-
PDF
- Split View
-
Views
-
Cite
Cite
Yanxin Jiang, Anran Zhang, Wenjing He, Qingqing Li, Bosi Zhao, Hongjiao Zhao, Xubo Ke, Yalu Guo, Piaoyun Sun, Tongwen Yang, Zheng Wang, Biao Jiang, Junjun Shen, Zheng Li, GRAS family member LATERAL SUPPRESSOR regulates the initiation and morphogenesis of watermelon lateral organs, Plant Physiology, Volume 193, Issue 4, December 2023, Pages 2592–2604, https://doi.org/10.1093/plphys/kiad445
- Share Icon Share
Abstract
The lateral organs of watermelon (Citrullus lanatus), including lobed leaves, branches, flowers, and tendrils, together determine plant architecture and yield. However, the genetic controls underlying lateral organ initiation and morphogenesis remain unclear. Here, we found that knocking out the homologous gene of shoot branching regulator LATERAL SUPPRESSOR in watermelon (ClLs) repressed the initiation of branches, flowers, and tendrils and led to developing round leaves, indicating that ClLs undergoes functional expansion compared with its homologs in Arabidopsis (Arabidopsis thaliana), rice (Oryza sativa), and tomato (Solanum lycopersicum). Using ClLs as the bait to screen against the cDNA library of watermelon, we identified several ClLs-interacting candidate proteins, including TENDRIL (ClTEN), PINOID (ClPID), and APETALA1 (ClAP1). Protein–protein interaction assays further demonstrated that ClLs could directly interact with ClTEN, ClPID, and ClAP1. The mRNA in situ hybridization assay revealed that the transcriptional patterns of ClLs overlapped with those of ClTEN, ClPID, and ClAP1 in the axillary meristems and leaf primordia. Mutants of ClTEN, ClPID, and ClAP1 generated by the CRISPR/Cas9 gene editing system lacked tendrils, developed round leaves, and displayed floral diapause, respectively, and all these phenotypes could be observed in ClLs knockout lines. Our findings indicate that ClLs acts as lateral organ identity protein by forming complexes with ClTEN, ClPID, and ClAP1, providing several gene targets for transforming the architecture of watermelon.
Introduction
Plant architecture is determined by the organization and activities of shoot apical meristem (SAM) and axillary meristem (AM), as well as by the successive development of lateral organs, including leaves, branches, and flowers (Wang et al. 2018). Previous studies have characterized numerous genes that form a complex regulatory network, which play crucial roles in regulating the development of lateral organs, including the initiation of lateral branches, morphogenesis of leaves, and differentiation of flowers (Zik and Irish 2003; Du et al. 2018; Luo et al. 2021). Watermelon (Citrullus lanatus) is an important species of vegetable crop that belongs to the Cucurbitaceae family. The lobed leaves of watermelon commonly initiate from the flank of the SAM, while the branches, tendrils, and unisexual flowers initiate from the axils of leaves (Supplemental Fig. S1). All these lateral organs collectively establish the morphology of watermelon plants. However, the molecular mechanisms controlling the identity of lateral organs in watermelon remain to be elucidated.
Several genetic regulators of branch identity, including Lateral suppressor (Ls) in tomato (Solanum lycopersicum), and its putative orthologs in rice (Oryza sativa), namely, MONOCLUM 1 (MOC1), and Arabidopsis (Arabidopsis thaliana) LATERAL SUPPRESSOR (LAS), directly regulate the initiation of AM to induce the formation of branches or tillers (Schumacher et al. 1999; Greb et al. 2003; Li et al. 2003). Ls, LAS, and MOC1 are members of the GRAS family of transcription factor genes and have been shown to integrate multiple internal genes that regulate the initiation of branches from leaf axils. Previous studies on Arabidopsis have demonstrated that LAS functions upstream of REVOLUTA (REV), which is necessary for activating the transcription of SHOOT MERISTEMLESS (STM) during the initiation of AM (Otsuga et al. 2001). In rice, the degradation of MOC1 is mediated by the anaphase promoting complex/cyclosome (APC/C) E3 ubiquitin ligase complex in leaf axils, which decreases the expression level of a meristem identity gene, HOMEOBOX 1 (OsH1). This subsequently inhibits the initiation of AM and formation of tillers (Lin et al. 2012). DELLA protein SLENDER RICE 1 (SLR1) is an inhibitor of gibberellin (GA) signaling and prevents the degradation of MOC1 to regulate tillering (Hirano et al. 2010). Additionally, a high level of GA can activate the APC/C complex, promote the degradation of MOC1 in the AM, and inhibit tillering in rice (Lin et al. 2020).
Tendrils are specialized filamentous organs that are derived from various morphological structures, including shoot-derived, leaflet-derived, and inflorescence-derived tendrils (Sousa-Baena et al. 2018). The Chiba Tendril-Less (CTL) melon (Cucumis melo) and the CG9192 cucumber (Cucumis sativus) are mutant lines belonging to the Cucurbitaceae family that are devoid of tendrils, and the tendrils are replaced by tendril-like branches in these mutant lines (Mizuno et al. 2015; Wang et al. 2015). Fine mapping studies have demonstrated that the CTL and CG9192 mutants are produced due to mutations in CmTCP1 (homologous to the Arabidopsis TEOSINTE BRANCHED1/CYCLOIDEA/PCF family member TCP1) and CsTEN, respectively (Mizuno et al. 2015; Wang et al. 2015). Further studies have demonstrated that CmTCP1 and CsTEN are homologous genes. A defective mutation in the Class I homeodomain leucine zipper (HD-ZIP) transcription factor gene, TENDRIL-LESS (TL), in garden pea (Pisum sativum) leads to the conversion of tendrils into leaflets (Gourlay et al. 2000). It has been demonstrated that a mutation in VvGAI1, which encodes a DELLA protein, results in GA insensitivity, dwarf stature, and absence of tendrils compared to wild-type (WT) grapevine (Vitis vinifera) plants (Boss and Thomas 2002).
Leaf morphogenesis primarily includes the initiation of leaves from the flank of the SAM, determination of leaf polarity, and leaf outgrowth and bifacial flattening (Efroni et al. 2010; Du et al. 2018). Previous studies have identified numerous proteins that play key regulatory roles in leaf initiation and development, including NO APICAL MERISTEM (NAM), CUP-SHAPED COTYLEDONS (CUC), ANGUSTIFOLIA (AN), ROTUNDIFOLIA (ROT), LATE MERISTEM IDENTITY1 (LMI1), PINOID (PID), and PHANTASTICA (PHAN) (Souer et al. 1996; Benjamins et al. 2001; Kim et al. 2002, 2003; Vroemen et al. 2003; Andres et al. 2017).
Floral meristems are continuously produced during the transition from the vegetative phase to the reproductive phase, and result in the production of flowers (Prusinkiewicz et al. 2007; Sablowski 2015). The floral identity genes, LEAFY (LFY), APETALA1 (AP1), and CAULIFLOWER (CAL), form a regulatory feedback loop and cooperate to initiate the process of floral differentiation (Gustafson-Brown et al. 1994; Liljegren et al. 1999; Goslin et al. 2017).
Commercial watermelon has the characteristics of multiple branches, and it is time-consuming and labor-costing to remove them manually, especially in protected cultivation (Dou et al. 2022). Therefore, we would like to identify a gene module to manipulate the formation of watermelon lateral branches. It is well known that Ls/LAS/MOC1 homologous proteins play pivotal roles in the determination of shoot branching or tillering in model plants (Schumacher et al. 1999; Greb et al. 2003; Li et al. 2003). However, in this study, we observed that the Ls protein of watermelon (ClLs) control the identity of all lateral organs. Knockout of ClLs in watermelon led to the lack of tendrils, branches, and flowers in the leaf axils and resulted in the formation of round leaves without lobed margins. Using yeast two-hybrid system with ClLs as a bait to screen a cDNA library from watermelon shoot apex, we identified ClTEN, ClPID, and ClAP1 as partners of ClLs. The results of in vivo and in vitro biochemical experiments further indicated that the ClLs interacted with ClTEN, ClPID, and ClAP1 at the protein level. In situ hybridization studies revealed that the mRNA distribution patterns of ClLs overlapped with those of ClTEN, ClPID, and ClAP1. In addition, knockout of ClTEN, ClPID, and ClAP1 resulted in the lack of tendrils, formation of round leaves, and floral diapause, respectively, in watermelon. Altogether, these findings indicated that the ClLs underwent functional expansion and not only determined shoot branching but also regulated the identity of all lateral organs.
Results
Knockout of ClLs results in lacking lateral organs at leaf axils and producing round leaves without lobed margins
In order to identify the Ls homologous gene of watermelon (C. lanatus), a phylogenetic tree of the GRAS family genes of watermelon and Arabidopsis (A. thaliana) was constructed (Supplemental Fig. S2). The GRAS family of watermelon comprises 37 members, of which Cla97C10G188130 (ClLs) shared the highest sequence similarity with the Arabidopsis LAS gene (Supplemental Fig. S2). To determine the function of ClLs in watermelon, two target DNA fragments located in the first exon of the ClLs gene were selected for constructing the gene editing vectors for CRISPR/Cas9-mediated knockout (Fig. 1A). Six ClLs gene knockout lines, including ClLsCR-A, ClLsCR-B, and ClLsCR-M, carrying a homozygous allele with a 65, 31, and 2 bp deletion, respectively, were obtained by genetic transformation of the inbred line “TC” of watermelon (Fig. 1A). All the knockout lines were identified to lack tendrils, branches, and flowers in the leaf axils as compared to WT plants through phenotypic observations (Fig. 1B, Supplemental Fig. S3C). Interestingly, the leaves of the ClLsCR-A, ClLsCR-B, and ClLsCR-M lines were fused and rounded, while the leaves of the WT (“TC” watermelon) plants had obvious lobulations (Fig. 1D). The ClLs gene was repeatedly knocked out in a second watermelon inbred line “YL”, and eight lines were obtained, including ClLsCR-1, ClLsCR-3, and ClLsCR-7, carrying a homozygous allele with a 29, 46, and 4 bp deletion, respectively (Fig. 1A). Phenotypic observations revealed that these three lines also lacked tendrils, branches, and flowers in the leaf axils (Fig. 1C, Supplemental Fig. S3A and B). The leaves of these knockout lines also appeared round owing to the absence of lobulation (Fig. 1D). Inspection of the abaxial surface of the leaves of ClLs knockout lines revealed that the lateral veins were more extensively connected by veinlets owing to the absence of lobulation (Supplemental Fig. S3D and E).
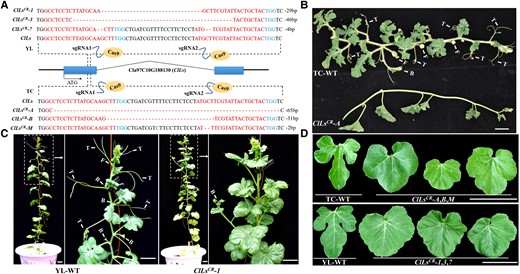
ClLs knockout repressed the initiation of tendrils, branches, and male and female flowers, and resulted in the formation of round nonlobulated leaves in watermelon. A) Schematic representation of the ClLs with two CRISPR/Cas9 target sites in the watermelon inbred lines, “YL” and “TC”. The promoter and terminator regions are indicated by black lines. The sequences of the sgRNA are depicted in red, while the PAM sites are indicated in blue. Genotypic identification of ClLs knockout plants with “YL” and “TC” background revealed partial deletions in the DNA sequence of the first exon. B and C) The “TC”-WT and “YL”-WT plants developed normal tendrils, branches, and flowers. The ClLsCR-A (“TC” background) and ClLsCR-1 (“YL” background) knockout lines lacked tendrils, branches, and flowers. D) Comparison of the leaf morphology of WT (“TC”-WT and “YL”-WT) and ClLs knockout plants. Images were digitally extracted for comparison. T, tendril; F, flower; B, branch. Scale bars = 5 cm.
ClLs interacts with ClTEN, ClPID, and ClAP1 in vitro and in vivo
To further understand the possible cause for functional expansion of ClLs in watermelon, a yeast two-hybrid screen was performed against the cDNA library of watermelon shoot apex using ClLs as a bait and isolated multiple possible interacting proteins, including ClTEN (Cla97C11G212330.1, a TCP family protein), ClPID (Cla97C03G057690.1, a serine/threonine kinase), and ClAP1 (Cla97C08G153400.1, a MADS-box family protein), whose functions have not yet been verified in watermelon (Supplemental Table S1). A series of biochemical assays in vitro and in vivo were performed to further verify the interaction relationship between ClLs and ClTEN, ClPID, and ClAP1. The results of yeast two-hybrid assays demonstrated that ClLs could interact with ClTEN, ClPID, and ClAP1, and their corresponding yeasts exhibited blue staining with X-α-gal (Fig. 2A). The yeasts transformed with a combination of negative control plasmids were incapable of proliferating (Fig. 2A). The pull-down assay further verified that ClLs interacted with the ClTEN, ClPID, and ClAP1 proteins in vitro (Fig. 2B to D). These protein–protein interactions were then confirmed in vivo by firefly luciferase complementation imaging (LCI) assays and bimolecular fluorescence complementation (BiFC) assays with the leaves of Nicotiana benthamiana. Strong fluorescence signals were detected in the N. benthamiana leaves infected with Agrobacterium tumefaciens transformed with the ClLs-ClTEN, ClLs-ClPID, and ClLs-ClAP1 plasmid combinations, while no signals were detected in the negative controls (Fig. 2E and F). These results indicated that ClLs mediated tendril initiation, leaf morphogenesis, and flower development, possibly by forming complexes with ClTEN, ClPID, and ClAP1, respectively.
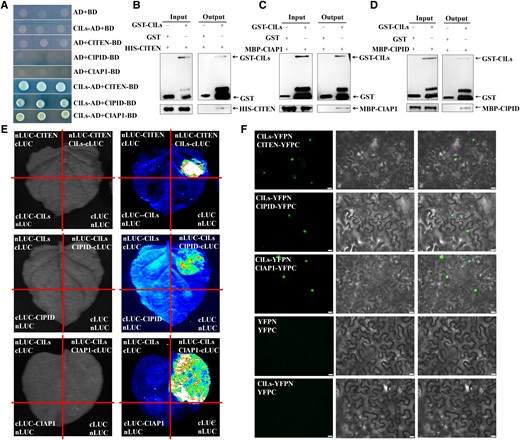
ClLs directly interacts with ClTEN, ClPID, and ClAP1 at the protein level. A) The interaction of ClLs with ClTEN, ClPID, and ClAP1 were determined by yeast two-hybrid assays. B to D) ClLs interacted with ClTEN, ClPID, and ClAP1 in vitro, as determined by the GST pull-down assay. Combinations of GST and His-ClTEN, GST and MBP-ClPID, GST and MBP-ClAP1 were used as controls. The black arrows indicate the target protein bands. E) The results of LCI analysis revealed that ClLs interacted with ClTEN, ClPID, and ClAP1. The images on the left were captured under a bright field, while the LUC images on the right were captured using a low-light cooled CCD imaging apparatus. ClLs-cLUC or nLUC-ClLs was transiently co-expressed with nLUC-ClTEN, nLUC-ClPID, and nLUC-ClAP1 in N. benthamiana, and the remaining combinations were used as controls. F) The interactions between ClLs and ClTEN, ClPID, and ClAP1 were determined by the BiFC assay. The columns from left to right indicate fluorescence, differential interference contrast, and merged channels, respectively. YFPC and YFPN represent the fusion of a given protein with the C- or N-terminus of YFP in frame, respectively. Scale bars = 20 μm.
In situ hybridization of ClLs, ClTEN, ClPID, and ClAP1 revealed overlapping expression signals in AM
In order to intuitively explore the expression patterns of ClLs, ClTEN, ClPID, and ClAP1 in the SAM of watermelon, the accumulation of the transcripts of these four genes was observed by in situ hybridization assays (Fig. 3). The mRNA signals of ClLs were detected in the leaf primordia and AM (Fig. 3B and C). No signals were detected following hybridization with the sense probe of ClLs (Fig. 3A). ClTEN displayed specific signals in the tissues below the SAM, which could be attributed to the tendril primordium, while no signals were detected in the control group (Fig. 3D to F). Interestingly, the transcriptional pattern of ClPID was similar to that of ClLs, whose mRNA signals accumulated in the leaf primordia and AM (Fig. 3H and I), while no signals were detected in the control group (Fig. 3G). The mRNA of ClAP1 also displayed strong signals in the AM, which indicates that ClAP1 might be involved in floral primordia production during the transition from the vegetative to the reproductive period of growth (Fig. 3K and L). The ClAP1 sense probe was hybridized as a negative control (Fig. 3J). These findings indicated that the transcriptional sites of ClLs overlapped with ClTEN, ClPID, and ClAP1, respectively, in the lateral tissues of watermelon.
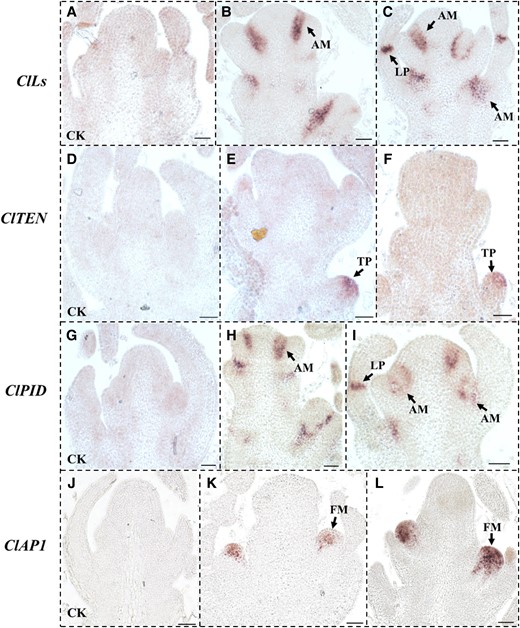
Analyses of the mRNA expression patterns of ClLs, ClTEN, ClPID, and ClAP1 by in situ hybridization in the SAM of the “TC” line of watermelon. A to C) Analysis of the expression of ClLs in SAM by in situ hybridization. D to F) The mRNA signals of ClTEN were enriched in the tendril primordium. G to I) The results of in situ hybridization assays revealed that the distribution of ClPID mRNA overlapped with that of ClLs mRNAs in SAM. J to L) Results of in situ hybridization assays for determining the expression of ClAP1 in the floral meristem. A, D, G, and J) The sense probes of ClLs, ClTEN, ClPID, and ClAP1 were hybridized as controls. The arrows in panels B, C, E, F, H, I, K, and L indicate the mRNA signals. SAM, shoot apical meristem; LP, leaf primordia; TP, tendril primordia; AM, axillary meristem; FM, floral meristem. Scale bars = 50 μm.
Knockout of ClTEN leads to tendril diapause or morphological variations
In order to verify the function of ClTEN in watermelon, the ClTEN gene was knocked out using a CRISPR/Cas9 system. Five ClTEN knockout lines, including ClTENCR-1, ClTENCR-2, and ClTENCR-3, carrying a homozygous allele with a 9, 1, and 2 bp deletion, respectively, were obtained (Fig. 4A). Compared to the WT plants, none of the three knockout lines produced tendrils at the early stage of development (Supplemental Fig. S4A and B). The WT plants continuously produced climbing tendrils during growth, up to a length of 30 nodes (Fig. 4B). However, the ClTENCR-3 line did not produce any tendrils in the leaf axils during this time (Fig. 4B), while the ClTENCR-1 line produced tendrils in the form of leaves (Fig. 4C). Additionally, the ClTENCR-2 line did not produce any tendrils; however, its branches were curved and flexible, like tendrils compared with the WT plants (Supplemental Fig. S4C and D). These findings indicate that ClTEN is involved in the formation of tendrils in watermelon.
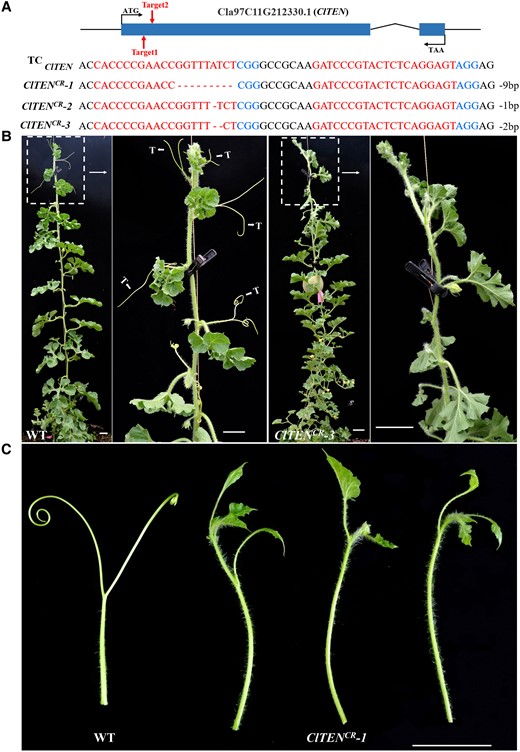
ClTEN knockout inhibited the development of tendrils. A) Schematic representation of the ClTEN gene depicting the two CRISPR/Cas9 target sites. The sequence targeted by sgRNA is indicated in red, while the PAM sites are depicted in blue. The hyphens denote deleted nucleotides. B) Comparison of the phenotypes of WT (“TC”-WT) and mutant (ClTENCR-3) plant. The leaf axils of “TC”-WT plant contained a normal tendril each, while the ClTENCR-3 plant lacked tendrils. Images were digitally extracted for comparison. C) Images of the normal tendrils of WT plant and the abnormal tendrils of the ClTENCR-1 plant. Images were digitally extracted for comparison. T, tendril. Scale bars = 5 cm.
Knockout of ClPID prevents leaf lobulation and induces the formation of round leaves
Previous studies have demonstrated that the PID protein of cucumber is involved in the development of lateral organs, including the morphogenesis of leaves and flowers (Liu et al. 2019; Song et al. 2019). We knocked out the watermelon ClPID gene using the CRISPR/Cas9 method, and 12 ClPID knockout lines were obtained, including ClPIDCR-1, ClPIDCR-2, and ClPIDCR-3 (Fig. 5A). Compared to that of the WT plants, the ClPID gene of the ClPIDCR-1, ClPIDCR-2, and ClPIDCR-3 knockout lines had 147, 2, and 4 bp deletions, respectively (Fig. 5A). All the three knockout lines were substantially dwarfed, exhibited early flowering and defected floral organs (Fig. 5B to F). Besides, the leaves of the knockout lines were not lobulated and became round (Fig. 5G). These data suggested that the ClPID protein is involved in leaf morphogenesis and the development of lateral organs in watermelon.
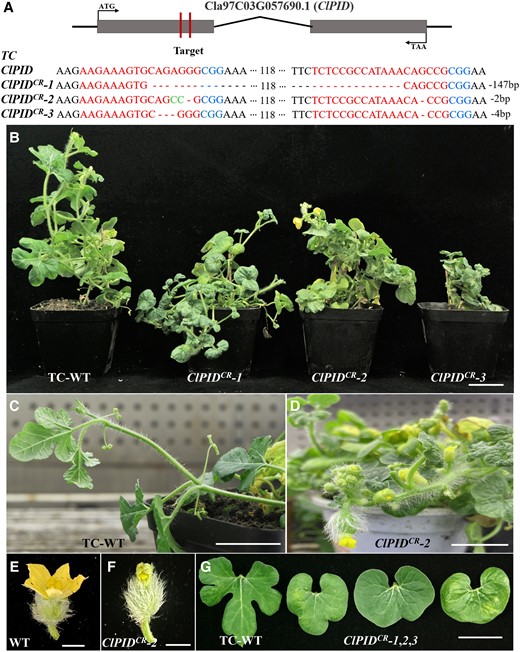
Phenotypic characterization of the ClPID knockout lines of watermelon. A) Schematic representation of the ClPID gene of watermelon depicting the two CRISPR/Cas9 target sites. The sgRNA targeted the first exon of ClPID, which resulted in a deletion mutation in ClPID.B) The ClPIDCR-1, ClPIDCR-2, and ClPIDCR-3 knockout lines displayed a dwarfed phenotype. Scale bar = 5 cm. C and D) The ClPIDCR-2 plant (D) exhibited early flowering compared with WT plants (C). Scale bars = 5 cm. E and F) The flower of ClPIDCR-2 plant (F) showed defects in sepals and petals compared with that of WT plant (E). Scale bars = 1 cm. G)ClPID knockout resulted in the formation of round leaves without lobulation in watermelon. Scale bar = 5 cm.
Knockout of ClAP1 inhibits floral initiation
The AP1 gene encodes a MADS-box family transcription factor, and acts as a floral meristem identity gene (Mandel et al. 1992). To verify the function of ClAP1 in watermelon, two target sites near the N-terminal of its first exon were selected for the construction of the CRISPR/Cas9 gene editing vectors (Fig. 6A). The results of DNA sequencing in the ClAP1CR-1, ClAP1CR-2, and ClAP1CR-3 knockout lines revealed that they had 1 bp, 3 bp (encoding an amino acid in the MADS-box domain of AP1), and 5 bp deletions, respectively (Fig. 6A, Supplemental Fig. S5). Phenotypic observations revealed that the formation of flowers was completely inhibited in all the three knockout lines throughout their whole growth cycle (Fig. 6B, Supplemental Fig. S6). The results demonstrated that ClAP1 also regulated the initiation of flowers, which act as a kind of lateral organ in watermelon.
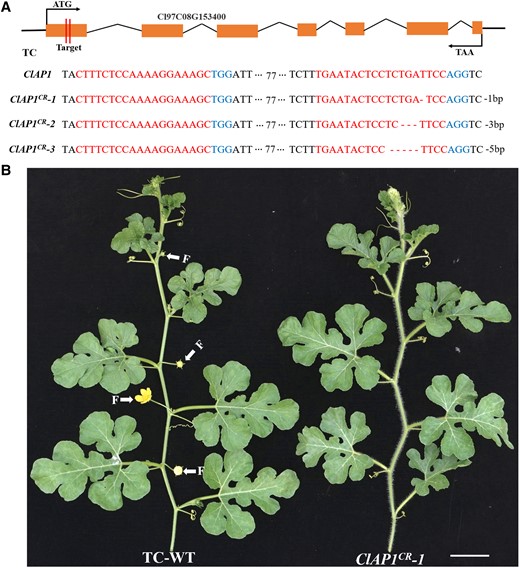
ClAP1 knockout inhibited the formation of male and female flowers in watermelon. A) Schematic representation of the ClAP1 gene of watermelon depicting the two CRISPR/Cas9 target sites. The orange boxes represent exons, while the black lines represent introns. The partial DNA sequences depict the deletions in the first exon. B)ClAP1 acts as a floral identity gene. Unlike WT plants, the ClAP1 knockout lines lacked male and female flowers in the leaf axils. Scale bars = 5 cm.
Discussion
ClLs acts as a lateral organ identity gene in watermelon
The Ls/LAS/MOC1 genes, encoding GRAS family transcription factors, have been characterized as key regulatory factors in shoot branching or tillering in monocots and dicots (Schumacher et al. 1999; Greb et al. 2003; Li et al. 2003; Ponraj and Theres 2020; Feng et al. 2021). The loss-of-function of ClLs in watermelon (C. lanatus) led to absence of all the lateral organs in the leaf axils, and resulted in few branches at maturity, which were inconsistent with the observations for putatively orthologous genes in related species (Fig. 1). The leaves of watermelon plants have distinct lobes, which are distinct from those of other plants in the Cucurbitaceae family, including cucumber (C. sativus), melon (C. melo), and pumpkin (Cucurbita moschata). However, the results of phenotypic observations revealed that the leaves of ClLs knockout lines lacked lobes and had smooth round margins, which have not been previously observed in reported watermelon mutants (Fig. 1). These functional differences between ClLs of watermelon and its putatively orthologous in other species could be attributed to various reasons, as described hereafter. Firstly, the expression pattern of ClLs was considerably different from that of its putatively orthologous in other species in that the ClLs mRNAs of watermelon accumulated in the leaf primordia and lateral organ meristems (Fig. 3). Secondly, all the lateral organs of watermelon originated from the AM in the axils of leaves. ClLs is an AM identity gene; its product could be involved in the initiation of AM and possibly controls the initiation of all watermelon lateral organs. Thirdly, previous studies have demonstrated that the tendrils of cucumber and melon evolve from branches, which could also occur in watermelon. Therefore, the formation of tendrils in watermelon could also be controlled by ClLs. Similarly, the loss-of-function of the Ls protein in tomato (S. lycopersicum) results in the formation of flowers with abnormal morphology, while the protein putatively orthologous to the Ls protein of tomato appeared to play a more potential role in floral initiation in watermelon.
ClLs directly interacts with the ClTEN tendril identity gene, the ClAP1 floral identity gene, and the ClPID leaf morphogenesis gene
The architecture of climbing vine is established by the iterative formation of lateral organs, which is regulated by several proteins with specific functions (Wang and Li 2008). The regulatory proteins in the ABC model function in a coordinated manner to determine the identity of floral organs (Coen and Meyerowitz 1991; Causier et al. 2010). Tendrils are characteristic lateral organs in plants belonging to the Cucurbitaceae family, and are regulated by the TCP family of transcription factors in cucumber and melon (Mizuno et al. 2015; Wang et al. 2015). However, the functional characteristics of only a few proteins involved in the development of lateral organs in watermelon have been studied to date. In this study, we used the CRISPR/Cas9 technology to knock out the ClTEN, ClAP1, and ClPID genes in watermelon, and the resulting mutants displayed a lack of tendrils, floral diapause, and round leaves, respectively (Figs. 4 to 6). Interestingly, all these phenotypes were observed in the ClLs knockout lines, while the transcription levels of ClLs in ClTEN knockout lines, ClPID knockout lines, and ClAP1 knockout lines were significantly decreased (Fig. 1, Supplemental Fig. S7). The results of in situ hybridization assays revealed that the mRNA signals of ClTEN, ClAP1, and ClPID overlapped with that of ClLs in the corresponding tissues (Fig. 3). A series of biochemical experiments further demonstrated that ClLs could directly interact with ClTEN, ClAP1, and ClPID in vivo and in vitro (Fig. 2), suggesting that ClLs functions by forming complexes with other proteins. These findings confirmed that ClLs regulated lateral organ initiation and leaf morphogenesis in watermelon, instead of only controlling branch formation. The PID protein of Arabidopsis is known to regulate auxin transport by phosphorylating PIN proteins (Benjamins et al. 2001); therefore, further studies are necessary for determining whether ClPID can phosphorylate ClLs in watermelon.
In summary, our results show that ClLs underwent functional expansion in watermelon and regulated the identity and morphogenesis of all lateral organs, including branches, tendrils, flowers, and leaves. The manner in which ClLs performs its functions is mainly driven by the following: (1) ClLs directly interacts with ClTEN to regulate tendril initiation and branch morphogenesis; (2) ClLs modulates leaf morphogenesis by forming a complex with ClPID; and (3) ClLs as a partner together with ClAP1 controls flower formation (Fig. 7).
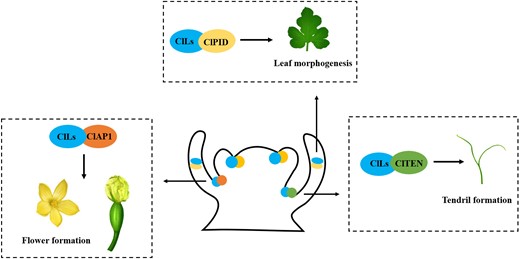
Working model of ClLs regulation of plant architecture in watermelon. ClLs co-expresses with ClTEN, ClPID, and ClAP1 in axillary meristems and leaf primordia. Besides, ClLs modulates the initiation and morphogenesis of tendril through interacting with ClTEN, regulate leaf morphogenesis by forming complex with ClPID, and together with ClAP1 controls flower formation.
Materials and methods
Plant materials and growth conditions
The “YL” and “TC” high generation inbred lines of watermelon (C. lanatus) were collected from the city of Yulin and Tongchuan, Shaanxi Province, China, respectively, and were used for genetic transformation and analysis of gene expression. The seeds were soaked in sterile water at a temperature of 55 °C for 30 min, and subsequently placed on moist gauze in a dark incubator at 28 °C until the seeds germinated. The germinating seeds with radicles were transferred to seedling raising pots in a growth chamber at 25 °C during the day and 20 °C at night under a 16 h/8 h light/dark photoperiod. The seedlings were transplanted to the solar greenhouse at the 2 to 3 true leaf stage. The management of fertilization and pest control were performed according to standard practices. Nicotiana benthamiana was cultivated in a growth chamber at 26 °C under a 16 h/8 h light/dark cycle. The leaves collected from plants aged 4 to 5 weeks were used for transient transformation.
Phylogenetic analysis
The GRAS family genes of watermelon and Arabidopsis were obtained from the NCBI data bases (https://www.ncbi.nlm.nih.gov/). Full-length protein sequences of GRAS family members were aligned using ClustalW in MEGA X, and the phylogenetic tree was constructed using the neighbor-joining (NJ) method with 1,000 bootstrap values.
Plasmid construction for CRISPR assay
Two sgRNAs were designed for targeting the genes using the CRISPR-P web server (http://cbi.hzau.edu.cn/cgi-bin/CRISPR), to construct the ClLs-CRISPR, ClPID-CRISPR, ClTEN-CRISPR, and ClAP1-CRISPR vectors. The sgRNA-U6-26t-U6-29p-sgRNA cassettes were amplified using pCBC-DT1T2 as the template and were subsequently inserted into the pBSE402 binary vector via a Bsa I (NEB, USA) restriction site. The primers used for generating the constructs are shown in Supplemental Table S2.
Stable transformation of watermelon
The watermelon cotyledons that were used as explants were transformed using the previously described A. tumefaciens mediated method (Tian et al. 2017; Cao et al. 2022). The plump seeds were disinfected with a solution of 70% v/v ethanol and 6% v/v sodium hypochlorite, sown on 0.64% agar powder plates, and cultured at 28 °C for 48 h. The cotyledons without embryos were cut into small pieces and subsequently infected with the GV3101 strain of A. tumefaciens which carried the pBSE402 CRISPR/Cas9 vector. The infected tissues were cultured in co-cultivation medium at 28 °C in the dark for 3 to 4 days. The explants were transferred into dedifferentiation medium containing 1.5 mg/L 6-BA (6-benzylaminopurinehydrochloride). The adventitious buds with positive GFP fluorescence were selected after 10 to 15 days and cultured in selective elongation medium supplemented with 0.01 mg/L NAA (1-naphthaleneacetic acid) and 0.1 mg/L 6-BA. The genomic DNA was extracted from the fresh leaves of the plants that exhibited positive fluorescence and WT plants using the CTAB method (Pahlich and Gerlitz 1980). The fragments of genomic DNA with two target sites were amplified and cloned into the TA vector for genotypic sequencing. The primers are showed in Supplemental Table S2.
In situ hybridization
The in situ hybridization assay was performed as previously described (Zhang et al. 2013). The gene-specific cDNA fragments of ClLs, ClTEN, ClAP1, and ClPID were generated by polymerase chain reaction (PCR). The fragments were prepared prior to constructing the sense and antisense RNA probes containing the SP6 and T7 promoters with a DIG RNA Labeling Kit (Roche, Germany). The shoot tips of 30-day-old watermelon seedlings were fixed in 3.7% (w/v) formaldehyde–acetic acid–ethanol fixing solution, dehydrated in different concentration gradients of alcohol (50%, 70%, 85%, 95%, and 100%), fixed with xylene, and infiltrated with paraffin wax. The prepared tissues were sliced into 8 μm-thick sections, and subjected to the hybridization assay. The primers used for this assay are showed in Supplemental Table S2.
Yeast two-hybrid assay
The full-length coding sequences (CDSs) of ClLs, ClTEN, ClAP1, and ClPID were cloned for constructing the pGBKT7 bait vector and the pGADT7 prey vector for the yeast two-hybrid assay. The primers are shown in Supplemental Table S2. After verifying self-activation, the bait and prey plasmids were co-transformed into the Y2HGold strain of yeast and cultured at 30 °C for 2 to 4 days on SD/-Trp-Leu plates. Selected monoclonal yeast transformants were cultured on SD/-Trp-Leu-His-Ade/X-α-gal plates for 2 to 4 days to detect the protein–protein interactions. The occurrence of normal growth and the blue coloration of the colonies revealed the possibility of interaction between two proteins.
LCI assay
For the LCI assay, the full-length CDS of ClLs was cloned into the pCAMBIA1300-cLUC vector, and the full-length CDSs without the stop codon of ClTEN, ClAP1, and ClPID were cloned into the pCAMBIA1300-nLUC vector via the Kpn I and Sal I sites. The primers are shown in Supplemental Table S2. The constructed plasmids and a P19 helper plasmid (containing a gene-silencing suppressor) were transformed into the GV3101 strain of A. tumefaciens and subsequently co-infiltrated into the leaves of N. benthamiana as previously described (Voinnet et al. 2003; Lasierra and Prat 2018). After 48 h of incubation, the abaxial surfaces of the leaves were sprayed with 1 mm luciferin (AAT Bioquest, USA) in the dark for 5 to 10 min and observed under a low-light cooled CCD imaging apparatus (Lumazone Pylon 2048B, USA).
BiFC assay
The full-length CDSs of ClLs, ClTEN, ClPID, and ClAP1 without stop codons were amplified and ligated into the pSPYNE-35S and pSPYCE-35S vectors containing the N- or C-terminal half of yellow fluorescent protein (YFP). The constructs were introduced into the GV3101 strain of A. tumefaciens and co-expressed in N. benthamiana. The infiltration point was marked, and the plants were cultured for 2 to 3 days. The fluorescence was observed under an Olympus BX63 fluorescence microscope (Japan), with experimental setup (laser, 488 nm; intensity, 2%; collection bandwidth, 479 to 542 nm; and gain, 400).
The primers used for the assay are showed in Supplemental Table S2.
Protein purification
The full-length CDS of ClLs was cloned and inserted into the EcoR I and Sal I sites of the pGEX-4T-1 plasmid to generate pGEX-GST-ClLs construct. The CDSs of ClAP1 and ClPID were cloned into the Xba I and Sal I sites of the pMAL-c2x plasmid for generating the pMAL-MBP-ClAP1 and pMAL-MBP-ClPID constructs, and the full-length CDS of ClTEN was cloned via the Nde Ⅰ and Xho Ⅰ sites of the pET-30a vector to generate the pET-30a-HIS-ClTEN vector construct. The primers used for vector construction are shown in Supplemental Table S2. The ClLs-GST fusion protein was obtained by culturing Escherichia coli (BL21) cells for 18 h at 16 °C after induction with 0.3 mm IPTG. The pMAL-MBP-ClAP1, pMAL-MBP-ClPID, and pET-30a-HIS-ClTEN plasmids were separately transformed into E. coli Rosetta (DE3) cells, and cultured for 18 h in liquid medium at 16 °C to produce the fusion protein after induction with 0.5 mm IPTG. The GST-tagged proteins were purified with glutathione (Smart-lifesciences, China), while the 6 × His-tagged and MBP-tagged proteins were purified using Ni-NTA agarose beads and dextrin beads (Smart-lifesciences, China).
Pull-down assay
For the GST pull-down assay, 1 mg each of the GST-tagged or GST-tagged fusion proteins were bound to 20 μL of glutathione sepharose resin (Smart-lifesciences, China) for 2 h at 4 °C on a rotator, followed by the addition of 1 mg each of 6 × His-tagged or MBP-tagged fusion proteins, and subsequent incubation at 4 °C for 2 h. The proteins bound to the beads were washed with 0.1% v/v NP-40 in PBS and subsequently eluted with elution buffer (PBS, pH 7.3, 10 mm glutathione). The pull-down samples were resolved by SDS-PAGE using 10% gels, and subsequently detected by immunoblotting assays with antibodies against GST, MBP, and HIS (Beyotime, China; dilution 1:1,000). The GST beads alone were used in the control setup.
Protein sequence alignment
The full-length amino acid sequence of APETALA 1 (AtAP1) was obtained from Arabidopsis genome database (https://www.arabi-dopsis.org). To identify the AP1 homologous genes in tomato, tobacco, rice, cucumber, and watermelon, TBLASTN searches were performed in the NCBI data bases (https://www.ncbi.nlm.nih.gov/) using the AtAP1 protein sequence as a reference. All deduced amino acid sequences were analyzed using the NCBI Conserved Domain Database (https://www.ncbi.nlm.nih.gov/Structure/cdd/wrpsb.cgi) to detect conserved motifs among the proteins.
Reverse transcription quantitative PCR analysis
Total RNA was extracted from apical buds of WT plants and mutants using TRIzol reagent (Vazyme, China). First-strand cDNA was synthesized using a HiScript II 1st Strand cDNA Synthesis Kit (Vazyme). Reverse transcription quantitative PCR was performed to determine the expression levels of genes using the SYBR qPCR Master Mix (Vazyme) on an ABI 7500 Fast Real-Time PCR System (Applied Biosystems, USA). Three biological replicates and three technical replicates were performed for each gene. The watermelon ClACTIN gene was used as the internal control in the analysis (Kong et al. 2014). The relative expression levels were analyzed using the comparative 2−ΔΔCT method.
Statistical analysis
All statistical analyses were performed using GraphPad Prism 8.3.0 (GraphPad Software, La Jolla, CA, USA). Data are shown as mean ± SD.
Accession numbers
Sequence data from this article can be found in the GenBank/EMBL data libraries under accession numbers listed in Supplemental Table S1.
Acknowledgments
We are grateful to Prof. Yong Xu and Prof. Changlong Weng (Vegetable Research Center of Beijing Academy of Agriculture and Forestry) for providing stabilized transformation technology of watermelon.
Author contributions
Z.L. and J.S. designed and supervised this study; Y.J., A.Z., W.H., Q.L., B.Z., H.Z., X.K., Y.G., P.S., T.Y., and Z.W. performed experiments and analyzed the data; Z.L., J.S., B.J., and Y.J. wrote and revised the paper, which was approved by all authors.
Supplemental data
The following materials are available in the online version of this article.
Supplemental Figure S1. Longitudinal section of the shoot apex showing the distribution of leaf primordia (LP).
Supplemental Figure S2. Phylogenetic tree of the GRAS family genes of watermelon and Arabidopsis was generated using the neighbor-joining (NJ) method with 1,000 bootstrap replicates.
Supplemental Figure S3. Phenotypic characterization of ClLs knockout lines in watermelon.
Supplemental Figure S4. Phenotypic characterization of the ClTEN knockout lines.
Supplemental Figure S5. Amino acid sequence alignment of AP1 homologs in different species.
Supplemental Figure S6.ClAP1 knockout repressed floral initiation in watermelon.
Supplemental Figure S7. The expression levels of ClLs decreased in ClTEN knockout lines, ClPID knockout lines, and ClAP1 knockout lines.
Supplemental Table S1. Gene information used in this study.
Supplemental Table S2. The primers used in this study.
Funding
This work was supported by the National Key Research and Development Program of China (grant number 2022YFD1602000), the National Natural Science Foundation of China (32202519, U22A20498, and 32072596), the Key Research and Development Program of Shaanxi (2023-YBNY-011), and the Science and Technology Innovation Team of Shaanxi (2021TD-32).
Data availability
All relevant data are included in the article and Supplemental data.
Dive Curated Terms
The following phenotypic, genotypic, and functional terms are of significance to the work described in this paper:
References
Author notes
The author responsible for distribution of materials integral to the findings presented in the article in accordance with the policy described in the Instructions for Authors (https://dbpia.nl.go.kr/plphys/pages/general-instructions) is: Zheng Li ([email protected]).
Conflict of interest statement. None declared.