-
PDF
- Split View
-
Views
-
Cite
Cite
Zhi-Xiong Xu, Xin-Meng Zhu, Huachun Yin, Bo Li, Xiao-Jie Chen, Xu-Li Fan, Neng-Qi Li, Marc-André Selosse, Jiang-Yun Gao, Jia-Jia Han, Symbiosis between Dendrobium catenatum protocorms and Serendipita indica involves the plant hypoxia response pathway, Plant Physiology, Volume 192, Issue 3, July 2023, Pages 2554–2568, https://doi.org/10.1093/plphys/kiad198
- Share Icon Share
Abstract
Mycorrhizae are ubiquitous symbioses established between fungi and plant roots. Orchids, in particular, require compatible mycorrhizal fungi for seed germination and protocorm development. Unlike arbuscular mycorrhizal fungi, which have wide host ranges, orchid mycorrhizal fungi are often highly specific to their host orchids. However, the molecular mechanism of orchid mycorrhizal symbiosis is largely unknown compared to that of arbuscular mycorrhizal and rhizobial symbiosis. Here, we report that an endophytic Sebacinales fungus, Serendipita indica, promotes seed germination and the development of protocorms into plantlets in several epiphytic Epidendroideae orchid species (6 species in 2 genera), including Dendrobium catenatum, a critically endangered orchid with high medicinal value. Although plant–pathogen interaction and high meristematic activity can induce the hypoxic response in plants, it has been unclear whether interactions with beneficial fungi, especially mycorrhizal ones, also involve the hypoxic response. By studying the symbiotic relationship between D. catenatum and S. indica, we determined that hypoxia-responsive genes, such as those encoding alcohol dehydrogenase (ADH), are highly induced in symbiotic D. catenatum protocorms. In situ hybridization assay indicated that the ADH gene is predominantly expressed in the basal mycorrhizal region of symbiotic protocorms. Additionally, the ADH inhibitors puerarin and 4-methylpyrazole both decreased S. indica colonization in D. catenatum protocorms. Thus, our study reveals that S. indica is widely compatible with orchids and that ADH and its related hypoxia-responsive pathway are involved in establishing successful symbiotic relationships in germinating orchids.
Introduction
In plants, mycorrhizal symbiosis is extremely important, not only in spontaneous ecosystems but also in agriculture (van der Heijden et al. 2015). In a typical mycorrhizal symbiosis, fungi growing within (endomycorrhiza) or on (ectomycorrhiza) plant roots acquire mineral nutrients from the surrounding soil for the host plants (Wang et al. 2017). The host plants, in turn, provide the fungi with photosynthetic products (organic carbon such as sugars and lipids) (Wang et al. 2017; Genre et al. 2020). More than 90% of vascular plants are mycorrhizal (Brundrett and Tedersoo 2018), among which orchid mycorrhizae (OrM) represent a special case.
Orchidaceae is one of the largest plant families, with more than 27,000 species (Dearnaley and Cameron 2017). Orchid seeds are dust-like, with a simple embryo and minimal nutrient reserves. During seed germination, the embryo first develops into a protocorm before forming a plantlet (Dearnaley et al. 2016; Yeung 2017). Orchid protocorms are small, spherical, undifferentiated tuber-like bodies with a meristematic region (Fang et al. 2016; Yeung 2017). OrM fungi have high host specificity when compared with arbuscular mycorrhiza (AM) fungi (van der Heijden et al. 2015; Brundrett and Tedersoo 2018). This has hampered orchid conservation efforts, as it is difficult to obtain compatible fungi. Noncompatible fungi may stimulate orchid seed germination, but they do not support plantlet development (Rasmussen et al. 2015; Huang et al. 2018; Meng et al. 2019b; Favre-Godal et al. 2020).
Orchids generally form mycorrhiza with saprotrophic fungi, usually members of Cantharellales (Tulasnellaceae and Ceratobasidiaceae) or Sebacinales among basidiomycetes (Dearnaley et al. 2012). Serendipita indica (syn. Piriformospora indica), a fungus from the Serendipitaceae (Sebacinales; Weiss et al. 2016), has been well-studied as a root-colonizing fungus that confers diverse beneficial effects on a broad range of host plants (Zuccaro et al. 2011; Qiang et al. 2012). In nonorchid plants like Arabidopsis thaliana, S. indica usually does not form mycorrhizae but establishes a loose, undifferentiated colonization in the roots called endophytism (Qiang et al. 2012; Selosse et al. 2022), as do many Serendipitaceae (Selosse et al. 2009). Molecular identification has been used to detect its sequence on the roots of Microtis media, an Australian terrestrial orchid (De Long et al. 2013), and Hoffmannseggella cinnabarina, a Brazilian epilithic orchid (Oliveira et al. 2014), but no visual confirmation has verified that the fungus was truly OrM in these adult plants. Moreover, it is unclear whether S. indica can colonize the seed or protocorm and promote seed germination and protocorm development in orchids. Here, we tested the potential of S. indica for wide application in orchid conservation and breeding and determined that S. indica is compatible with the seed and protocorm of several epiphytic orchids.
Symbiosis at the protocorm stage is an intriguing model in which to study the biological interactions between plants and fungi because of the high dependency of the protocorms on their fungus partners for survival and growth (Favre-Godal et al. 2020). However, OrM symbiosis remains little understood compared to those of AM or nitrogen-fixing Rhizobium on legumes (Zipfel and Oldroyd 2017; Genre et al. 2020), despite a few pioneering studies (e.g. Valadares et al. 2014; Fochi et al. 2017; Jakalski et al. 2021). The establishment of a symbiotic relationship between a legume plant and Rhizobium bacteria is characterized by the formation of nodules, which create a low-oxygen environment that allows the fixation of atmospheric nitrogen (Soupene et al. 1995; Berger et al. 2019). Hypoxia-responsive genes are involved in the interaction between plants and pathogens (fungi, bacteria, and protists), and they are highly upregulated upon invasion of these pathogens (Gravot et al. 2016; Kerpen et al. 2019; Chung and Lee 2020; Valeri et al. 2021; Wang et al. 2021b). However, it is unclear if the interactions with beneficial fungi, especially mycorrhizal ones, involve the hypoxia response in plants.
Hypoxia (low oxygen) conditions in meristem niches are a developmental regulator in plants and influence the rates of leaf and lateral root formation (Le Gac and Laux 2019; Weits et al. 2019; Xia et al. 2022). Recent studies have shown that hypoxia-responsive genes are highly induced in rapidly proliferating cell types, even when the cells are in an aerobic environment (DeBerardinis and Chandel 2016; Le Gac and Laux 2019; Weits et al. 2021; Xia et al. 2022). The meristematic regions of symbiotic protocorms, like the meristem of other plants, comprise rapidly proliferating cell types (Yeung 2017). During the hypoxic response in plants, such as growth under submerged or waterlogged conditions, glycolysis metabolism and alcoholic fermentation are simultaneously upregulated (Bailey-Serres et al. 2012; Ventura et al. 2020). Alcohol dehydrogenase (ADH) and pyruvate decarboxylase (PDC) are key enzymes for alcoholic fermentation in most plants, and the expression of their encoding genes is induced by plant-specific group VII ethylene response factors under hypoxia conditions (Bailey-Serres et al. 2012; Licausi 2013; Ventura et al. 2020). ADH reduction activity, which catalyzes the conversion of NADH to NAD+ during alcoholic fermentation, maintains glycolysis metabolism during hypoxic stress (Bailey-Serres et al. 2012). Plant cysteine oxidases (PCOs) control oxygen-dependent protein degradation in plants under normoxic (normal oxygen) conditions and act as oxygen sensors (Weits et al. 2014). Specific PCO genes are highly induced by intracellular oxygen deficiency (Weits et al. 2014). Generally, hypoxia levels in plant tissues are indirectly monitored by detecting the upregulation of hypoxia-responsive genes (ADH, PDC, PCO, etc.) (Ventura et al. 2020; Valeri et al. 2021).
Here, we investigated the gene expression profiling of the protocorm of the orchid Dendrobium catenatum just after a stable mycorrhizal symbiosis had been established, finding that key hypoxia-responsive genes, especially ADH, are highly induced in the symbiotic protocorm. An in situ hybridization experiment demonstrated that ADH is predominantly expressed in the basal mycorrhizal region of the symbiotic protocorm. In vitro and in vivo assays further showed that D. catenatum ADHs have typical ADH activity, and this ADH activity positively regulates the establishment of a symbiotic relationship between D. catenatum protocorms and S. indica. In summary, our results suggest that ADHs and the associated hypoxia-responsive pathway are involved in the interaction between orchid protocorms and beneficial OrM fungi.
Results
S. indica is compatible with D. catenatum and many other orchids
In Southeast Asia and China, many Dendrobium orchids used in traditional medicine have been excessively collected and are currently endangered (Xiang et al. 2013; Cheng et al. 2019; Jo et al. 2022). We collected the seeds of numerous Epidendroideae orchids distributed or cultured in south China, mainly Dendrobium species, and analyzed the effects of the endophytic fungus S. indica on seed germination and the growth of orchid protocorms into plantlets in 12 ecologically and taxonomically diverse orchid species from this collection (Table 1). Orchid protocorms, formed after seed germination, are small, spherical, tuber-like bodies with 1 meristematic region at the anterior end where new leaves and roots form sequentially (Favre-Godal et al. 2020). We found that S. indica promoted growth in 6 of the 12 orchid species: D. catenatum (syn. Dendrobium officinale), Dendrobium nobile, Dendrobium wangliangii, Dendrobium chrysotoxum, Cymbidium aloifolium, and Cymbidium mannii (Fig. 1, A and B; Supplemental Fig. S1A). Although a phylogenetic tree based on rDNA internal transcribed spacer sequences showed the 6 compatible orchids were not clustered together, all 6 species are epiphytic orchids (Table 1; Supplemental Fig. S1B). S. indica not only stimulated seed germination in these species but also promoted protocorm development into plantlets (Fig. 1, A and B; Supplemental Fig. S1A). The growth promotion effect of S. indica was high for D. catenatum but low for C. mannii (Fig. 1B). Next, we analyzed S. indica colonization in protocorm cells via semi-thin tissue sections and toluidine blue O (TBO) staining. The intracellular mycelium was located at the basal regions of the orchid protocorms (Fig. 1C), the site of future root development. Intracellular hyphal coils (pelotons; Fig. 1C) in the protocorms were morphologically consistent with those of other OrM (Perotto et al. 2014; Fochi et al. 2017), showing both living pelotons, with easily recognizable hyphae, and old and lysed pelotons (sometimes in the same cell) undergoing final degradation. Therefore, our results showed that S. indica forms a typical OrM association with some orchids and promotes seed germination and protocorm development into plantlets.
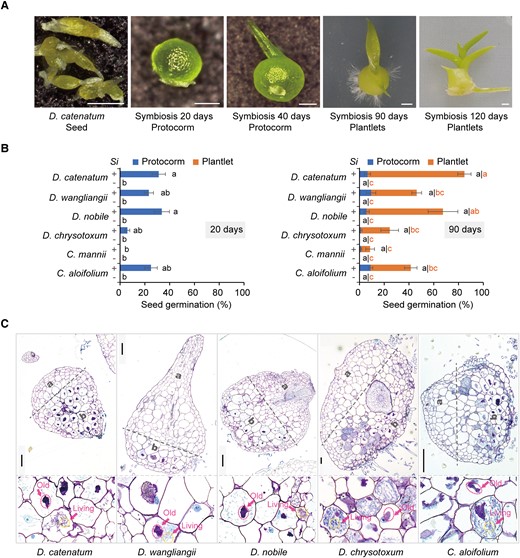
S. indica is compatible with many orchid species A) D. catenatum seeds and their symbiotic protocorms or plantlets after inoculation with S. indica. Scale bars: 250 μm in left three images, 1 mm in right two images. B) S. indica (Si) promotes seed germination and protocorm development in six different orchid species: D. catenatum, D. wangliangii, D. nobile, D. chrysotoxum, C. mannii, and C. aloifolium. The seeds germinated in the presence of S. indica formed protocorms by about 20 days after inoculation with S. indica, with most protocorms developing into plantlets by 90 days, whereas no protocorms were formed from aseptically cultured seeds (controls, −). Data are mean ± SE, n ≥ 3 (10 plates on average for each value). Different letters next to error bars indicate statistically significant differences at P ≤ 0.05 (ANOVA with Tukey HSD post hoc test). Images are shown in Supplemental Figure S1. C) S. indica colonizes protocorm cells as an orchid mycorrhizal fungus. Sections of resin-embedded protocorms show the apical, uninfected region (a) and basal, mycorrhizal region (b) of orchid protocorms, with details of colonized cells at the bottom. Circles indicate lysed intracellular fungal pelotons (old pelotons), and living pelotons in the enlarged images. Scale bars, 100 μm.
Orchid species used in the symbiotic growth experiment with S. indica. Bold indicates species compatible with S. indica
. | Orchid species . | Tribe . | Life form . | Seed vigor (%) . | Expected mycorrhizal fungi . | Compatibility with S. indica . |
---|---|---|---|---|---|---|
1 | D. catenatum | Epidendroideae Dendrobieae | Epiphytic | 84 | Sebacinales and Tulasnellaceae (Wang et al. 2021a) | Yes |
2 | D. nobile | Epidendroideae Dendrobieae | Epiphytic | 11 | Sebacinales (Wang et al. 2011) | Yes |
3 | Dendrobium aphyllum | Epidendroideae Dendrobieae | Epiphytic | 95 | Tulasnellaceae (Zi et al. 2014) | No |
4 | D. wangliangii | Epidendroideae Dendrobieae | Epiphytic | 97 | Unknown | Yes |
5 | Dendrobium chrysanthum | Epidendroideae Dendrobieae | Epiphytic | 42 | Unknown | No |
6 | D. chrysotoxum | Epidendroideae Dendrobieae | Epiphytic | 92 | Sebacinales and Tulasnellaceae (Shao et al. 2020) | Yes |
7 | C. mannii | Epidendroideae Cymbidieae | Epiphytic | 87 | Tulasnellaceae (Chun-Ling et al. 2012) | Yes |
8 | C. aloifolium | Epidendroideae Cymbidieae | Epiphytic | 93 | Sebacinales (Shah et al. 2019) | Yes |
9 | Acampe rigida | Epidendroideae Vandoideae | Epiphytic | 95 | Unknown | No |
10 | Cymbidium sinense | Epidendroideae Cymbidieae | Terrestrial | 25 | Unknown | No |
11 | Geodorum densiflorum | Epidendroideae Cymbidieae | Terrestrial | 40 | Unknown | No |
12 | Calanthe triplicata | Epidendroideae Collabiinae | Terrestrial | 8 | Unknown | No |
. | Orchid species . | Tribe . | Life form . | Seed vigor (%) . | Expected mycorrhizal fungi . | Compatibility with S. indica . |
---|---|---|---|---|---|---|
1 | D. catenatum | Epidendroideae Dendrobieae | Epiphytic | 84 | Sebacinales and Tulasnellaceae (Wang et al. 2021a) | Yes |
2 | D. nobile | Epidendroideae Dendrobieae | Epiphytic | 11 | Sebacinales (Wang et al. 2011) | Yes |
3 | Dendrobium aphyllum | Epidendroideae Dendrobieae | Epiphytic | 95 | Tulasnellaceae (Zi et al. 2014) | No |
4 | D. wangliangii | Epidendroideae Dendrobieae | Epiphytic | 97 | Unknown | Yes |
5 | Dendrobium chrysanthum | Epidendroideae Dendrobieae | Epiphytic | 42 | Unknown | No |
6 | D. chrysotoxum | Epidendroideae Dendrobieae | Epiphytic | 92 | Sebacinales and Tulasnellaceae (Shao et al. 2020) | Yes |
7 | C. mannii | Epidendroideae Cymbidieae | Epiphytic | 87 | Tulasnellaceae (Chun-Ling et al. 2012) | Yes |
8 | C. aloifolium | Epidendroideae Cymbidieae | Epiphytic | 93 | Sebacinales (Shah et al. 2019) | Yes |
9 | Acampe rigida | Epidendroideae Vandoideae | Epiphytic | 95 | Unknown | No |
10 | Cymbidium sinense | Epidendroideae Cymbidieae | Terrestrial | 25 | Unknown | No |
11 | Geodorum densiflorum | Epidendroideae Cymbidieae | Terrestrial | 40 | Unknown | No |
12 | Calanthe triplicata | Epidendroideae Collabiinae | Terrestrial | 8 | Unknown | No |
Orchid species used in the symbiotic growth experiment with S. indica. Bold indicates species compatible with S. indica
. | Orchid species . | Tribe . | Life form . | Seed vigor (%) . | Expected mycorrhizal fungi . | Compatibility with S. indica . |
---|---|---|---|---|---|---|
1 | D. catenatum | Epidendroideae Dendrobieae | Epiphytic | 84 | Sebacinales and Tulasnellaceae (Wang et al. 2021a) | Yes |
2 | D. nobile | Epidendroideae Dendrobieae | Epiphytic | 11 | Sebacinales (Wang et al. 2011) | Yes |
3 | Dendrobium aphyllum | Epidendroideae Dendrobieae | Epiphytic | 95 | Tulasnellaceae (Zi et al. 2014) | No |
4 | D. wangliangii | Epidendroideae Dendrobieae | Epiphytic | 97 | Unknown | Yes |
5 | Dendrobium chrysanthum | Epidendroideae Dendrobieae | Epiphytic | 42 | Unknown | No |
6 | D. chrysotoxum | Epidendroideae Dendrobieae | Epiphytic | 92 | Sebacinales and Tulasnellaceae (Shao et al. 2020) | Yes |
7 | C. mannii | Epidendroideae Cymbidieae | Epiphytic | 87 | Tulasnellaceae (Chun-Ling et al. 2012) | Yes |
8 | C. aloifolium | Epidendroideae Cymbidieae | Epiphytic | 93 | Sebacinales (Shah et al. 2019) | Yes |
9 | Acampe rigida | Epidendroideae Vandoideae | Epiphytic | 95 | Unknown | No |
10 | Cymbidium sinense | Epidendroideae Cymbidieae | Terrestrial | 25 | Unknown | No |
11 | Geodorum densiflorum | Epidendroideae Cymbidieae | Terrestrial | 40 | Unknown | No |
12 | Calanthe triplicata | Epidendroideae Collabiinae | Terrestrial | 8 | Unknown | No |
. | Orchid species . | Tribe . | Life form . | Seed vigor (%) . | Expected mycorrhizal fungi . | Compatibility with S. indica . |
---|---|---|---|---|---|---|
1 | D. catenatum | Epidendroideae Dendrobieae | Epiphytic | 84 | Sebacinales and Tulasnellaceae (Wang et al. 2021a) | Yes |
2 | D. nobile | Epidendroideae Dendrobieae | Epiphytic | 11 | Sebacinales (Wang et al. 2011) | Yes |
3 | Dendrobium aphyllum | Epidendroideae Dendrobieae | Epiphytic | 95 | Tulasnellaceae (Zi et al. 2014) | No |
4 | D. wangliangii | Epidendroideae Dendrobieae | Epiphytic | 97 | Unknown | Yes |
5 | Dendrobium chrysanthum | Epidendroideae Dendrobieae | Epiphytic | 42 | Unknown | No |
6 | D. chrysotoxum | Epidendroideae Dendrobieae | Epiphytic | 92 | Sebacinales and Tulasnellaceae (Shao et al. 2020) | Yes |
7 | C. mannii | Epidendroideae Cymbidieae | Epiphytic | 87 | Tulasnellaceae (Chun-Ling et al. 2012) | Yes |
8 | C. aloifolium | Epidendroideae Cymbidieae | Epiphytic | 93 | Sebacinales (Shah et al. 2019) | Yes |
9 | Acampe rigida | Epidendroideae Vandoideae | Epiphytic | 95 | Unknown | No |
10 | Cymbidium sinense | Epidendroideae Cymbidieae | Terrestrial | 25 | Unknown | No |
11 | Geodorum densiflorum | Epidendroideae Cymbidieae | Terrestrial | 40 | Unknown | No |
12 | Calanthe triplicata | Epidendroideae Collabiinae | Terrestrial | 8 | Unknown | No |
Among these orchids, D. catenatum is a critically endangered orchid with high medicinal value, and, more importantly, its whole genome is available (Zhang et al. 2016). Therefore, we studied the symbiotic mechanism between orchids and endophytic fungi as represented by the D. catenatum–S. indica symbiotic system.
Transcriptome profiles of symbiotic protocorms differ from those of aseptic protocorms
Orchid seeds are very small and establish symbiosis with fungi very early in seed germination (Dearnaley et al. 2016; Yeung 2017), so it is difficult to obtain sufficient biomass for transcriptome analysis when a stable mycorrhizal symbiosis has just been established. In previous transcriptome studies of orchid mycorrhizal symbiosis, the orchid plant materials used were those that had established a long-term stable symbiosis with OrM fungi (Zheng et al. 2018; Favre-Godal et al. 2020). These data do not accurately capture the gene expression profiles at the time when a stable mycorrhizal symbiosis has just been established. In this study, to obtain enough biomass for reverse transcription-quantitative PCR (RT-qPCR) and transcriptome analysis, we used 30-d-old D. catenatum protocorms that originated from aseptically germinated seeds as starting material. The 30-d-old protocorms were transplanted to symbiotic medium (OMA medium), cultured aseptically (control), or inoculated with S. indica (Si), and then collected for subsequent RT-qPCR or transcriptome analysis. RT-qPCR analysis of S. indica Translation elongation factor 1 (SiTef1) expression, normalized to that of D. catenatum Actin-7 (DcActin7), in the inoculated protocorms showed that the S. indica colonization level peaked on the 9th day after inoculation (Fig. 2A). Inspection of semi-thin sections further confirmed the presence of intracellular mycelium in the symbiotic protocorm at that time, located at the basal regions of the protocorms (Fig. 2B), which was consistent with our earlier observations of protocorms germinated in the presence of S. indica (Fig. 1C). Therefore, a stable mycorrhizal symbiosis was established between the protocorm and fungus by the 9th day after inoculation. Symbiotic protocorms at 9 d after inoculation were used for subsequent transcriptome analysis.
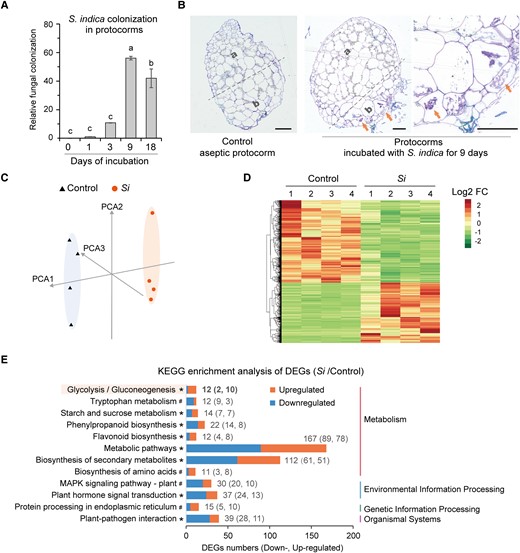
Transcriptome analysis of symbiotic protocorms. A) Relative colonization level of S. indica in symbiotic protocorms after different incubation times detected using RT-qPCR analysis of the expression of SiTef1 normalized to that of DcActin7. Different letters above error bars indicate statistically significant differences at P ≤ 0.05 (ANOVA with Tukey HSD post hoc test). B) Semi-thin sections showing the apical region (a) and basal region (b) of aseptic or symbiotic protocorms. Red arrows point to the intracellular fungal peloton. Scale bar, 100 μm. C) Principal component analysis (PCA) of D. catenatum transcriptome samples. Four biological duplications were conducted for each sample. D) Heat map showing expression patterns of the upregulated and downregulated genes in S. indica–inoculated protocorms compared to controls. The “Euclidean” distance and “complete” algorithm were used in the clustering analysis. E) KEGG enrichment analysis of differentially expressed genes (DEGs) between Si and control samples. There were 12 enriched KEGG pathways with at least 11 DEGs (*P < 0.1; #P ≥ 0.1). Supplemental Table S2 shows DEGs in each KEGG pathway. These enriched pathways are mainly related to plant metabolism and plant defense and immunity. In the glycolysis/gluconeogenesis metabolic pathway, the number of upregulated DEGs (Si divided by control) is much greater than that of downregulated DEGs (see Supplemental Figure S2 for details).
The transcriptome sequencing data were mapped to the D. catenatum genome, which has been reported previously (Zhang et al. 2016). Transcriptome analysis of D. catenatum showed distinct expression profiles for the aseptic and symbiotic protocorms (Fig. 2, C and D). We identified 356 differentially expressed genes (DEGs) between the 2 groups of protocorms (defined by an absolute value of log2(fold change) ≥ 1 and Q-value ≤ 0.05) and found that these DEGs were annotated in several crucial KEGG pathways (Fig. 2E; Supplemental Table S2). As expected, plant defense and immune response-related pathways were enriched, including plant hormone signal transduction, plant–pathogen interaction, and MAPK signaling pathway-plant. The DEGs were also enriched in several metabolic pathways (flavonoid biosynthesis, phenylpropanoid biosynthesis, and tryptophan metabolism) that have been shown to be involved in plant defense and immune responses (Hiruma et al. 2013; Fukunaga et al. 2017; Bajaj et al. 2018; Pastorczyk et al. 2020; Jiang et al. 2021; Wolinska et al. 2021). The numbers of upregulated and downregulated DEGs (Si divided by control) were similar in most enriched pathways (Fig. 2E). Considering that the colonization level of S. indica on the 18th day was lower than that on the 9th day (Fig. 2A), we suggest that, after the establishment of stable mycorrhizal symbiosis, the colonization of mycorrhizal fungi in host plants is limited and accompanied by reprogramming of defense and immune-related pathways.
In addition, basic metabolic pathways such as glycolysis were enriched in the KEGG analysis (Fig. 2E; Supplemental Table S2). Among the KEGG pathways, the number of upregulated DEGs (Si divided by control) in the glycolysis pathway was much higher than that of downregulated DEGs (Fig. 2E). Notably, 4 DcADH genes were upregulated in the glycolysis pathway (Supplemental Fig. S2). This is considerably more than the usual number of DEGs for a given gene code (1 or 2) and implies that ADHs of D. catenatum may play an important role in mycorrhizal symbiosis.
Hypoxia-responsive genes are induced in symbiotic protocorms
ADH is a key hypoxia-responsive gene, which, together with PDC, plays important roles in fermentative metabolism during low-oxygen responses in plants (Bailey-Serres et al. 2012; Ventura et al. 2020). Using homology analysis, we identified 12 homologous ADH genes in D. catenatum (Supplemental Fig. S3A). Transcriptome analysis and an RT-qPCR assay showed that almost all DcADH genes were upregulated in symbiotic protocorms, with the expression of DcADH1 and DcADH2 being the most highly induced (Fig. 3, A and C; Supplemental Fig. S3B). Consistent with this, biochemical assays showed that the reduction activity of ADH in symbiotic protocorms on the 9th day after inoculation was markedly higher than that in aseptic protocorms (Fig. 3, D and E). Additionally, phylogenetic analysis of their protein sequences revealed that DcADH1 and DcADH2 are clustered together (Supplemental Fig. S3A). These results suggest that DcADH1 and DcADH2 may be specifically associated with mycorrhizal symbiosis.
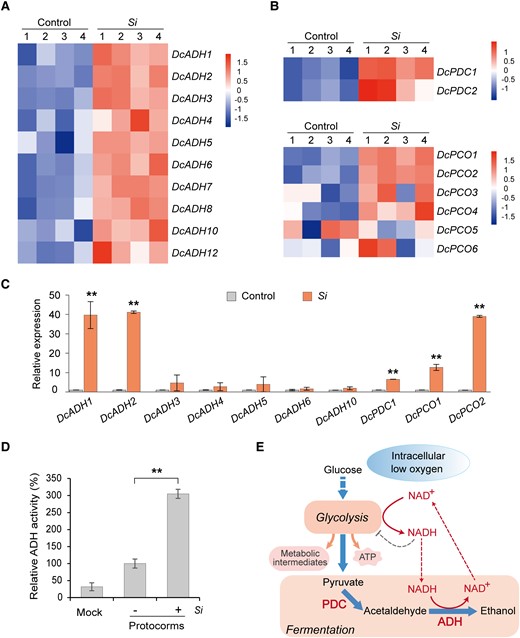
Hypoxia-responsive genes of D. catenatum are induced in symbiotic protocorms. A, B) Heat map analysis of the expression levels of DcADH, DcPDC, and DcPCO genes in D. catenatum. S. indica colonization upregulated these key hypoxia-responsive genes in symbiotic protocorms. C) The expression of these key hypoxia-responsive genes in D. catenatum symbiotic protocorms was analyzed using RT-qPCR. Si indicates protocorms inoculated with S. indica 9 days after inoculation. Control is the aseptic protocorms. The expression level of each gene in the control sample is set to 1. Data are mean ± SD, n = 4. Two asterisks at the top indicate highly significant differences between control and Si samples tested using Student's t-test, P < 0.01. D) ADH activity of symbiotic protocorms (+) is higher than that of aseptic protocorms (-). Mock refers to the protein extraction buffer. Data are mean ± SD, n = 4. Two asterisks indicate highly significant differences tested using Student's t-test, P < 0.01. E) Hypoxia metabolism is regulated by ADH and PDC in plants. Intracellular low oxygen (hypoxia) induces glycolysis pathways, which produce ATP and metabolic intermediates (for the synthesis of nucleic acids, proteins, and lipids) to sustain cell growth and survival. Glycolysis produces copious NADH, which in turn inhibits glycolysis. In plant cells, ADH catalyzes the conversion of NADH into NAD+ through the alcoholic fermentation pathway, thereby allowing glycolysis to proceed.
The transcriptome analysis and RT-qPCR also showed that DcPDC genes were markedly upregulated in symbiotic protocorms (Fig. 3, B and C). Also upregulated were DcPCO1 and DcPCO2 (Fig. 3, B and C), the D. catenatum homologs of Arabidopsis PCO1 and PCO2, which are specific hypoxia-inducible genes that act as oxygen sensors (Weits et al. 2014). Additionally, the expression of these hypoxia-responsive genes, including DcADH1 and DcADH2, was positively correlated with symbiosis at the temporal level (Supplemental Fig. S4). Therefore, these results showed that key hypoxia-responsive genes, especially DcADH genes, are induced by the colonization of S. indica in the symbiotic protocorms of D. catenatum.
ADH activity positively regulates mycorrhizal symbiosis in protocorms
To test whether the upregulation of DcADH1 or DcADH2 is responsible for increased ADH activity in symbiotic protocorms, we cloned the DcADH1 and DcADH2 coding sequences and expressed them in Escherichia coli (Supplemental Fig. S5). In vitro biochemical assays of protein extracts from the recombinant bacteria showed that DcADH1 and DcADH2 had the expected ADH activity, converting NADH to NAD+ (Figs. 3E and 4A). Then, we confirmed that the ADH inhibitors puerarin and 4-methylpyrazole both inhibited the NADH-converting activities of DcADH1 and DcADH2 in vitro (Fig. 4, B and C; Supplemental Fig. S6). Among them, DcADH2 is most highly expressed in the symbiotic protocorms (Supplemental Fig. S3). Thus, we performed in situ hybridization using a digoxigenin (DIG)-labeled oligonucleotide probe of DcADH2. This DcADH was predominantly expressed in the basal mycorrhizal region rather than the apical region in the symbiotic protocorm (Fig. 5, A and B), suggesting that DcADH expression in protocorms is positively correlated with symbiosis at the spatial level. Therefore, a key question is whether ADH activity regulates the symbiotic relationship between D. catenatum protocorms and S. indica.
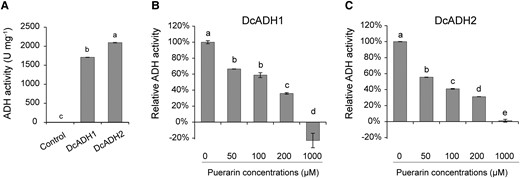
DcADH1 and DcADH2 show ADH activity. A) In vitro assays show that the purified recombinant proteins of DcADH1 and DcADH2 have ADH reduction activity to catalyze the conversion of NADH to NAD+. Control is the purified free GST protein. B, C) Inhibitory effects of different concentrations of puerarin on the ADH reduction activity of purified DcADH1 and DcADH2 in vitro. Data are mean ± Sd, n ≥ 4. Different letters indicate statistically significant differences at P ≤ 0.05 (ANOVA with Tukey HSD post hoc test).
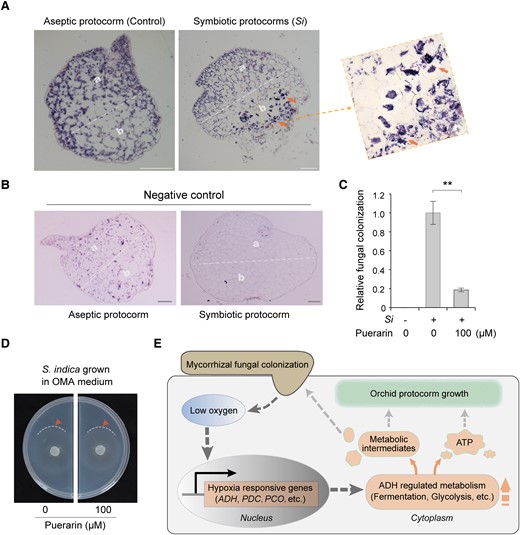
ADH activity is required for OrM fungal colonization of D. catenatum protocorms. A) In situ hybridization of DcADH mRNA on longitudinal sections of the aseptic and symbiotic protocorms. The location of DcADH2 (LOC110098298) mRNA was detected using the DIG-labeled oligonucleotide probe. DcADH2 shows strong expression signals (Signals are indicated by arrows) at the basal mycorrhizal region (b) but not the apical region (a) in symbiotic protocorm. Aseptic protocorm is the negative control. B) In situ hybridization of DcADH2 sense (instead of antisense) probe (another negative control) in the aseptic and symbiotic protocorms. Scale bars, 100 μm. C) In vivo assays showed that puerarin, an inhibitor of DcADHs, repressed S. indica colonization in symbiotic protocorms on the 9th day after inoculation. Thirty-day-old aseptic protocorms were used as starting material. The relative colonization level was detected using RT-qPCR analysis of SiTef1 normalized to the expression of DcActin7. Data are mean ± SD, n = 4. Two asterisks indicate highly significant differences tested using Student's t-test, P < 0.01. D) Puerarin had no significant adverse effect on the growth of S. indica: The growth of S. indica in OMA medium was similar to that in OMA medium containing 100 μM puerarin. Locations of growing mycelium boundaries are indicated by arrows. E) Proposed working model explaining ADH-regulated mycorrhizal symbiosis in orchid protocorms. Fungal colonization first causes intracellular hypoxia in orchid protocorms, which may be due to fungal competition for oxygen or increased respiration in protocorms. The resulting low intracellular oxygen concentration enhances the expression of hypoxia-responsive genes (ADH, etc.) and promotes the anaerobic metabolism of fermentation and glycolysis. ATP and metabolic intermediates produced by anaerobic metabolic pathways enhance symbiotic protocorm growth. Meanwhile, symbiotic protocorms may supply metabolic intermediates that cannot be synthesized by mycorrhizal fungi to facilitate fungal growth and colonization, thus promoting mycorrhizal symbiosis.
Currently, it is technically difficult to knock out genes in D. catenatum, especially for DcADH with its many homologous genes. As an alternative approach, we used puerarin to inhibit the function of DcADHs and observed its effect on the symbiotic relationship between D. catenatum protocorms and S. indica. We found that puerarin significantly reduced S. indica colonization in D. catenatum protocorms (Fig. 5C) but had no significant adverse effects on the growth of axenic S. indica (Fig. 5D). Meanwhile, another ADH inhibitor, 4-methylpyrazole, also significantly reduced the colonization of S. indica in D. catenatum protocorms (Supplemental Fig. S7), although 4-methylpyrazole at 200 μ M concentration appeared to slightly inhibit the growth of axenic S. indica (Supplemental Fig. S7). In short, these results suggest that the inhibition of ADH activity inhibits S. indica colonization in D. catenatum protocorms. Collectively, our results revealed that ADH and its related hypoxia response pathway are key factors for the symbiotic relationship between protocorms of D. catenatum and its partner fungus S. indica.
Discussion
S. indica is a broad-spectrum orchid-germinating OrM fungus
S. indica was first isolated from the rhizosphere soil of desert shrubs (Verma et al. 1998) and was then found to be able to colonize a large range of hosts (Qiang et al. 2012; Varma et al. 2012) as an endophyte, i.e. via loose colonization without symptoms. This feature is a hallmark of many species of Serendipitaceae (Selosse et al. 2009; Weiss et al. 2016), and, from that stage, several lineages have evolved OrM abilities (Selosse et al. 2022). Indeed, some species related to S. indica are OrM fungi, such as the Serendipita group “vermifera” and S. restingae (Weiss et al. 2016; Fritsche et al. 2021). Although molecular identification revealed S. indica in the roots of Australian and Brazilian orchids (De Long et al. 2013; Oliveira et al. 2014), we provide here the first visual, anatomical evidence that S. indica forms OrM symbiosis, at least at the protocorm stage. We observed that the characteristic hyphal coils, called pelotons, are formed in OrM symbiosis (Dearnaley et al. 2016; Favre-Godal et al. 2020), and their typical old lytic stage is followed by cell recolonization by new pelotons. Our results also showed that S. indica promotes the development of protocorms into plantlets in orchids from the subfamily Epidendroideae and tribes Cymbidieae and Dendrobieae. Orchids have high OrM fungi specificity (Rasmussen et al. 2015; Huang et al. 2018; Meng et al. 2019b; Favre-Godal et al. 2020). Tulasnellaceae are likely the compatible fungi for C. mannii (Chun-Ling et al. 2012), so it is not surprising that S. indica fungus, which is a member of Serendipitaceae, has lower growth promotion for C. mannii. The findings of De Long et al. (2013) and Oliveira et al. (2014) indicate that the tribe Laeliinae (in the same orchid subfamily of our orchid species, Epidendroideae) and Diurideae (subfamily Orchidoideae) also harbor this Sebacinales fungus species. Thus, we propose that S. indica is an OrM fungus with a broad host spectrum both in vitro and in natura.
The acquisition of compatible OrM fungi is often limiting for the successful wild reintroduction and conservation of orchids. Although half of the species investigated in this study did not respond to S. indica, the fungus provides a new resource for orchid conservation. This widely available fungus (Varma et al. 2012) can be tested possibly using other large-spectrum Serendipitaceae strains, whenever the specific mycorrhizal fungi are unknown. Further analyses on a wider set of orchid species may demonstrate whether S. indica displays generalist behavior when endophytic as when OrM.
Moreover, thanks to available genomic data on S. indica (Zuccaro et al. 2011), this species offers a relevant model system for further study of the mechanism underlying OrM symbiosis, especially for comparing endophytic and OrM interactions (Selosse et al. 2022)—as S. indica displays both abilities, like the related S. restingae (Fritsche et al. 2021)—and for comparing the mycoheterotrophic and adult autotrophic stages of interaction with host plants (Jakalski et al. 2021). Our investigation of genes related to hypoxia offers an example of relevant studies in a model interaction with S. indica.
ADH activity plays a role in protocorm–fungus interaction
ADH is a key hypoxia-responsive gene in plants, and its expression is highly induced under hypoxic conditions, such as flooding stress (Komatsu et al. 2011; Bailey-Serres et al. 2012; Licausi 2013; Ventura et al. 2020). In addition, ADH overexpression can enhance plant resistance to the pathogenic bacterium Pst DC3000 and to abiotic stresses such as cold stress (Shi et al. 2017; Song et al. 2019; Su et al. 2020). Alcoholic fermentation catalyzed by ADH is predominant in plants and differs from the fermentative metabolism catalyzed by lactate dehydrogenase in human cells (Bailey-Serres et al. 2012; Ventura et al. 2020). ADH-catalyzed fermentation recycles NAD+ from NADH produced during glycolysis to maintain the continuation of glycolysis and produce ATP and metabolic intermediates for nucleotide, lipid, and protein biosynthesis (Bailey-Serres et al. 2012; DeBerardinis and Chandel 2016). Glycolysis is a physiological response of normal tissues to hypoxia, and it has also been observed in cancer cells under normoxic conditions (DeBerardinis and Chandel 2016). Hypoxic responses in plants are also significantly induced in tissues under normoxic conditions, such as in plant meristems, pathogen-infected leaves, and proliferating plant tissues induced by microbial infection (Licausi 2013; Gravot et al. 2016; Kerpen et al. 2019; Hammarlund et al. 2020; Valeri et al. 2021; Wang et al. 2021b). However, it was unclear whether interactions with beneficial fungi involved the hypoxic response in plants.
Endophytic S. indica is known to induce massive changes in host plant gene expression locally (Lahrmann et al. 2015) and by systemic reprogramming (Chen et al. 2022). Generally, colonization of mycorrhizal fungi reprograms host plant metabolism to ensure the supply of needed metabolites to the fungus (Zhao et al. 2013; Wang et al. 2017; Zheng et al. 2018; Genre et al. 2020; Jakalski et al. 2021). The orchid Serapias vomeracea may export NH4+ to its OrM partner Tulasnella calospora (Dearnaley and Cameron 2017; Fochi et al. 2017). Investigations of gene expression in D. catenatum (D. officinale) germinating with a Serendipitaceae strain (isolated by seed baiting from plantlets of the same species) revealed higher expression levels of genes assigned to various categories, including defense and stress response, and energy metabolism (Zhao et al. 2013). However, hypoxia-related functions were not reported in these studies.
Our study showed that ADH and other key hypoxia-responsive genes were highly induced during the interaction between the host plant D. catenatum and beneficial mycorrhizal fungus S. indica. The expression of DcADH predominantly occurred at the basal mycorrhizal region in symbiotic protocorms. ADH inhibitor treatment reduced S. indica colonization in these symbiotic protocorms. Mycorrhizal fungi often lack a few basic metabolic processes, such as fatty acid biosynthesis in AM fungi (Jiang et al. 2017; Luginbuehl et al. 2017; Wang et al. 2017). One possibility is that ADH activity during the hypoxic response contributes to a hitherto unknown biochemical pathway that regulates the synthesis of metabolites required for symbiosis (Fig. 5E). For example, metabolites that cannot be synthesized by mycorrhizal fungi may be supplied by orchid protocorms to support the symbiotic relationship. In fact, this hypothesis is also supported by our transcriptome data, which show that the expression of genes involved in glycolysis and other metabolic pathways (such as amino acid biosynthesis) is reprogrammed in symbiotic protocorms. Future studies should aim to identify the metabolites that regulate mycorrhizal symbiosis during mycoheterotrophy and analyze the synthesis and transport pathways of these metabolites.
Materials and methods
Germination of symbiotic orchid seeds
S. indica (syn. P. indica) fungus strain DSM11827 was grown and conserved in PDA medium (200 g L−1 potato water [filtrate obtained after boiling peeled and chopped potatoes in clear water], 20 g L−1 dextrose, and 20 g L−1 agar). The seeds of each orchid species (Table 1) were surface-sterilized with 0.02% (v/v) sodium hypochlorite solution for 5 min, rinsed with sterile water, inoculated with the fungus in OMA medium (4 g L−1 ground oats and 8 g L−1 agar), and cultured in an illuminated incubator at 25 °C with a 12-h-light/12-h-dark photoperiod. The number and viability of orchid seeds were assessed before fungal inoculation (Meng et al. 2019a), and the estimate of viable seeds was used in subsequent statistics. Germinated orchid seeds develop first into protocorms and then into plantlets characterized by the initiation of leaf and root formation. Therefore, we calculated the percentage of protocorms or plantlets at 20 and 90 d after incubation.
Resin sections for light microscopy
To detect the colonization pattern of S. indica in the protocorms via tissue sections, symbiotic protocorms were fixed in formalin:acetic acid:alcohol fixative overnight. After dehydration in an ethanol series [50, 75, 85, 95, and 100% (v/v); 2 h each step] at room temperature, the samples were infiltrated in 2:1 (v/v) ethanol:LR white acrylic resins (L9774; Sigma-Aldrich, USA) for 1 h, 1:2 (v/v) ethanol:LR white acrylic resins for 2 h, and 100% LR white acrylic resins overnight at 4 °C and finally embedded in LR white acrylic resins. Semi-thin sections (3 μm) were stained with 1% (w/v) TBO and observed under a light microscope (Chen et al. 2010).
Transcriptome analysis of symbiotic protocorms
D. catenatum seeds were germinated and aseptically (i.e. without fungal inoculation) cultured in Hyponex germination medium (3 g L−1 Hyponex No.1 [N:P:K = 7:6:19; S18147; Shanghai Yuanye Bio-Technology, China], 2 g L−1 peptone, 20 g L−1 sucrose, 40 g L−1 potato homogenate, and 6.5 g L−1 agar, pH 5.8) (Zhang and Gao 2020). Thirty-day-old protocorms were used as the starting material to inoculate with S. indica on OMA medium (symbiotic medium). Samples of aseptic protocorms (control) and protocorms inoculated with S. indica (Si) for 9 d were collected in liquid nitrogen and then used for transcriptome analysis. Total RNA extracted from control and Si samples was used for sequencing with the Illumina HiSeqTM 2000 platform. The clean reads were mapped to the D. catenatum genomic database (Zhang et al. 2016). Differential expression analysis was performed using DESeq2. Expression-altered genes with |log2(fold change)| ≥ 1 and Q-value ≤ 0.05 (Si divided by control) were considered to be significant DEGs. The genes with log2(fold change) ≥ 1 were considered upregulated genes and those with values ≤ −1 downregulated genes. Heat map showing expression patterns of the upregulated and downregulated genes in S. indica-inoculated protocorms compared with controls. In the heat map analysis, the values in the individual samples were represented by Z-scores, and Z-scores were normalized values by scaling (also called z-transforming) the row values of the gene expression matrix. Different colors (red and blue or green) represent the relative expression values of the same gene among different samples in the same row. Pathway annotation against KEGG pathways for all DEGs was implemented, and the pathways with a hit number > 10 were statistically analyzed. Pathways meeting a statistical cut-off (P value < 0.1) were considered to be significantly changed. The data that support the findings of this study have been deposited into CNGB Sequence Archive (CNSA) (Guo et al. 2020) of China National Gene Bank Data Base (CNGBdb) (Chen et al. 2020) with accession number CNP0003765. Blast analysis in the D. catenatum genome was conducted using Arabidopsis ADH1 protein sequence, and 12 homologous genes in the D. catenatum genome were found to be annotated as ADH.
RT-qPCR analysis of symbiotic protocorms
The expression of SiTef1 (GenBank: AJ249911; Nizam et al. 2019) was analyzed using RT-qPCR to measure the colonization level of S. indica in the protocorms. Samples of aseptic protocorms (control) and protocorms inoculated with S. indica (Si) for 3, 9, and 18 d were collected for RT-qPCR analysis. The collected protocorm samples were immediately placed in liquid nitrogen and ground using a tissue grinder (OSE-Y50; Tiangen, China), and their total RNA was extracted with TRNzol Universal Reagent (DP424; Tiangen, China). First-stand cDNA synthesis and qPCR were performed using the One-Step gDNA Removal and cDNA Synthesis SuperMix kit (AT311; TransGen, China) and SuperReal PreMix Plus (SYBR Green) kit (FP205; Tiangen, China), respectively. Transcriptome analysis showed that the expression of DcActin7 (LOC110111141) was almost identical in the aseptic and symbiotic protocorms (Supplemental Fig. S3B); thus, we used DcActin7 as the internal reference for RT-qPCR. All primers are listed in Supplemental Table S1.
mRNA in situ hybridization
The aseptic and symbiotic protocorms were fixed overnight in fresh 4% paraformaldehyde solution (pH 7.2) at 4 °C and finally embedded in Paraplast (P3683; Sigma-Aldrich, USA). Gene-specific fragments of the 5′-UTRs of DcADH2 (LOC110098298) were used as templates for the synthesis of DIG-labeled RNA probes with the DIG RNA labeling kit (Code 11277073910; Roche, Switzerland). Primers used for the probe synthesis were DcADH2-5F: GGCGGCTCACATGGAAATAGGAGTT and DcADH2-5R: TAATACGACTCACTA TAGGGGATGATTTGACCCGCAGTCCTCGAC (the sequence in italics is the T7 promoter sequence). Sectioning and section treatments were performed as described previously with slight modifications (Kramer 2005). 1× PBS solution was replaced with diethylpyrocarbonate-treated water before hybridization, and the hybridization temperature was 53 °C. Images were captured with a Leica DM3000 LED microscope.
ADH activity assays
The catalytic activity of ADH was measured using a spectrophotometric method (Chen et al. 2014). We improved the method to measure the reduction activity of ADH, which converts NADH to NAD+. The activity of ADHs was detected in the reaction mixture (PBS buffer [10 mM Na2HPO4, 2 mM KH2PO4, 137 mM NaCl, and 2.7 mM KCl, pH 7.4] containing 0.2 mM NADH and 2 mM acetaldehyde as substrate). To initiate the reaction, the protein solution was added to the reaction mixture. The activity of acetaldehyde reduction to ethanol catalyzed by ADH was determined by measuring the decrease in NADH absorption at 340 nm and 25 °C. One unit (U) of ADH activity was defined as the amount of enzyme required to decrease 1 μmol NADH absorption per min per mg of protein under the experimental conditions. The protein solution used in the ADH activity assays was the total protein extracted from protocorm tissues with Buffer F (50 mM Tris-HCl, pH 7.5, 25 mM NaCl, 2 mM MgCl2, 1 mM EDTA, 5% (v/v) glycerol, and 0.2% (v/v) β-mercaptoethanol) or the purified recombinant proteins. Among them, the recombinant proteins DcADH1 or DcADH2 were expressed and purified in E. coli. The coding sequences for DcADH1 (LOC110103190) and DcADH2 (LOC110098298) were amplified and cloned into the prokaryotic expression vector pGEX-4T-1 (N-terminal GST tag). The constructed vectors were transformed into Transetta (DE3) E. coli strain (CD801; TransGen, China). Protein expression and affinity purification were conducted using BeaverBeads GSH Magnetic Beads (70601; Beaver, China). Briefly, the expression of recombinant proteins was induced by adding 0.5 mM isopropyl-β-D-thiogalactoside at 25 °C for 12 h with shaking at 220 rpm. The collected E. coli cell pellets were lysed in lysis buffer (50 mM Tris-HCl, 150 mM NaCl, 1 mM EDTA, and 0.1% Triton X-100, pH 7.4) for affinity purification. The proteins bound to the beads were washed with washing buffer (50 mM Tris-HCl, 150 mM NaCl, and 1 mM EDTA, pH 7.4) and eluted with elution buffer (50 mM Tris-HCl, 150 mM NaCl, and 15 mM GST [glutathione], pH 8.0). The purified recombinant proteins of GST-DcADH1 and GST-DcADH2 were confirmed by SDS-PAGE and used in ADH activity assays.
ADH inhibitor assays
Puerarin (CAS: 3681-99-0; BP1176, Biopurify, China) is an isoflavone with inhibitory effects on ADH (Chen et al. 2014). 4-Methylpyrazole (CAS: 7554-65-6; HY-B0876, MedChemExpress, USA) is a competitive inhibitor of ADH in plants (Perata and Alpi 1991). To evaluate their inhibitory effects on the reduction activity of DcADH1/2, puerarin or 4-methylpyrazole at different concentrations was added to the reaction mixture before adding purified recombinant DcADH1/2 proteins. All experiments were repeated at least 3 times. The 30-d-old aseptically cultured protocorms were inoculated with S. indica in OMA medium containing 100 μ M puerarin or 200 μ M 4-methylpyrazole. In the control, the protocorms were inoculated with S. indica in an OMA medium without ADH inhibitors. The protocorms were collected on the 9th day after inoculation, and the colonization level of S. indica in the protocorms was detected using RT-qPCR, as described previously.
Accession numbers
The data that support the findings of this study have been deposited into CNSA of CNGBdb with accession number CNP0003765. The accession numbers of the major genes are mentioned in Supplemental Table S1.
Acknowledgments
We thank Hong Liao and Ao-Dan Huang for their valuable help in the in situ hybridization experiments.
Author contributions
J.J.H., J.Y.G., Z.X.X., and X.M.Z. conceived and designed the research. Z.X.X. and X.M.Z. performed the experiments. X.J.C., X.L.F., and N.Q.L. provided the experimental materials. J.J.H., Z.X.X., X.M.Z., H.Y., and B.L. analyzed the resulting data. J.J.H., Z.X.X., B.L., X.M.Z., J.Y.G., and M.A.S. wrote the manuscript.
Supplemental data
The following materials are available in the online version of this article.
Supplemental Table S1. Primers used in this study.
Supplemental Table S2. DEGs in KEGG pathways.
Supplemental Figure S1.S. indica promotes seed germination and protocorm development of many orchids.
Supplemental Figure S2. Heat map analysis for gene expression levels of DEGs in the glycolysis/gluconeogenesis pathway.
Supplemental Figure S3. DcADH1 and DcADH2 are highly induced in symbiotic protocorms.
Supplemental Figure S4. Hypoxia-responsive genes are positively correlated with symbiosis at the temporal level.
Supplemental Figure S5. Purified recombinant DcADH1 and DcADH2 proteins.
Supplemental Figure S6. 4-Methylpyrazole inhibits ADH reduction activity of DcADHs.
Supplemental Figure S7. 4-Methylpyrazole inhibits fungal colonization in D. catenatum protocorms.
Funding
This project was financially supported by grants from the National Key Research and Development Program of China (2022YFC2601100), National Natural Science Foundation of China (31970287 and U1702235), the Joint Special Project on Construction of “First-class Universities and Disciplines” of Yunnan University (202201BF070001-017) and Innovative Research Foundation for Graduate Students of Yunnan University (2021Y409).
Data availability
The data that support the findings of this study have been deposited into CNSA of CNGBdb with accession number CNP0003765. This information was described in the part of “Accession numbers”.
References
Author notes
These authors contributed equally to this article.
Senior author.
The author responsible for distribution of materials integral to the findings presented in this article in accordance with the policy described in the Instructions for Authors (https://dbpia.nl.go.kr/plphys/pages/general-instructions) is: Jia-Jia Han ([email protected]).
Conflict of interest statement. The authors declare no conflict of interest.