-
PDF
- Split View
-
Views
-
Cite
Cite
Tingting Bao, Shadrack Kimani, Yueqing Li, Hongjie Li, Song Yang, Jia Zhang, Qiuyue Wang, Zhaoxuan Wang, Guogui Ning, Li Wang, Xiang Gao, Allelic variation of terpene synthases drives terpene diversity in the wild species of the Freesia genus, Plant Physiology, Volume 192, Issue 3, July 2023, Pages 2419–2435, https://doi.org/10.1093/plphys/kiad172
- Share Icon Share
Abstract
Terpene synthases (TPSs) play pivotal roles in conferring the structural diversity of terpenoids, which are mainly emitted from flowers, whereas the genetic basis of the release of floral volatile terpenes remains largely elusive. Though quite similar in sequence, TPS allelic variants still function divergently, and how they drive floral terpene diversity in closely related species remains unknown. Here, TPSs responsible for the floral scent of wild Freesia species were characterized, and the functions of their natural allelic variants, as well as the causal amino acid residues, were investigated in depth. Besides the 8 TPSs previously reported in modern cultivars, 7 additional TPSs were functionally evaluated to contribute to the major volatiles emitted from wild Freesia species. Functional characterization of allelic natural variants demonstrated that allelic TPS2 and TPS10 variants changed the enzymatic capacity while allelic TPS6 variants drove the diversity of floral terpene products. Further residue substitution analysis revealed the minor residues determining the enzyme catalytic activity and product specificity. The clarification of TPSs in wild Freesia species reveals that allelic TPS variants evolved differently to determine the interspecific floral volatile terpenes in the genus and might be used for modern cultivar improvement.
Introduction
Plant volatiles are small and low-boiling point molecules emitted by plants from various tissues of vegetative and reproductive organs, such as leaves, fruits, and flowers. Generally, they belong to 3 groups based on their biosynthetic source in plants, i.e. terpenoids, phenylpropanoids/benzenoids, and fatty acid derivatives (Pichersky et al. 2006; Dudareva et al. 2013; Muhlemann et al. 2014; Kumari et al. 2017). In terrestrial flowering plants, terpenoids are the structurally and quantitatively leading family of plant-derived organic volatiles, which are known to be exclusively biosynthesized from 2 compartmentally separate pathways: the mevalonic acid (MVA) pathway in cytosol, endoplasmic reticulum, and peroxisomes and methylerythritol phosphate (MEP) pathway in plastids (Pulido et al. 2012; Vranova et al. 2013). Notably, there are enormous variations in the types and amounts of terpenoids produced by individual species (Broekgaarden et al. 2011; Falara et al. 2011; Tholl and Lee 2011; Aros et al. 2012; Magnard et al. 2015; Bao et al. 2020). However, the mechanism of species-specific terpenoid variation in a genus still remains elusive, which is noteworthy to be extensively explored, as floral fragrance plays versatile roles in multiple physiological processes, as well as in the interaction between plants and insects (Hoballah et al. 2007; Klahre et al. 2011; Yang et al. 2013; Byers et al. 2014; Lin et al. 2017; Chen et al. 2018; Knauer et al. 2018). Moreover, the floral fragrance trait in ornamental flowers possesses high agronomic commercial values. However, in the domestication process of some ornamental plants, the fragrance gradually faded or vanished while the diversity of flower colors and long lifetime of flowering was searched for (Amrad et al. 2016; Raguso 2016). Therefore, wild genetic resources have a great potential for the improvement of floral fragrance of cultivated varieties.
Results have shown that mid-sized gene family encoding terpene synthases (TPSs) accepts the ubiquitous prenyl diphosphates, geranyl diphosphate (GPP), (E,E)/(Z,Z)-farnesyl diphosphate (FPP), neryl diphosphate (NPP), and geranylgeranyl diphosphate (GGPP) as substrates and converts them into the basic monoterpene, sesquiterpene, and diterpene carbon skeletons, respectively (Falara et al. 2011; Tholl 2015; Abbas et al. 2017; Bao et al. 2020). These TPSs can be classified into TPS-a to TPS-h subfamilies in the phylogenetic analysis or be categorized into Class I and Class II types (Chen et al. 2011; Gao et al. 2012; Christianson 2017; Jiang et al. 2019; Jia et al. 2022). Class I TPSs contain the aspartate-rich DDXX(D,E) or NSE/DTE motif at their C-terminal domain, named as “α domain,” which facilitates the substrate(s) cation formation via binding the Mg2+ or Mn2+ that interacts with the prenyl diphosphate molecules (Christianson 2017). Class II TPSs contain a DXDD motif in the “β domain” near the N-terminus, with the second aspartate essential for the protonation-initiated cyclization of GGPP to form copalyl diphosphate (CPP) or other cyclic diterpene diphosphates (Chen et al. 2011; Jia et al. 2022). Generally, TPSs responsible for the floral volatile terpenes are from Class I, and TPSs from angiosperm plants are clustered into TPS-a, TPS-b, and TPS-g subgroups (Chen et al. 2011). The roles of the conserved domains and the basic catalytic properties of angiosperm plant-specific TPSs have been well documented. However, how these TPSs influence the huge diversity of floral volatile terpenes remains elusive.
The selective TPS expression has been shown to determine the volatile diversity in different tissues and developmental stages of plants (Byers et al. 2014; Gao et al. 2018). In addition, although TPSs have conservative N- and C-terminal sequence motifs linked to catalytic properties, some studies have demonstrated that even in species of the same genus, or even in different cultivars or varieties of 1 plant species, their catalytic function may be enhanced, reduced, or even lost (Klahre et al. 2011; Zhou and Pichersky 2020; Yang et al. 2022). Thus, the study of allelic sequence variations and how they affect the catalytic efficiency of TPSs may elucidate the evolution of TPS genes in closely related species and facilitate the product prediction of such TPS proteins. However, previous studies seldom characterized TPSs in different plant species and were less focused on the role of single amino acid in TPS catalysis (Tamer et al. 2003; Kollner et al. 2004, 2020; Garms et al. 2012; Yang et al. 2022). The accurate prediction of product specificities based on amino acid sequences has not yet been possible (Pichersky et al. 2006; Degenhardt et al. 2009).
Attractive scent is one of the most distinguished floral traits of Freesia genus, making it one of the most important ornamentals in the cut-flower trade, particularly in Europe and Japan. Most of the Freesia-cultivated varieties, usually named as Freesia hybrida, that have been produced are scented with different extent. The scent is mainly composed of volatile terpenes, such as linalool, α-terpineol, β-ionone, and copaene (Wongchaochant et al. 2005; Fu et al. 2007; Manning and Goldblatt 2010; Gao et al. 2018; Weng et al. 2021). Eight TPSs (FhTPS1 to FhTPS8) responsible for these major volatile terpenes have been characterized in 2 F. hybrida varieties, and the transcriptional regulation of FhTPS1 has also been studied (Gao et al. 2018; Yang et al. 2020). Recent studies have shown that wild species contain a rich genetic diversity which highlights the importance of characterizing more wild species in terms of floral scent diversity (Bischoff et al. 2014; Zu et al. 2016; Li et al. 2018a). Correspondingly, preliminary analysis of the floral volatile compounds within the wild Freesia genus reveals the chemo-diversity among individual species with compounds not detected in the cultivated varieties and some species that may have lost the ability to release floral fragrance (Wongchaochant et al. 2005; Manning and Goldblatt 2010). These observations motivate the analysis of the composition of floral terpenoids and molecular mechanism accompanying the floral fragrance biosynthesis among different species within the Freesia genus. In this study, volatile compounds released from flowers of 9 wild species were detected, and 7 additional TPSs (TPS9 to TPS15) were cloned and functionally characterized. In addition, allelic natural variations responsible for the major volatile terpenes were investigated to decipher the evolution of TPSs in the Freesia genus. The results of this study will shed light on the genetic basis of floral volatile terpene biosynthesis, dynamic evolution of duplicated TPSs in the Freesia genus, and may contribute to the establishment of ornamental flower varieties with desired fragrances.
Results
TPS genes from wild Freesia species are similar to previously studied plant TPS genes
The whole transcriptome of blooming flowers was sequenced and analyzed using bioinformatics toolkits, yielding a total of 15 TPS putative genes which have been isolated using specific primers (Supplemental Tables S1 and S2), among which 8 were homologous genes of those previously cloned and functionally assayed from cultivated Freesia varieties (from TPS1 to TPS8, Gao et al. 2018). Subsequently, 7 additional TPSs were additionally isolated and compared with TPSs from other plants (Supplemental Table S3). The cloned 7 TPS genes were named as FcaTPS9, FviTPS10, FreTPS11, FviTPS12, FcoTPS13, FreTPS14, and FreTPS15, from the species where they were initially found (a prefix containing the species names such as Fca for Freesia caryophyllacea) (Supplemental Table S2). Moreover, TPSs responsible for sesquiterpene biosynthesis in angiosperms usually cluster in the TPS-a subgroup while those mainly responsible for monoterpene synthesis are in TPS-b. In contrast, the TPS-g family has both monoterpene and sesquiterpene synthase functions. Phylogenetically, 8, 4, and 3 members of the identified 15 TPSs belonged to TPS-a, TPS-b, and TPS-g, respectively (Fig. 1A; Supplemental Table S2). Canonical TPS proteins have typical conserved domains such as DDXXD and NSE/DTE involved in binding divalent metal cations except FcaTPS4 in which the typical conserved domain did not fully conform to the NSE/DTE motif pattern (Fig. 1B). Additionally, except FcaTPS4, FfeTPS6, FleTPS7, FcaTPS9, and FcaTPS14, the N-termini of other TPSs contained the conserved domain R(R,P,Q)(X)8W known to possess catalytic properties for substrate cyclization (Fig. 1B).
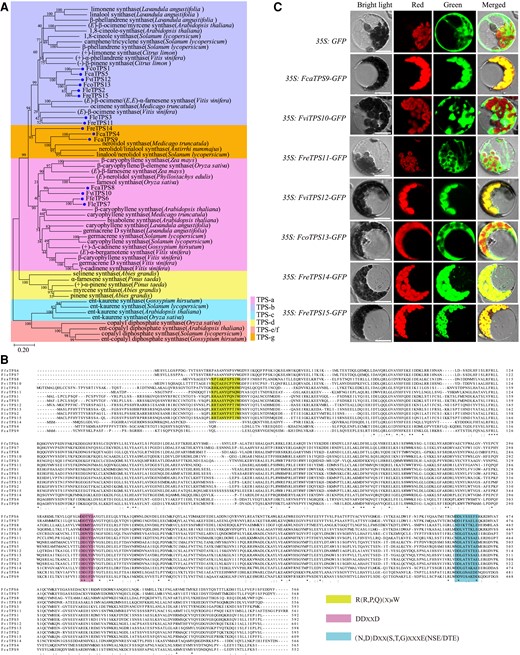
The identification of TPS genes in wild Freesia species. A) Phylogenetic analysis of TPS proteins from Freesia and other plants. The percentage of replicate trees in which the associated taxa clustered together in the bootstrap test (1,000 replicates) are shown next to the branches. The tree is drawn to scale, with branch lengths in the same units as those of the evolutionary distances used to infer the phylogenetic tree. The evolutionary distances are computed in the units of the number of amino acid substitutions per site, as shown by the scale below the tree. Freesia TPS proteins are indicated by blue balls. GenBank accessions of TPS proteins are provided in Supplemental Table S3. B) Amino acid sequence alignment of Freesia TPS proteins. The conserved domains R(R, P, Q)(X)8W, DDXXD, and NSE/DTE are highlighted. Numbers represent positions of the last residue in each line. *, identical amino acids; : or ·, similar amino acids. C) Subcellular localizations of the cloned TPS9 to TPS15 from representative wild Freesia species observed in Arabidopsis protoplasts. Bright light, bright field image; green, GFP fluorescence detected in the green channel; red, chlorophyll autofluorescence detected in the red channel; merged, merged green and red channel images. Bars = 25 µ M.
The biosynthesis of monoterpenes and sesquiterpenes is thought to be compartmentalized, with monoterpenes produced in the plastids where GPP is synthesized, and sesquiterpenes formed in the cytosol where FPP is generated (Chen et al. 2011; Dudareva et al. 2013). Subcellular localization analysis showed that FcaTPS9, FviTPS12, FreTPS14, and FreTPS15 were localized in the plastids, whereas FviTPS10, FreTPS11, and FcoTPS13 were distributed in the cytoplasm (Fig. 1C). However, the sites where TPSs may function and their subcellular locales are not completely consistent (Gutensohn et al. 2012; May et al. 2013; Chen et al. 2017). Therefore, these 15 TPS genes obtained from wild Freesia species might be responsible for the volatile compounds released from the wild Freesia resources, among which TPS1 to TPS8 might be responsible for the shared volatile terpenes between Freesia wild species and cultivated varieties, whereas the 7 additional TPS genes (from TPS9 to TPS15) might attribute to the floral volatile terpenes specific to the wild species.
Composition analysis of floral scent and TPS expression patterns reveal interspecific variations among wild Freesia species
To identify floral volatiles of the wild Freesia species, flowers blooming in the first day were chosen to trap the emitted organic compounds. Consequently, approximately 49 volatile compounds were detected (Fig. 2A; Supplemental Fig. S1), dominated by linalool, nerol, α-terpineol, β-caryophyllene, and β-ionone in different species. The relative contents of terpenes were calculated based on the standard curve of linalool (Supplemental Fig. S2). Briefly, linalool accounted for about 32.23% [1,649.22 ± 193.46 ng/g FW (fresh weight of whole flowers)], 36.27% (1,041.91 ± 35.98 ng/g FW), and 94.56% (3,436.59 ± 242.85 ng/g FW) in Freesia verrucose, F. caryophyllacea, and Freesia corymbosa, respectively. Nerol accounted for 80.49% (44,931.15 ± 868.91 ng/g FW) in Freesia refracta, α-terpineol accounted for 50.19% (45,787.29 ± 1,472.36 ng/g FW) in Freesia leichtlinii, and the content of β-caryophyllene was 4,723.4 ± 458.97 ng/g FW accounting for 51.31% in Freesia viridis. Some carotenoid derivatives were also major components in some species, for instance, β-ionone accounted for 87.48% (138,085.44 ± 13,082.61 ng/g FW), 34.95% (998.18 ± 34.47 ng/g FW), and 62.8% (28,422.62 ± 1,849.24 ng/g FW) in Freesia fergusoniae, F. caryophyllacea, and Freesia speciosa, respectively (Supplemental Table S4). Moreover, among the monoterpene/sesquiterpene detected, linalool and limonene were the 2 components shared by all the 6 scented wild Freesia species (Fig. 2A). As previously reported, among the volatiles detected in the cultivated varieties (named as F. hybrida), linalool and α-terpineol were dominant components (Wongchaochant et al. 2005; Manning and Goldblatt 2010; Gao et al. 2018), which could indicate that the F. hybrida have been domesticated from F. corymbosa and F. leichtlinii (Manning and Goldblatt 2010).

Volatile terpene emissions and TPS expression patterns among wild Freesia species. A) Contents of volatile compounds released from blooming flowers of different wild Freesia species. The release patterns of the volatile compounds are illustrated in Supplemental Fig. S1 and relative contents are detailed in Supplemental Table S4. The mean contents of the volatiles relative to linalool are processed by TBtools with HeatMap Illustrator plugin (Chen et al. 2020). B) PCA of volatile components detected from wild Freesia species. The PCA is performed on Freesia species and volatiles, by TBtools with basic PCA plugin. C) Relative expression levels of Freesia TPS genes in fully blooming flowers of different wild Freesia species. Top right triangles represent data from FPKM values of RNA-seq while bottom left triangles indicate data from RT-qPCR. Primers used in RT-qPCR are provided in Supplemental Table S1. Ct values are compared with Fhactin and Fhubi. The mean expression values are normalized by log2 and illustrated by TBtools with HeatMap Illustrator.
To further explore the volatile pattern in the Freesia genus, we used principal component analysis (PCA) to analyze the volatile compounds in 8 scented species (Fig. 2B). Principal component one (PC1) and principal component two (PC2) accounted for 57.3% and 21.1% of the total variation, respectively. The 8 wild Freesia species could be divided into 2 groups based on PC1. In detail, F. leichtlinii, F. fergusoniae, F. speciosa, F. viridis, and F. refracta were more similar, whereas F. corymbosa, Freesia verrucosa, and F. caryophyllacea clustered together. Moreover, PCA was used to investigate the detected volatiles contributing substantially to the diversity of the 8 wild Freesia species. Carotenoid derivatives such as β-ionone and dihydro-β-ionone were the most diverged components, followed by α-terpineol, β-ocimene, α-selinene, and β-caryophyllene (Fig. 2B). In conclusion, these volatiles contributed more to the diversity of wild Freesia species.
TPS abundance usually corresponds with the floral volatile emission pattern (Gao et al. 2018). Herein, we determined the expression patterns of the 15 TPSs within the 9 Freesia species using expected number of fragments per kilobase of transcript sequence per million base pairs sequenced (FPKM) values calculated from the assembled transcriptome database and reverse transcription quantitative PCR (RT-qPCR) (Fig. 2C). The expression patterns of most TPSs obtained from RT-qPCR experiments coincided well with those of transcriptome-derived FPKM values. Conspicuously, TPS1, which was previously characterized as a linalool synthase in F. hybrida, had relatively high and similar transcript abundance among most wild Freesia species especially in F. verrucose, F. corymbosa, F. fergusoniae, F. leichtlinii, and F. caryophyllacea. TPS2 and TPS6 encoding α-terpineol and α-selinene synthase, respectively, in F. hybrida were found to be highly expressed in F. leichtlinii and F. fergusoniae. Moreover, the cloned TPS9 and TPS14 were highly expressed in F. caryophyllacea and F. refracta respectively, whereas TPS10 had comparable transcripts in F. speciosa, F. leichtlinii, F. caryophyllacea, and F. viridis. In addition, correlation analysis between the main volatile terpenes and these highly expressed Freesia TPS genes showed that TPS1, TPS6, TPS10, and TPS14 were significantly correlated with the emissions of linalool, α-selinene, β-caryophyllene, and nerol, respectively (Supplemental Fig. S3). TPS2 transcript had positive correlations with either limonene or α-terpineol while no obvious correlation was observed between TPS9 and the main volatiles (Supplemental Fig. S3). Taken together, these highly expressed TPS genes should play a role in the biosynthesis of these dominant terpenes.
Biochemical analysis of monoterpene and sesquiterpene synthases shows promiscuity and plasticity
Studies have suggested that TPS genes evolve rapidly (Chen et al. 2011; Huang et al. 2022; Jia et al. 2022). Although TPS sequences have certain conserved types and even putative orthologs share the same residues in most sites, their catalytic products might vary greatly. In other words, TPS1 to TPS8 which had already been characterized from F. hybrida might have diverse functions in wild species considering the pivotal roles of nucleotide polymorphism in allelic TPS variants, and thus, a detailed functional evaluation of TPS enzymes is still required (Srividya et al. 2015; Kollner et al. 2020; Yang et al. 2022). Therefore, the 7 additionally mined TPS9 to TPS15, as well as the earlier characterized TPS1 to TPS8, were tentatively cloned from specific wild Freesia species here and their recombinant proteins were purified from Escherichia coli BL21(DE3) (Supplemental Fig. S4). In vitro enzymatic assays of TPS1 to TPS8 from representative wild species were firstly conducted to check their functions with GPP, NPP, (E,E)-FPP, or (Z,Z)-FPP (Supplemental Fig. S5 and Table S5). In detail, FcoTPS1 and FcaTPS4 shared a similar enzymatic result that both of them could utilize GPP, NPP mainly to generate linalool (Peak 16) and primarily transform (E,E)-FPP into (E)-nerolidol (Peak 83) (Supplemental Fig. S5). Comparatively, GPP or NPP could be catalyzed by FleTPS2 chiefly into α-terpineol (Peak 18) or by FleTPS3 into (Z)-β-ocimene (Peak 10) (Supplemental Fig. S5). More complicated product patterns were observed for FleTPS6, FleTPS7, and FcaTPS8 as they could use all the 4 kinds of substrates, which led to a series of products. However, it seemed that TPS5 from F. caryophyllacea was nonfunctional as no products were captured from the in vitro enzymatic assays (Supplemental Fig. S5), which was in accordance with our earlier study (Gao et al. 2018).
As for TPS9 to TPS15, FcaTPS9 could either transform the canonical monoterpene–related substrate GPP mainly into linalool (Peak 16), or sesquiterpene-related (E,E)-FPP into (E)-nerolidol (Peak 83), occupying 96.62% (490.15 ± 212.47 ng) and 83.07% (392.84 ± 162.16 ng) of the total products, respectively (Fig. 3). FviTPS10 could only use (E,E)-FPP as substrate to yield β-caryophyllene (Peak 30, 79.17 ± 1.89 ng, 79.61%). In contrast, no volatile products were detected when FreTPS11 was incubated with the 4 kinds of substrates (Fig. 3). Moreover, FviTPS12 could only catalyze GPP into a series of monoterpenes mainly composed of α-thujene (Peak 1, 177.34 ± 2.08 ng, 12.94%), sabinene (Peak 4, 268.59 ± 20.12 ng, 23.06%), and γ-terpinene (Peak 13, 279.66 ± 17.42 ng, 24.49%), whereas FreTPS14 could use GPP and NPP to yield geraniol (Peak 21, 133.67 ± 17.92 ng, 75.14%) and nerol (Peak 19, 155.88 ± 13.46 ng, 84.82%), main volatile compounds released by F. refracta (Fig. 3). Comparatively, FcoTPS13 and FreTPS15 were among the TPSs with the highest substrate tolerance, which could utilize GPP, NPP, (E,E)-FPP, or (Z,Z)-FPP, yielding a series of volatile terpenes (Fig. 3). In detail, when using GPP as substrate, (1R)-(+)-α-pinene (Peak 2, 280.45 ± 26.21 ng, 31.53%), (1S)-(−)-β-pinene (Peak 5, 213.53 ± 20.17 ng, 21.52%), and (+)-limonene (Peak 8, 224.75 ± 11.42 ng, 22.91%) were primarily generated by FcoTPS13. In contrast, when NPP was used as substrate, sabinene (Peak 4, 153.43 ± 69.91 ng, 32.17%) and (+)-limonene (Peak 8, 130.4 ± 57.12 ng, 24.86%) were the main products. TPS13 could also catalyze (E,E)-FPP and (Z,Z)-FPP principally into (E)-β-farnesene (Peak 57, 91.69 ± 7.01 ng, 58.77%) and zingiberene (Peak 99, 111.61 ± 35.22 ng, 73.16%), respectively. In comparison, α-thujene (Peak 1), sabinene (Peak 4), myrcene (Peak 6), α-terpineol (Peak 18), α-bergamotene (Peak 26), and β-bisabolene (Peak 73) were all products catalyzed by FreTPS15 from GPP, NPP, (E,E)-FPP, and (Z,Z)-FPP (Fig. 3). In general, the main volatile compounds released by the flowers of wild Freesia species were found in the enzymatic products of recombinant TPS proteins. For example, nerol and β-caryophyllene might be catalyzed by TPS14 and TPS10, respectively, further indicating a relationship between volatile compounds, gene expression, and enzyme activity.
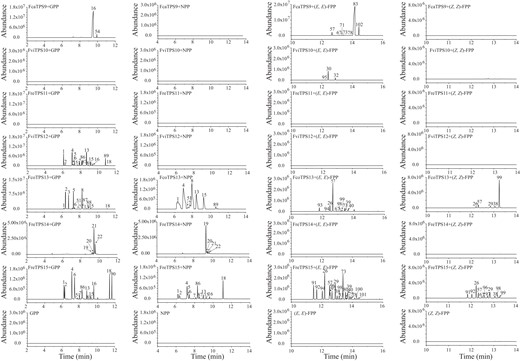
In vitro enzyme activity assay of TPS proteins from wild Freesia species. The relative contents of enzymatic products are detailed in Supplemental Table S6. One to 102 represent different volatiles: 1, α-thujene; 2, (1R)-(+)-α-pinene; 3, camphene; 4, sabinene; 5, (1S)-(−)-β-pinene; 6, myrcene; 7, α-terpinene; 8, limonene; 15, terpinolene; 16, linalool; 18, α-terpineol; 19, nerol; 20, (Z)-citral; 21, geraniol; 22, (E)-citral; 26, α-bergamotene; 29, (Z)-β-farnesene; 30, β-caryophyllene; 32, α-caryophyllene; 33, (−)-α-cedrene; 38, himachalene; 39, (Z)-α-himachalene; 40, β-sesquiphellandrene; 51, α-phellandrene; 54, linalool, methyl ester; 57, (E)-β-farnesene; 60, (E)-α-bergamotene; 67, cubebene; 71, α-farnesene; 73, β-bisabolene; 75, α-patchoulene; 77, (Z)-α-bisabolene; 80, (+)-α-longipinene; 83, (E)-nerolidol; 86, (S)-(−)-limonene; 87, β-phellandrene; 88, (Z)-terpineol; 89, (−)-4-terpineol; 90, terpinyl acetate; 91, α-bergamotene derivant; 92, α-bergamotene derivant; 93, γ-muurolene; 94, α-bergamotene derivant; 95, isocaryophyllene; 96, an unidentified sesquiterpene; 97, an unidentified sesquiterpene; 98, an unidentified sesquiterpene; 99, zingiberene; 100, (+)-ledol; 101, (+)-ledol derivant; 102, (E)-nerolidol derivant.
In vivo bioassay of TPS enzymes shows consistency with in vitro biochemical analysis
To further validate the functional characteristics of the highly expressed TPS genes specific to the wild Freesia species, these genes (TPS9 to TPS15) were transiently overexpressed in Nicotiana benthamiana leaves. Volatile analysis showed that transient overexpression of the TPS genes into N. benthamiana leaves resulted in a series of volatiles. Overexpression of FcaTPS9, FviTPS10, FreTPS11, FcoTPS13, and FreTPS14 mainly contributed to the formation of linalool (100%), β-caryophyllene (97.45%), α-farnesene (51.40%), (+)-limonene (50.20%), and geraniol (100%), respectively (Fig. 4). Notably, FviTPS12 was responsible for the biosynthesis of (1R)-(+)-α-pinene (51.71%) and sabinene (49.29%), whereas FreTPS15 mainly catalyzed the formation of α-bergamotene (18.28%), (E)-β-farnesene (16.88%), and β-bisabolene (16.44%) (Fig. 4). Consistently, these major products detected in the N. benthamiana leaves corresponded with those detected in the cell-free system (Fig. 3; Supplemental Tables S6 and S7). To summarize, considering the in vitro and in vivo enzymatic results, subcellular localizations, as well as expression patterns of the TPSs, it could be concluded that the major compounds linalool, α-terpineol, α-selinene, β-caryophyllene, and nerol released from wild Freesia flower were controlled by TPS1, TPS2, TPS6, TPS10, and TPS14, respectively.
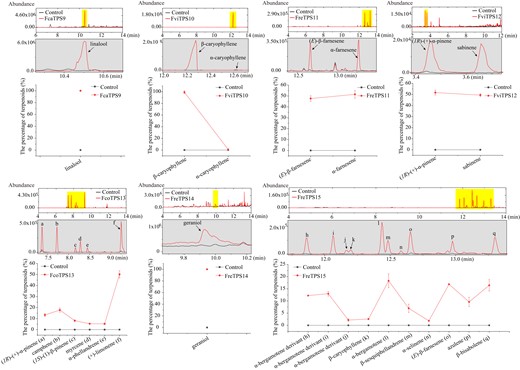
In vivo characterization of the TPS proteins from wild Freesia species. Volatile compounds detected in the control and N. benthamiana leaves transiently overexpressing TPSs. As for each TPS, the upper panel indicates the representative volatile pattern. The highlighted part in the upper panel is enlarged and detailed as the medium panel. The lower panel contains the relative content of detected volatile terpenes which can also be found in Supplemental Table S7.
Allelic variations of TPS2 and TPS10 affect substrate utilization efficiency and in vitro enzyme activity within wild Freesia species
Previous results showed that the alleles of TPS genes substantially diverged within closely related species, which might drive species-specific gain or loss of TPS activity (Kollner et al. 2004; Yang et al. 2022). Based on the results aforementioned, α-terpineol, β-ocimene, α-selinene, and β-caryophyllene were responsible for most of the floral volatile diversity of wild Freesia species (Fig. 2B). These volatiles were produced by TPS2, TPS3, TPS6, and TPS10, respectively (Fig. 3). Therefore, TPS2 and TPS10 were first taken as examples to further decipher the roles and catalytic mechanisms of allelic TPS variants from wild Freesia species.
Sequence alignment revealed that putative TPS2 orthologs from the 8 wild species shared the same residues at most sites (Supplemental Fig. S6A). However, further evaluation by in vitro enzyme activity assay demonstrated that a series of monoterpenoids were differentially produced from the substrate GPP by most putative TPS2 orthologs, among which α-terpineol (Peak 18) was the dominant one (Fig. 5, A and B; Supplemental Table S8). FcaTPS2 seemed to have lost the capacity of synthesizing α-terpineol but is able to synthesize other products, whereas no products were detected in reaction assays containing FreTPS2 or FviTPS2. No α-terpineol was detected in the flowers of F. caryophyllacea, although the expression level of TPS2 in F. caryophyllacea was high (Fig. 2C). A phylogenetic analysis revealed the highest similarity between FleTPS2 and FcaTPS2 as only 2 different residues were observed (Supplemental Fig. S6). In other words, these 2 residues in Positions 97 and 205 might determine α-terpineol formation. Further site mutation assays indicated that the valine in Position 97 of FleTPS2 almost absolutely abolished its capacity of synthesizing α-terpineol, while the arginine in 205 restrained its α-terpineol synthesis capacity (Fig. 5C). Herein, besides transcript abundance, the sequence variation of TPS2 also leads to the α-terpineol difference in flower volatiles, strengthening the fact that functional differences of allelic natural variants do exist in Freesia genus.
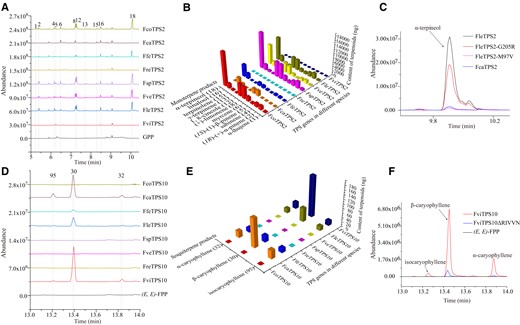
Functional divergence of TPS2 and TPS10 putative orthologs in wild Freesia species. A) Enzymatic patterns of TPS2 proteins from different Freesia species using GPP as substrate. B) Relative contents of enzymatic products of TPS2 natural variants using GPP as substrate. The relative contents are detailed in Supplemental Table S8. The data are calculated relative to α-terpineol (Supplemental Fig. S2B). C) Enzymatic patterns of site-mutated FleTPS2 and FcaTPS2. D) Enzymatic patterns of TPS10 proteins from different Freesia species using (E,E)-FPP as substrate. E) Relative contents of enzymatic products of TPS10 natural variants using (E,E)-FPP as substrate. The relative contents are detailed in Supplemental Table S9. The data are calculated relative to β-caryophyllene (Supplemental Fig. S2C). F) Enzymatic patterns of FviTPS10 and its mutant version. The peak numbers in (A) and (D) represent the corresponding compounds in (B) and (E).
Similarly, β-caryophyllene which was mainly catalyzed by TPS10 (Fig. 3) showed a high degree of quantitative release differences among the 8 wild Freesia species, which could not be finely interpreted by the different TPS10 expressions (Fig. 2A). Sequence alignment revealed a dozen of different residues, indicating a rapid evolution of TPS10 in the Freesia genus (Supplemental Fig. S7). The functional variation of putative TPS10 orthologs was further investigated by in vitro enzyme activity assay. Generally, the ability of TPS10 variants to catalyze β-caryophyllene production varied greatly. In detail, β-caryophyllene was not detected in the assays containing putative TPS10 orthologs isolated from of F. speciosa, F. verrucosa, F. corymbosa, and F. refracta, indicating that these TPS10 allelic natural variants might have lost the function of synthesizing β-caryophyllene during evolution (Fig. 5, D and E; Supplemental Table S9). Moreover, FviTPS10 had the relatively highest substrate utilization activity and produced the most abundant β-caryophyllene. When comparing the sequences of functional FviTPS10, FleTPS10, and FcaTPS10, an insertion of RIVVN amino acids attracted our attention (Supplemental Fig. S7). A subsequent sequence mutation was conducted on FviTPS10 and functionally checked by enzymatic assay. Interestingly, deletion of RIVVN in FviTPS10 substantially restrained its function of β-caryophyllene formation (Fig. 5F). Therefore, FviTPS10 showed great advantages in generating β-caryophyllene among these natural variants in the Freesia genus, which might be used as a preferred allele for future flower quality improvement.
Allelic variations of TPS6 affect the diversity of floral terpene emission in wild Freesia species
In addition to affecting the substrate utilization activity, the allelic natural TPS variants from Freesia species might also determine the composition of their enzymatic products as found in Aquilegia species (Yang et al. 2022). In this study, allelic natural variants of TPS6 genes were chosen for further analysis of this question. The multiple sequence alignment showed that TPS6 variants cloned from the 8 Freesia species could be divided into 4 types based on the sequence identity (Fig. 6A). In vitro enzyme activity assay showed that FcoTPS6, FfeTPS6, FspTPS6, and FveTPS6 primarily yielded β-elemene (Peak 25), α-selinene (Peak 36), (+)-Δ-cadinene (Peak 42), and α-copaene (Peak 24), respectively, when using (E,E)-FPP as substrate (Fig. 6B). The 4 representative sequences were further transiently expressed in N. benthamiana leaves to investigate their functions in vivo. Consequently, α-selinene (Peak 36), (+)-Δ-cadinene (Peak 42), and α-copaene (Peak 24) were the dominant volatile terpenes detected in N. benthamiana leaves transiently overexpressing FfeTPS6, FspTPS6, and FveTPS6, respectively (Supplemental Fig. S8). In contrast to the result from in vitro enzymatic assay, α-copaene (Peak 24) was more abundant than β-elemene (Peak 25) in N. benthamiana leaves transiently expressing FcoTPS6 (Supplemental Fig. S8 and Table S10). Although the function of TPS6 allelic variants substantially varied in the Freesia genus, they shared nearly the same amino acid sequence. For instance, there were only 3 amino acid variances between FspTPS6 and FveTPS6 at positions 287, 303, and 506 (Fig. 6A). Single-point substitutions were performed on the 3 positions in FspTPS6 to make I287A, M303L, and V506A, respectively, followed by protein activity assays in vitro (Fig. 6B; Supplemental Table S11). As results, FspTPS6-based M303L and V506A mutants did not substantially change the product pattern of FspTPS6. In comparison, the mutant FspTPS6-I287A showed similar products with FveTPS6, demonstrating that the single amino acid change in position 287 substantially influenced the product composition of FspTPS6.
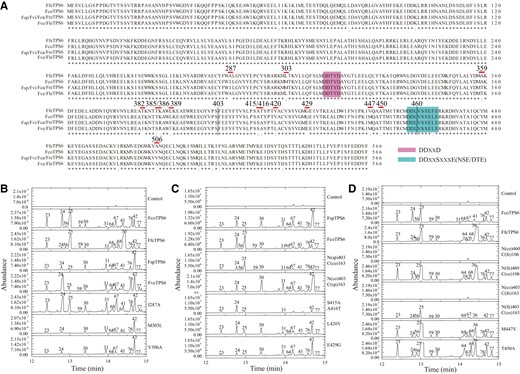
Functional divergence of TPS6 natural variants in wild Freesia species. A) Amino acid sequence alignment of putative TPS6 orthologs from wild Freesia species. The conserved domains DDXXD and NSE/DTE are highlighted and polymorphic amino acids are marked by red lines. The numbers up the lines represent the residue positions. Numbers at the end of each line indicate the positions of the last residue. B) Enzyme assays of representative FcoTPS6-, FfeTPS6-, FspTPS6-, FveTPS6-, and FspTPS6-based mutant proteins. I287A, M303L, and V506A represent site-mutated proteins derived from FspTPS6. C) Key amino acid residue analysis between FspTPS6 and FcoTPS6. L420V, E429G, and S415AA416T mutants are derived from FspTPS6. Fragments of FspTPS6 and FcoTPS6 are swapped at Position 403 to form N(sp)403C(co)163 and N(co)403C(sp)163 chimeras. D) Key amino acid residue analysis between FcoTPS6 and FfeTPS6. Fragments of FcoTPS6 and FfeTPS6 are swapped at Positions 460 and 403 to form N(co)46 0C(fe)106/N(fe)460C(co)106 and N(co)403C(fe)163/N(fe)403C(co)163 chimeras, respectively. M447V and T450A are mutated based on the N(fe)403C(co)163 backbone. All the assays are conducted with (E,E)-FPP. The numbers up the peaks and the relative contents of enzymatic products are detailed in Supplemental Tables S11 to S13.
Moreover, there were 9 residue differences between FspTPS6 and FcoTPS6, among which 5 amino acids (Positions 359, 382, 385, 386, and 389) were close to the N-terminus and another 4 were close to the C-terminus. For convenience, the fragments of FspTPS6 and FcoTPS6 were swapped at Position 403 to form N(sp)403C(co)163 (the 403 amino acids in the N-terminus of FspTPS6 and 163 residues in the C-terminus of FcoTPS6) and N(co)403C(sp)163 (the 403 amino acids in the N-terminus of FcoTPS6 and 163 residues in the C-terminus of FspTPS6) chimeras (Fig. 6A). Consequently, the engineered N(sp)403C(co)163 largely changed the product pattern of FspTPS6, whereas N(co)403C(sp)163 retained the similar function of FspTPS6 (Fig. 6C; Supplemental Table S12). Thus, it was N(sp)403C(co)163 rather than N(co)403C(sp)163 which mimicked the FcoTPS6. The terminus swapping assay indicated that the 4 amino acid residues in the C-terminus seemed to be more critical to determine FspTPS6 activity. To further investigate the key residue, the 4 amino acids in the C-terminus of FspTPS6 were mutated to construct single-site mutant L420V or E429G, and double-site mutant S415AA416T. Further in vitro enzyme assays showed that the S415AA416T mutation largely affected the function of FspTPS6 (Fig. 6C; Supplemental Table S12).
In addition, FfeTPS6 and FcoTPS6 were also selected to further explore the key sites affecting the functions of these 2 proteins by the similar strategy aforementioned. Terminus swapping was initially performed at Position 403 to obtain N(fe)403C(co)163 (the 403 amino acids in the N-terminus of FfeTPS6 and 163 residues in the C-terminus of FcoTPS6) and N(co)403C(fe)163 (the 403 amino acids in the N-terminus of FcoTPS6 and 163 residues in the C-terminus of FfeTPS6) (Fig. 6A). In vitro enzyme activity experiments showed that N(co)403C(fe)163 gave no evident products, whereas N(fe)403C(co)163 behaved more like FcoTPS6 (Fig. 6D; Supplemental Table S13). To minimize the key residue range, we further swapped the fragments of FfeTPS6 and FcoTPS6 at Position 460 to obtain N(fe)460C(co)106 (the 460 amino acids in the N-terminus of FfeTPS6 and 106 residues in the C-terminus of FcoTPS6) and N(co)460C(fe)106 (the 460 amino acids in the N-terminus of FcoTPS6 and 106 residues in the C-terminus of FfeTPS6) (Fig. 6A). Similar with N(co)403C(fe)163, N(co)460C(fe)106 also gave no evident products, while the enzyme activity of N(fe)460C(co)106 chimera was highly similar to that of FfeTPS6 (Fig. 6D; Supplemental Table S13). The results indicated that amino acid residues between site 403 and site 460 were crucial to the catalytic difference between FcoTPS6 and FfeTPS6. Furthermore, residues at positions 447 and 450 were mutated in the backbone of N(fe)403C(co)163, generating N(fe)403C(co)163-based M447V and T450A, respectively. Results from in vitro enzyme assay showed that either the modified M447V or T450A mimicked N(fe)460C(co)106 or even FfeTPS6 (Fig. 6D; Supplemental Table S13). Consequently, the results showed that sites 447 and 450 were essential for the formation of α-selinene (Peak 36) and could be responsible for the variation in the emission of sesquiterpenes between F. fergusoniae and F. corymbosa.
Discussion
Genetic resources of wild Freesia could be used for the floral scent improvement in cultivated varieties
It has been claimed that genetic diversity has been reduced during the domestication of grain or economic crops; breeders have found ways to go back to their wild relatives to excavate potentially useful genetic resources. Wild crop relatives, including the progenitors of cultivated varieties as well as other closely related species in the genus, have been undeniably beneficial to modern agriculture, especially for the recovery and improvement of the extinct traits in cultivated varieties (Li et al. 2018b; Yu et al. 2021). The genetic diversity of wild and cultivated horticultural plants has been widely explored, which paves the way for the genetic modifications of vegetables, such as potato, tomato, and cucumber (Gao et al. 2018; Zhu et al. 2018; Li et al. 2022; Tang et al. 2022). In contrast, less is known in ornamental plants.
Floral fragrance is a vital factor for the commercial values of ornamental flowers in the market trade. However, the beautiful floral scent is lost in most cultivated varieties which tend to be selected according to quality parameters like long stems, color, and vase lifespan, such as in modern cut roses (Rosa × hybrida) and Freesia cultivars (Borda et al. 2007; Manning and Goldblatt 2010; Caissard et al. 2022). Rose scent is commonly thought to be negatively associated with flower vase lifespan, and intensively pigmented Freesia flowers usually display light floral fragrance; e.g. white and yellow Freesia flowers are reported to be more scented than purple and pink flowers (Manning and Goldblatt 2010). Moreover, the coordination of flower color and fragrance has been confirmed in plant natural communities (Kantsa et al. 2017), indicating that the excessive pursuit of color richness in cultivated varieties might cause the loss of fragrance. In our previous studies, we found that α-terpineol and linalool were the 2 major volatile terpenes released from F. hybrida-cultivated varieties (Gao et al. 2018; Yang et al. 2020) and that these 2 components were also extensively released from F. leichtlinii and F. corymbosa, respectively, which were documented to be the fragrant progenitors of modern cultivated varieties (Manning and Goldblatt 2010). Besides F. leichtlinii and F. corymbosa, 14 additional wild species have also been included in the Freesia genus, which might be considered as genetic resources for the improvement of Freesia fragrances. Indeed, nerol, geraniol, and their derivatives, β-ocimene, limonene, α-selinene, and β-caryophyllene, were the other abundant components in addition to linalool and α-terpineol observed in cultivated varieties (Fig. 2; Manning and Goldblatt 2010). Previously, 6 functional TPS proteins were found to be the key for emission of volatile terpenes in Red River and ambience cultivars, including 1 single product enzyme linalool synthase (FhTPS1) and 5 multiproduct enzymes, i.e. FhTPS2, FhTPS4, FhTPS6, FhTPS7, and FhTPS8 (Gao et al. 2018). Herein, we identified the functions of genes encoding Freesia TPS proteins including 7 additional scent genes, which were involved in biosynthesis of monoterpenes and sesquiterpenes within the Freesia genus. Based on the enzymatic products of TPS proteins, expression profiles, and their subcellular localizations, a model for volatile terpene biosynthesis in flowers of wild Freesia species and cultivated varieties was tentatively proposed (Fig. 7). The identified TPS genes in wild Freesia species have great potential in the improvement of Freesia floral fragrances such as enriching the rosy note of flowers which is generated by geraniol, nerol, and their metabolic derivatives.
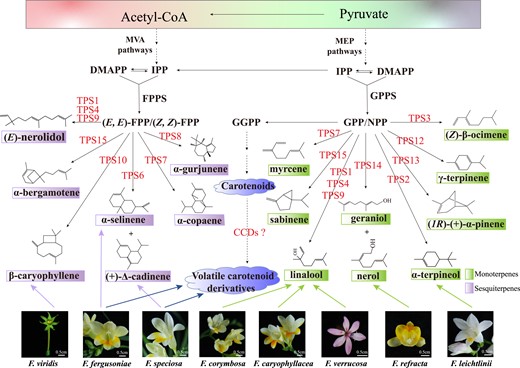
Proposed model of volatile terpene biosynthesis in flowers of wild Freesia species. Linalool, the most abundant volatile terpene released from the flowers of F. corymbosa, F. caryophyllacea, and F. verrucosa, is mainly controlled by TPS1, and TPS4 and TPS9 might also contribute to the biosynthesis of linalool. β-Caryophyllene, α-selinene, (+)-Δ-cadinene, nerol, and α-terpineol, the most abundant volatile terpenes released from flowers of F. viridis, F. fergusoniae, F. speciosa, F. refracta, and F. leichtlinii, respectively, are catalyzed by Freesia TPS10, TPS6 (for both α-selinene and (+)-Δ-cadinene), TPS14, and TPS2, respectively. Moreover, volatile carotenoid derivatives such as β-ionone are abundantly released from F. fergusoniae and F. speciosa by uncharacterized carotenoid cleavage dioxygenases (CCDs). Arrows between compounds with full lines indicate direct reaction. Arrows with dotted lines indicate multiple or uncharacterized steps. Arrows from the flowers represent volatiles released from specific flowers.
Allelic variation of terpene synthases plays determinant roles in the interspecific diversification of floral volatile terpenes
Floral scent, together with flower color and morphology, is the major constituent of floral traits that influence plant interactions with pollinators and other species, and the interspecific variations, as well as intraspecific variations of these floral traits, are widely observed in angiosperm plants (Friberg et al. 2019). Among the floral traits, floral scent is more complicated but less investigated in contrast to the other 2 traits although it is also considered as a crucial pollinator attractant (Hoballah et al. 2007; Kramer and Hodges 2010; Sheehan et al. 2016). How the interspecific diversification of floral scents emerges and maintains has long been questioned, and pollinator-mediated selection, natural enemy-mediated selection, genetic drift and gene flow, and environmental or biochemical constraints are the reported driving forces (Delle-Vedove et al. 2017). However, the genetic basis of the floral scent variation in closely related wild species remains poorly understood. In the Petunia genus (Solanaceae), the quality and complexity of floral scent emission, mainly consisting of phenylpropanoids/benzenoids, are reduced during the transition from moth to hummingbird pollination. Meanwhile, the allelic variant of the CNL (cinnamate-CoA ligase) gene in the hummingbird-pollinated Petunia species is found to be the causal gene responsible for the loss of scent biosynthesis (Amrad et al. 2016). Interestingly, CNL is also involved in the scent loss during the transition from outcrossing to selfing in Capsella (Brassicaceae), indicating that the targets of evolutionary change of interspecific floral scent variation might be conserved in plants (Raguso 2016; Sas et al. 2016).
Comparatively, the diversity and distribution of volatile terpenes in the floral scent are more extensive in contrast to phenylpropanoids/benzenoids, whereas only a small number of genes or their allelic variants have been associated with the interspecific differences. For instance, 3 floral terpenoid volatiles, (+)-limonene, myrcene, and (E)-β-ocimene that are attractive to bumblebee in monkeyflowers but are different between bumblebee-pollinated Mimulus lewisii and hummingbird-pollinated Mimulus cardinalis, and the interspecific diversification are ascribed to 2 allelic variants of TPS genes (Byers et al. 2014). In our recent study, the function of TPS allelic variants not only is consistent to the interspecific differences of floral volatile terpenes but also explains the intraspecific differences of floral emissions in Aquilegia species (Yang et al. 2022). TPS genes constitute a mid-size gene family, which have substantially expanded within species mainly through tandem duplication. After duplication, putative paralogous genes in the family might function divergently, and only a subset of members are specifically expressed in flowers to drive the floral scent emissions (Aros et al. 2012; Jin et al. 2015; Gao et al. 2018; Bao et al. 2020; Baudino et al. 2020). To date, TPS genes are mainly identified in certain species, whereas their evolution and functional diversification are less studied in closely related wild species. In this study, enzymatic assays demonstrate that TPS2 from F. leichtlinii, F. corymbosa, F. fergusoniae, F. verrucosa, and F. speciosa are functional when incubated with GPP, while their allelic natural variants from F. viridis and F. refracta fail to produce the corresponding terpene product α-terpineol (Fig. 5, A and B). This indicates that allelic TPS2 genes play different roles in the wild species of the Freesia genus when their expression levels change. If the expression level was reduced, they could be subfunctionalized or pseudogenized due to mutations in nonsynonymous amino acids. Similar results are also observed in the allelic variants of Freesia TPS10 genes. The relatively higher catalytic activities are obtained in the recombinant proteins encoded by the most extensively expressed FcaTPS10 and FviTPS10 genes (Fig. 5, C and D). In addition to affecting catalytic activities, major products of the allelic TPS variants are also altered. For instance, α-selinene and (+)-Δ-cadinene are the major products of FfeTPS6 and FspTPS6, respectively, which mirrors the floral emissions in the corresponding Freesia species (Fig. 6 and Fig. 2). Though lacking substantial evidences, allelic gene variants might also evolve differently to determine the interspecific floral volatiles, considering the wide variety of floral scents in other ornamental plants such as rose and peony (Paeonia lactiflora) (Magnard et al. 2015; Song et al. 2018).
Allelic variation of TPS from wild plant species is beneficial to identify the crucial amino acid residues for their enzymatic properties
As shown in Fig. 6, the alleles of Freesia TPS6 and their natural variant sequences substantially drive their catalytic activities. For instance, FspTPS6, FveTPS6, FcoTPS6, and FfeTPS6 yielded mostly (+)-Δ-cadinene, α-copaene, β-elemene, and α-selinene, respectively, and the variation might be due to 1 amino acid residue substitution between FspTPS6 and FveTPS6. After site substitution of FspTPS6 to yield FspTPS6-I287A mutant, the activity of the mutant protein was not different from FveTPS6 activity. When fragments of FspTPS6 and FcoTPS6 were swapped at position 403 to form N(sp)403C(co)163 and N(co)403C(sp)163 chimeras, N(sp)403C(co)163 chimera qualitatively lost the ability to yield the major product (+)-Δ-cadinene, whereas the β-elemene content increased substantially. This indicates that the amino acid differences near the C-terminal are critical to FspTPS6 enzyme activity to generate (+)-Δ-cadinene and that S415AA416T mutation largely affects FspTPS6 function to transfer its function to FcoTPS6. In conclusion, it could be postulated that the amino acid residue surrounding the active pocket of the protein is crucial for the enzymatic properties which has been confirmed in other plants. For instance, N338A in 1,8-cineole synthase of Salvia fruticosa, Y402L in β-farnesene synthase of Artemisia annua, and TPSs 4/10 in maize (Zea mays) demonstrate that the by-products of TPSs were determined not only by residues forming the center active pocket but also by adjacent residues impacting the conformation (Kollner et al. 2004, 2020; Kampranis et al. 2007; Karunanithi and Zerbe 2019).
Our results show that the minor amino acid residue differences between these natural variant sequences drive the catalytic activity of enzymes and product specificity. It is beneficial to identify the crucial amino acid residues for their enzymatic properties in wild plant species (Chen et al. 2014; Yang et al. 2022). Results in this study offer insights into the functional diversification and evolution of TPS genes in wild plant species and pave the way to clarify the genetic basis of the interspecific variance of floral scents. In addition, functionally verified TPS proteins as well as identification of crucial amino acid residues will also contribute to the establishment of flower varieties with attractive fragrance.
Materials and methods
Plant resources and growth settings
Nine wild Freesia species, F. verrucosa, F. corymbosa, F. refracta, F. speciose, F. fergusoniae, F. leichtlinii, F. caryophyllacea, F. viridis, and Freesia laxa, were cultivated in a greenhouse with a photoperiod of 16 h light and 8 h dark. To analyze the natural volatile compounds, flowers blooming on the first day were enclosed in a transparent and enclosed device to ensure their normal growth. For RNA-seq and gene expression analysis, flowers were immediately frozen in liquid nitrogen and stored at −80 °C until required. Arabidopsis (Arabidopsis thaliana; Columbia-0) used for subcellular localization in protoplasts were grown in a greenhouse at 25 °C with an alternating photoperiod of 16/8 h light/dark. Leaves of 3- to 4-wk-old Arabidopsis were used for protoplast isolation. For in vivo enzyme activity assay of TPSs, N. benthamiana plants were grown in a greenhouse at 25 °C under natural light. The youngest expanded leaves of 4-wk-old N. benthamiana were used for Agrobacterium infiltration experiments.
Gene identification, cloning, and sequence analysis
The extracted RNA from blooming flowers of the wild Freesia species was subjected to RNA-seq analysis by Novogene Co., Ltd. using routine pipelines. FhTPS1 (GenBank No. AFP23421.1) and AtTPS21 (GenBank No. NP197784.2) were employed as bait queries to BLAST against the assembled transcriptome database by TBtools with BlastZone Plugin (Chen et al. 2020). Moreover, the Pfam numbers PF01397 and PF03936 were used in the built-in Simple HMM Search in TBtools (Chen et al. 2020). The obtained sequences were further processed by a series of analysis such as Swiss-Prot annotation, CD-search, to confirm the Freesia TPS family. Before cloning, the FPKM values of candidate TPS genes were calculated to analyze their expression profiles in different wild Freesia species. TPS genes were isolated from the most highly expressed or all the scented Freesia species using designed primers (Supplemental Table S1). The PCR products with appropriate lengths were cloned into the pESI-Blunt vector (Hieff Clone Zero TOPO-Blunt Cloning Kit, Yeasen, Shanghai, China) and then transformed into E. coli TOP10 competent cells before sequencing confirmation.
TPS proteins from wild Freesia species (Supplemental Table S2) were submitted to Clustal Omega (https://www.ebi.ac.uk/Tools/msa/clustalo/) to perform multiple sequence alignment. For phylogenetic evaluation, the aligned sequences were subjected to MEGA-X to generate a neighbor-joining tree with bootstrap analysis (1,000 replicates) and gap handling by pairwise deletion. TPS proteins from other plants are provided in Supplemental Table S3.
Qualitative and quantitative analyses of volatile terpenoid elements
The volatile analysis was performed following our earlier studies (Gao et al. 2018; Bao et al. 2020; Yang et al. 2022). Briefly, headspace solid-phase microextraction (HS-SPME) was employed to collect the volatile compounds from flowers of wild Freesia species or transgenic N. benthamiana leaves. Total trapped volatile compounds were thermally desorbed and transferred to an Agilent 5975-6890N GC-MS apparatus (Agilent Technologies) to analyze the compounds which were subsequently identified by comparing mass spectra with the NIST 2008 mass spectra library as well as standard samples. The quantitative calculation of volatile terpenoids depended on the standard curves of linalool, α-terpineol, and β-caryophyllene which were the major volatile terpenes released from wild Freesia species flowers. All the volatile compound detections were performed in triplicate.
Subcellular localization of Freesia TPS proteins
The Minerva Super Fusion Cloning Kit (US EverbrightR Inc., Suzhou, China) was used to clone the ORF sequences of TPS genes from the pESI-Blunt vector into the NdeI and ClaI digested pUC19-HA-GmMYBA2-GFP vector in the backbone of the pUC19 vector which was described in our earlier studies (Gao et al. 2021). The plasmids were then extracted using the GoldHi EndoFree Plasmid Maxi Kit (CWBIO, Beijing, China) according to the manufacturer's instructions. The constructs were transfected into protoplasts isolated from Arabidopsis rosette leaves before incubation at room temperature for 20 to 22 h in darkness, as described previously (Li et al. 2018a, 2020a, 2020b, 2022; Shan et al. 2020). Arabidopsis protoplasts transiently expressing GFP and TPS-GFP fusions were visualized by an FluoView FV1000 confocal microscope (Olympus, Japan), exciting with a 488 nm laser; chlorophyll was excited at 546 nm.
RT-qPCR analysis
To investigate the expression profiles of TPS genes in wild Freesia species, a SYBR Green-based RT-qPCR assay was carried out in a total volume of 10 µL of reaction mixture containing 5 µL of 2× Master Mix (TOYOBO, Osaka, Japan), 0.5 µ M of each primer (Supplemental Table S1), and 1 µL template. Fh-actin and Fh-ubiquitin genes were used as internal controls. Relative expression was calculated using the 2−ΔΔCт formula (Livak and Schmittgen 2001). All measurements presented were prepared in triplicate.
Heterologous expression of Freesia TPS proteins in E. coli and in vitro enzyme assay
The Freesia TPSs were amplified with specific primers (Supplemental Table S1) and then seamlessly subcloned into pET-32a(+) or pMal-c2x (only for TPS14). Recombinant proteins were induced by 0.25 mM isopropyl-β-D-thiogalactopyranoside (IPTG) at 16 °C for 20 h. Afterwards, the cells were harvested by centrifugation and disrupted by sonication. The crude proteins were applied to a Ni-TED Sefinose column (Sangon Biotech Co. Ltd., Shanghai, China) for purification. One-hundred micrograms of purified proteins was mixed with 2 mM (E,E)-FPP or GPP or NPP or (Z,Z)-FPP as substrate, 7.5 mM MgCl2, 3.3 mM KCl, 0.6 mM MnCl2, 5 mM dithiothreitol (DTT), and 5% (v/v) glycerol in 50 to 90 mM HEPES buffer (pH 7.4). The mixtures were incubated at 30 °C for 2 h, and the volatiles were analyzed aforementioned. Extracts from E. coli transformed with empty pET-32a(+) and heat-denatured TPS proteins were served as controls and analyzed under the same conditions. The detailed procedures could be found in earlier studies (Gao et al. 2018; Bao et al. 2020; Yang et al. 2022).
In vivo characterization of TPSs
The intact TPS ORFs were cloned into the pEAQ-HT vector (Sainsbury et al., 2009), and the obtained vectors were transformed into Agrobacterium strain GV3101. The abaxial spaces of the youngest leaves of 4-wk-old N. benthamiana plants were infiltrated with the Agrobacterium strains harboring the TPS gene, together with AtGDS1 (A. thaliana GPP synthase 1, NP 850,234.1) or AtFPS2 (A. thaliana FPP synthase 2, NP 001328530.1) at a 1:1 ratio. After infiltration for 4 d, infected leaves were collected and placed in the transparent and enclosed bottles for volatile compound analysis. N. benthamiana leaves infiltrated by Agrobacterium mix containing control pEAQ-HT and AtGDS1 or AtFPS2(1:1) were served as negative controls.
Mutational experimental setup
The PCR-based mutagenesis was executed by the Fast Mutagenesis System (TransGen Biotech Ltd., Beijing, PRC). For instance, site substitutions were performed in FspTPS6 to get I287A, M303L, and V506A variants according to the differences between FspTPS6 and FveTPS6. The TPS6-based chimeras were created by swapping the C-terminus of FspTPS6 with that of FcoTPS6, generating N(sp)403C(co)163 and N(co)403C(sp)163. In addition, site substitutions were also performed (S415A, A416T, L420V, and E429G) in FspTPS6 to verify the roles of amino acid differences in the C-terminus of FspTPS6 and FcoTPS6, which were confirmed by the terminus swapping analysis mentioned above. Similarly, other TPS6 chimeras such as N(co)460C(fe)106/N(fe)460C(co)106 or N(co)403C(fe)163/N(fe)403C(co)163 were created by swapping the respective C-terminus of FcoTPS6 with that of FfeTPS6, and the candidate residues were mutated. All the mutagenized constructs were sequenced for confirmation.
Accession numbers
Sequence data from this article can be found in the GenBank data libraries under accession numbers: FcoTPS1 (OP946880), FleTPS2 (OP946881), FleTPS3 (OP946882), FcaTPS4 (OP946883), FcaTPS5 (OP946884), FfeTPS6 (OP946885), FleTPS7 (OP946886), FcaTPS8 (OP946887), FcaTPS9 (ON777837), FviTPS10 (ON777838), FreTPS11 (ON777839), FviTPS12 (ON777840), FcoTPS13 (ON777841), FreTPS14 (ON777842), and FreTPS15 (ON777843).
Acknowledgments
We would like to thank Sylvie Baudino (Univ Lyon, UJM-Saint-Etienne, CNRS, BVpam, Saint-Etienne, France) for her invaluable assistance in editing and proofreading this manuscript.
Author Contributions
T.B., S.K., Y.L., H.L., S.Y., J.Z., Q.W., and Z.W. performed the experiments and analyzed the data with X.G. and G.N. X.G. and Y.L. formulated the experimental design and discussed with L.W. The manuscript was drafted by S.K., T.B., and Y.L., which was revised by X.G. All authors have participated in this research and approved the final manuscript.
Supplemental data
The following materials are available in the online version of this article.
Supplemental Table S1. Primers used in the study.
Supplemental Table S2. TPS genes cloned from Freesia.
Supplemental Table S3. TPS proteins used in phylogenetic analysis.
Supplemental Table S4. Composition of volatile compounds released from flowers of wild Freesia species.
Supplemental Table S5. In vitro enzymatic products catalyzed by TPS1 to TPS8 proteins from representative wild Freesia species.
Supplemental Table S6. In vitro enzymatic products catalyzed by TPS9 to TPS15 proteins from representative wild Freesia species.
Supplemental Table S7. Volatile compounds detected in the control and transgenic N. benthamiana leaves transiently overexpressing Freesia TPS genes.
Supplemental Table S8. In vitro enzymatic products catalyzed by TPS2 proteins from different wild Freesia species.
Supplemental Table S9. In vitro enzymatic products catalyzed by TPS10 proteins from different wild Freesia species.
Supplemental Table S10. Volatile compounds detected in the control and transgenic N. benthamiana leaves transiently overexpressing the TPS6 gene.
Supplemental Table S11. In vitro enzymatic products catalyzed by representative Freesia TPS6 putative orthologs and its site-mutated versions.
Supplemental Table S12. In vitro enzymatic products catalyzed by FspTPS6-, FcoTPS6-, and FspTPS6-based mutant proteins.
Supplemental Table S13. In vitro enzymatic products catalyzed by FcoTPS6, FfeTPS6, and mutant proteins.
Supplemental Figure S1. Composition and contents of volatile compounds released from flowers of different wild Freesia species.
Supplemental Figure S2. Standard curve of linalool, α-terpineol, and β-caryophyllene.
Supplemental Figure S3. Correlation analysis between the main volatile terpenes and highly expressed Freesia TPS genes.
Supplemental Figure S4. SDS-PAGE and western blotting analysis of purified TPS proteins from different Freesia species.
Supplemental Figure S5. In vitro enzyme activity assay of TPS1 to TPS8 proteins from representative wild Freesia species.
Supplemental Figure S6. Sequence alignment and phylogenetic analysis of TPS2 from different wild Freesia species.
Supplemental Figure S7. Sequence alignment of TPS10 from different wild Freesia species.
Supplemental Figure S8. In vivo characterization of Freesia TPS6 proteins in N. benthamiana leaves.
Funding
This work was supported by the National Natural Science Foundation of China (31972445, 32272751) and the Department of Science and Technology of Jilin Province (20220508112RC, 20210101005JC). The funders had no role in the study design, data collection and analysis, decision to publish, or preparation of the manuscript.
Data availability
All data supporting the findings of this study are available within the paper and within its Supplemental materials published online.
References
Author notes
The author responsible for distribution of materials integral to the findings presented in this article in accordance with the policy described in the Instructions for Authors (https://dbpia.nl.go.kr/plphys/pages/General-Instructions) is Xiang Gao.
Conflict of interest statement. None declared.