP70-4, LOC107959100 (GH_A05G0973, Gh_A05G0823); GhCNX1, LOC107938025 (GH_A07G0521, Gh_A07G0399); GhHis3, LOC107950429 (GH_D03G0404, Gh_D03G0370); AtUBQ5; AT3G62250; AtMNS3, AT1G30000; AtMNS5, AT1G27520; AtHSP70-4, AT3G12580; and AtCNX1, AT5G20990.
Acknowledgments
We are grateful to Dr. Tianzhen Zhang, Yan Hu (Zhejiang University, China), and Kang Liu (Nanjing Agricultural University) for providing the yeast library for protein interaction screening. This study was also supported by the high-performance computing platform of Bioinformatics Center, Nanjing Agricultural University.
Author contributions
B.Z. and C.C. conceived and designed the project. C.C., H.W., Z.L., and N.A. performed the experiments. C.C. analyzed data. C.C. and B.Z. wrote the manuscript. B.Z. supervised and revised the manuscript. All authors read and approved the manuscript.
Supplemental data
The following materials are available in the online version of this article.
Supplemental Figure S1. Expression pattern of GH_A13G676 (GhBGLU24-A) gene during salt stress and developmental stages.
Supplemental Figure S2. Physical locations of BGLU family genes on chromosomes in G. hirsutum.
Supplemental Figure S3. Salt stress response in GhBGLU24-A transgenic A. thaliana.
Supplemental Figure S4. Effects of silencing and overexpressing GhBGLU24-A gene on physiological indexes in cotton and Arabidopsis, respectively.
Supplemental Figure S5. Comparative analysis of the GhBGLU24-A promoter region between DM37 and TM-1.
Supplemental Figure S6. Gene sequence of TRABA.
Supplemental Figure S7. Comprehensive analyses of the TRABA gene in cotton (G. hirsutum).
Supplemental Figure S8. Analysis of TRABA gene silencing efficiency.
Supplemental Figure S9. Schematic diagram of different promoter fragment activity analysis experiments.
Supplemental Figure S10. Characterization analysis of the GhRUBL gene.
Supplemental Figure S11. Effects of VIGS (TRV:00, TRV:GhBGLU24, and TRV:GhRUBL) on plant growth.
Supplemental Figure S12. Different VIGS plants (TRV:00, TRV:GhBGLU24, and TRV:GhRUBL) respond to ER stress.
Supplemental Figure S13. Effect of GhBGLU24 or TRABA on the expression of genes related to the ER stress pathway.
Supplemental Figure S14. u403 promoter sequence.
Supplemental Figure S15. Comparative analysis of the TRABA promoter region between DM37 and TM-1.
Supplemental Table S1. Gene ID and its corresponding name of glycoside hydrolase family 1 gene in upland cotton.
Supplemental Table S2. Coverage of uniquely mapped reads on TRABA genes.
Supplemental Table S3. Identification of proteins interacting with GhBGLU24-A by Y2H screening
Supplemental Table S4. Primer sequences were used in this study.
Funding
This work was supported by the Key Scientific and Technological Project of XPCC (2021AB010 to B.Z.) and the Key Scientific and Technological Projects of the Eighth Division of XPCC (2019NY01 to B.Z.). The funding body had no role in the design of the study and collection, analysis, and interpretation of data or in writing the manuscript.
Data availability
The data underlying this article are available in the article and in its online supplementary material.
Dive Curated Terms
The following phenotypic, genotypic, and functional terms are of significance to the work described in this paper:
References
Author notes
The author responsible for distribution of materials integral to the findings presented in this article in accordance with the policy described in the Instructions for Authors (https://dbpia.nl.go.kr/plphys/pages/General-Instructions) is Baoliang Zhou ([email protected]).
Conflict of interest statement. None declared.