-
PDF
- Split View
-
Views
-
Cite
Cite
Yang Lei, Bilang Li, Xiaomin Wang, Junyou Wei, Peiyi Wang, Jun Zhao, Fei Yu, Yafei Qi, Chloroplast SRP54 and FtsH protease coordinate thylakoid membrane-associated proteostasis in Arabidopsis, Plant Physiology, Volume 192, Issue 3, July 2023, Pages 2318–2335, https://doi.org/10.1093/plphys/kiad199
- Share Icon Share
Abstract
Thylakoid membrane protein quality control (PQC), which requires the coordination of membrane protein translocation and degradation of unassembled proteins, determines chloroplast development during de-etiolation. Despite numerous efforts, the regulation of this process in land plants is largely unknown. Here, we report the isolation and characterization of pale green Arabidopsis4 (pga4) mutants in Arabidopsis (Arabidopsis thaliana) with defects in chloroplast development during de-etiolation. Map-based cloning and complementation assays confirmed that PGA4 encodes the chloroplast Signal Recognition Particle 54 kDa (cpSRP54) protein. A heterogeneous Light-Harvesting Chlorophyll a/b Binding-Green Fluorescent Protein (LhcB2-GFP) fusion protein was generated as an indicative reporter for cpSRP54-mediated thylakoid translocation. LhcB2-GFP was dysfunctional and degraded to a short-form dLhcB2-GFP during de-etiolation through an N-terminal degradation initiated on thylakoid membranes. Further biochemical and genetic evidence demonstrated that the degradation of LhcB2-GFP to dLhcB2-GFP was disrupted in pga4 and yellow variegated2 (var2) mutants caused by mutations in the Filamentous Temperature-Sensitive H2 (VAR2/AtFtsH2) subunit of thylakoid FtsH. The yeast two-hybrid assay showed that the N-terminus of LhcB2-GFP interacts with the protease domain of VAR2/AtFtsH2. Moreover, the over-accumulated LhcB2-GFP in pga4 and var2 formed protein aggregates, which were insoluble in mild nonionic detergents. Genetically, cpSRP54 is a suppressor locus for the leaf variegation phenotype of var2. Together, these results demonstrate the coordination of cpSRP54 and thylakoid FtsH in maintaining thylakoid membrane PQC during the assembly of photosynthetic complexes and provide a trackable substrate and product for monitoring cpSRP54-dependent protein translocation and FtsH-dependent protein degradation.
Introduction
During chloroplast development, the proper biogenesis of photosynthetic protein complexes requires translocation of apoproteins into the membrane, assembly of each subunit with the right stoichiometry, as well as the tight regulation of biosynthesis and incorporation of photosynthetic pigments (Pribil et al. 2014; Wang and Grimm 2015; Zhu et al. 2022). Chloroplast Signal Recognition Particle 54 kDa (cpSRP54) protein is the plant homolog of bacterial SRP54, involved in co-translational targeting of nascent chloroplast proteins to thylakoid membrane (Akopian et al. 2013; Hristou et al. 2019). In addition, cpSRP54 is also required for the translocation of Light-Harvesting Chlorophyll a/b binding Proteins (LHCP) to thylakoids, by forming a transit complex with cpSRP43 and the hydrophobic client LHCP (Schünemann et al. 1998; Yuan et al. 2002; Goforth et al. 2004; Holdermann et al. 2012; Ziehe et al. 2018). The assembly of LHCP on thylakoid membranes is tightly coordinated with the biosynthesis of chlorophylls (Wang and Grimm 2015; Wang et al. 2018a). For instance, in Arabidopsis chlorina mutants lacking chlorophyll b, caused by the lesion of chlorophyll a oxygenase, the accumulation of LHC apoprotein was reduced drastically, most likely due to a proteolytic degradation performed by yet unknown chloroplast proteases (Reinbothe et al. 2006). These indicated that the chloroplast protein quality control (PQC) system could work cooperatively with the membrane protein targeting or assembly system when highly hydrophobic photosynthetic apoproteins are prone to be aggregated, misfolded, or photo-damaged during the assembly of photosynthetic complexes. Nonetheless, a convenient and trackable substrate protein marker to dissect the coordination work in vivo has not been reported in land plants.
FtsH (Filamentous Temperature-Sensitive H), belonging to the AAA (ATPases associated with a variety of cellular activities) protease family, is a specific type of membrane-localized metalloprotease to maintain membrane PQC. FtsH exists universally in almost all prokaryotic species and organelles of eukaryotic cells such as mitochondria and chloroplasts (Ogura et al. 1991; Tomoyasu et al. 1995; Arlt et al. 1996; Sakamoto et al. 2003). In Escherichia coli, FtsH is responsible for the degradation of numerous substrates with various physiological functions (Ito and Akiyama 2005; Langklotz et al. 2012) including heat shock sigma factor σ32 (Herman et al. 1995; Tomoyasu et al. 1995). In yeast (Saccharomyces cerevisiae), 2 types of FtsH homologs are anchored to the inner membrane of mitochondria, including the i-AAA protease Yeast Mitochondrial Escape1 (YME1) facing intermembrane space and the m-AAA protease Yeast Tat-binding Analogs10/12 (YTA10/12) complex to the matrix side (Arlt et al. 1996; Weber et al. 1996; Glynn 2017). The proposed FtsH working model for membrane PQC is that the ATPase domain performs as an unfoldase to unfold its substrates to polypeptide chains, which are subsequently translocated to the proteolytic domain for degradation (Suno et al. 2006; Bieniossek et al. 2009; Puchades et al. 2017).
In Arabidopsis, thylakoid FtsH complexes are heterohexamers, composed of type A (AtFtsH1 and 5) and type B (AtFtsH2 and 8) subunits, with the ATPase domain and the protease domain facing the stroma side (Sakamoto et al. 2003; Yu et al. 2004; Zaltsman et al. 2005). The pronounced phenotype of variegated rosette leaves in yellow variegated2 (var2) and yellow variegated1 (var1) was caused by mutations in VAR2/AtFtsH2 and VAR1/AtFtsH5, respectively (Chen et al. 2000; Sakamoto et al. 2002). Complete loss of type A or type B leads to albino seedlings, indicating that thylakoid FtsH is essential for chloroplast development (Yu et al. 2005; Zaltsman et al. 2005). Taking advantage of the leaf variegation phenotype of var2, a set of genetic modifier mutants and their loci, including Suppressors of Variegation (SVR) and Enhancers of Variegation (EVR), were identified recently (Zheng et al. 2016; Wang et al. 2018b; Liu et al. 2019; Qi et al. 2020; Wang et al. 2022). One group of those suppressor loci encode proteins that participate in chloroplast translation directly, including the chloroplast Ribosomal Protein L24 (RPL24) (Liu et al. 2013), the chloroplast translation initiation factor IF3 (Zheng et al. 2016), and the chloroplast translation elongation factor EF-Tu (Liu et al. 2019). These SVRs and EVRs demonstrated that the balance of chloroplast translation and cytosol translation regulates chloroplast development and leaf variegations (Wang et al. 2018b).
Similar to the mitochondrial inner membrane for oxidative phosphorylation (OXPHOS), the thylakoid membrane of chloroplast is where photophosphorylation takes place. Driven by light energy, the electron transfer reaction is achieved by photosynthetic protein complexes that are embedded in thylakoid membranes, including PSII, cytochrome b6f (Cytb6f), and PSI. Among them, PSII is the most vulnerable, and its reaction center proteins are prone to being photo-damaged under high light stress (Aro et al. 1993; Nixon et al. 2010). FtsH plays an essential role in the PQC of photosynthetic protein complexes, for instance, by degrading photo-damaged PSII reaction center protein D1 (Lindahl et al. 2000; Bailey et al. 2002; Silva et al. 2003; Malnoë et al. 2014). Under other abiotic stress conditions such as sulfur deprivation or dark-induced senescence, other potential substrates of thylakoid FtsH have also been reported, including Cytb6f, and light-harvesting complex LHCII and LHCI (Luciński and Jackowski 2013; Malnoë et al. 2014; Bujaldon et al. 2017). However, the assessment of D1 fragment degradation is difficult under nonphotoinhibitory light conditions in Arabidopsis (Kato et al. 2012).
The protein processing activity of FtsH requires the presence of a stable domain existing in the substrate, as the unfoldase activity of FtsH is not robust, and it will release the processed substrate when encountering the stable domain (Okuno et al. 2006; Okuno and Ogura 2013). In this work, we generated a transgenic line expressing a chimeric Light-Harvesting Chlorophyll a/b Binding-Green Fluorescent Protein (LhcB2-GFP) to dissect the regulation of chloroplast PQC and the processing activity of FtsH. Using pale green Arabidopsis mutants, pga4-1 and pga4-2 with disruptions in cpSRP54, we confirmed that the chimeric LhcB2-GFP was targeted to the thylakoid membrane by cpSRP54 and degraded to dLhcB2-GFP mediated by thylakoid FtsH through N-terminal processing. More interestingly, PGA4/cpSRP54 is a genetic suppressor locus of var2 leaf variegation. Our work presented an indicative marker to dissect the proteolytic activity of thylakoid FtsH, and most importantly, provided insight that cpSRP54 and thylakoid FtsH function together for chloroplast PQC during de-etiolation.
Results
PGA4 encodes cpSRP54
To study the mechanisms underlying chloroplast protein homeostasis, we systematically isolated and characterized pale green Arabidopsis (pga) mutants from our ethyl methanesulfonate (EMS) mutagenesis pools (Li et al. 2022). The pga4-1 showed pale-green leaves, indicating defects in chloroplast development and accumulation of photosynthetic pigments and protein complex (Supplemental Fig. S1A). We employed the map-based cloning approach to identify the PGA4 locus (Fig. 1A). Initial mapping placed PGA4 on chromosome 5, and further fine mapping narrowed down the location of PGA4 near the marker F8F6#1 (Fig. 1A). DNA sequencing identified a G to A mutation in the coding region of AT5G03940, resulting in a nonsense mutation (TGA) in the 89th amino acid residue tryptophan (TGG) of cpSRP54 (Fig. 1A). In addition, the second allele of PGA4 mutant, pga4-2 recovered from an activation tagging mutagenesis pool (Yu et al. 2008), was confirmed by the allelism test with pga4-1 and PCR-based genotyping (Supplemental Fig. S1, A to C). RT-qPCR confirmed that the accumulation of cpSRP54 transcripts is severely decreased in pga4-2 (Supplemental Fig. S1D).
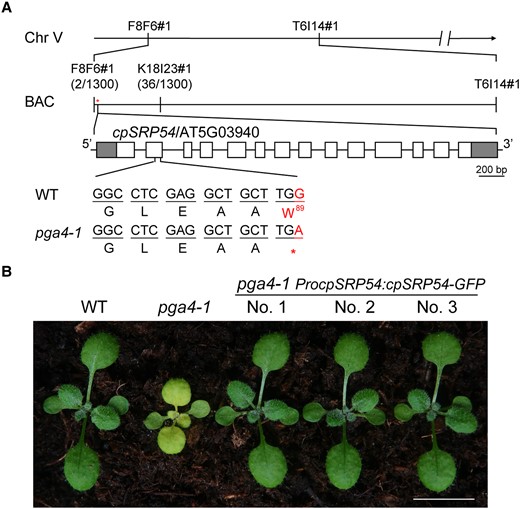
PGA4 encodes cpSRP54. A) The map-based cloning of pga4-1. The PGA4 locus was mapped between F8F6#1 and K18I23#1 using 650 individual plants. In this region, a nonsense mutation (Trp/W89 to a stop codon) in cpSRP54/AT5G03940 was identified. Gray and white boxes represent UTRs and exons of cpSRP54/AT5G03940, respectively. B) Phenotypic complementation of the A. thaliana pga4-1 mutant with the binary construct ProcpSRP54:cpSRP54-GFP. Bar, 1.0 cm.
For a complementation assay, a plant binary vector ProcpSRP54:cpSRP54-GFP was constructed, in which the genomic DNA of cpSRP54 is driven by its native promoter and fused with coding sequences of green fluorescent protein (GFP) at its 3′ end. The pale-green pga4-1 was recovered to the wild-type (WT) phenotype using this vector (Fig. 1B). In pga4-1 ProcpSRP54:cpSRP54-GFP transgenic lines, cpSRP54-GFP were accumulated in etioplasts (0 h) and chloroplasts (24 h) during de-etiolation (Supplemental Fig. S2). These finally confirmed that the pale-green phenotype of pga4-1 and pga4-2 was caused by mutations in cpSRP54.
Generation of a stable transgenic line expressing a chimeric LhcB2-GFP
As cpSRP54 is required for targeting LHC proteins to thylakoid membranes, we tried to express a heterogeneous LhcB2-GFP as a client for cpSRP54. We constructed a binary vector ProLhcB2:gLhcB2-GFP, in which the genomic DNA of AT2G05070 (LhcB2.2) was driven by its native promoter and fused with the GFP coding sequence at its 3′ end (Fig. 2A). To confirm the expression of LhcB2-GFP is consistent with the endogenous LhcB2, we transformed this construct into the WT Arabidopsis. One representative WT ProLhcB2:gLhcB2-GFP line (hereafter designated WT LhcB2-GFP) was treated with lincomycin (Lin) and norflurazon (NF) that could block chloroplast translation and carotenoid biosynthesis, respectively (Susek et al. 1993; Mulo et al. 2003). Chemical treatments led to albino seedlings as expected (Fig. 2B). RT-qPCR analyses revealed that the accumulation of LhcB2-GFP and LhcB2 transcripts was severely reduced in Lin or NF-treated seedlings compared with those in the mock (Fig. 2C). Those together indicated that the expression of LhcB2-GFP in WT LhcB2-GFP is regulated by retrograde repression, being consistent with the endogenous LhcB2.
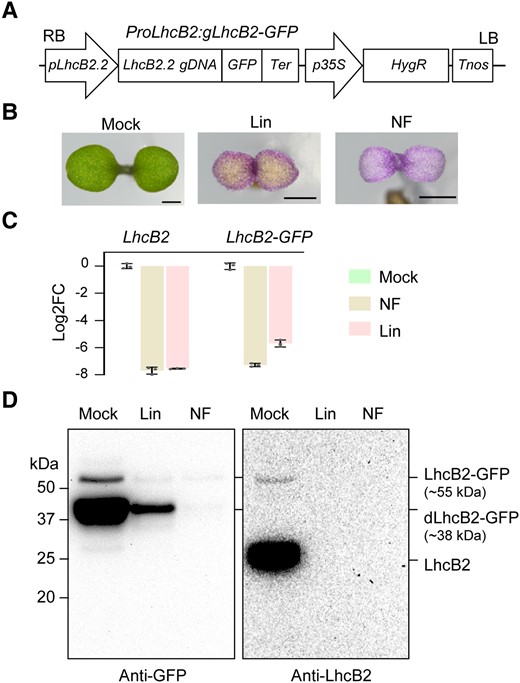
The generation of a transgenic line WT LhcB2-GFP in A. thaliana. A) The vector construct of ProLhcB2:gLhcB2-GFP. B) Seedlings of WT LhcB2-GFP were grown on 1/2 MS (Mock), and 1/2 MS containing 0.5 mM lincomycin (Lin) or 5 μM norflurazon (NF) for 4 d. Bars, 1 mm. C) RT-qPCR analyses of accumulations of LhcB2 and LhcB2-GFP in WT LhcB2-GFP treated with lincomycin and norflurazon as in (B). The base 2 logarithm of the fold-change (Log2FC) was calculated, and PP2A was used as the reference gene. Data are means ± s.d. of 3 biological replicates. D) The accumulation of LhcB2-GFP (∼55 kDa) and dLhcB2-GFP (∼38 kDa) in 4-d-old WT LhcB2-GFP treated with Lin and NF as in (B). An anti-GFP antibody and an anti-LhcB2 antibody were used, respectively. Protein loading was normalized to equal fresh tissue weight.
The accumulation of LhcB2-GFP in those seedlings was examined with immunoblotting, using an anti-GFP antibody and an anti-LhcB2 antibody. A full-length LhcB2-GFP (∼55 kDa) and a degradation form of LhcB2-GFP designated dLhcB2-GFP (∼38 kDa) were highly accumulated in the mock compared with those in seedlings treated with Lin or NF (Fig. 2D). The dLhcB2-GFP was only recognized by the anti-GFP antibody, but not by the anti-LhcB2 antibody (Fig. 2D). Consistently, reduced GFP and chlorophyll fluorescence were observed in cotyledon chloroplasts in seedlings treated with Lin or NF compared with the mock (Supplemental Fig. S3). We also examined the accumulation of LhcB2-GFP during 24-h de-etiolation (Supplemental Fig. S4). This assay could provide a rational starting point to analyze the accumulation of photosynthetic proteins (Qi et al. 2020; Pipitone et al. 2021). Consistent with those in 4-d-old seedlings, both LhcB2-GFP (∼55 kDa) and dLhcB2-GFP (∼38 kDa) were strongly accumulated in 24-h de-etiolated WT LhcB2-GFP (Fig. 2D and Supplemental Fig. S4A). Besides, a larger form of band (∼65 kDa) was observed after a long exposure time, which was likely the LhcB2-GFP precursor (pLhcB2-GFP) containing the chloroplast transit peptide (cTP). As LhcB2-GFP was driven by its native promoter, the accumulation of LhcB2-GFP and dLhcB2-GFP was responsive to light induction (Supplemental Fig. S4A). In 24-h de-etiolated WT LhcB2-GFP, the overlap of GFP and chlorophyll fluorescence confirmed that LhcB2-GFP is targeted into chloroplasts (Supplemental Fig. S4B).
As the anti-LhcB2 antibody could detect endogenous LhcB2 and LhcB2-GFP, but not dLhcB2-GFP (Fig. 2D), it was deduced that the anti-LhcB2 antibody recognizes the N-terminal region of LhcB2. To prove that, we expressed the recombinant LhcB2 proteins, including GST-LhcB2, GST-ΔN-LhcB2, and GST-LhcB2-ΔC in E. coli. The partial N- or C-terminal segments of LhcB2 were removed in the recombinant GST-ΔN-LhcB2 and GST-LhcB2-ΔC, respectively. GST-LhcB2, GST-ΔN-LhcB2, and GST-LhcB2-ΔC were well detected by an anti-GST antibody, whereas only GST-ΔN-LhcB2 was undetectable by the LhcB2 antibody (Supplemental Fig. S5).
The chimeric LhcB2-GFP could be targeted to thylakoid membranes during de-etiolation
The detection of the full-length LhcB2-GFP enables us to check its localization in the chloroplast, via chloroplast fractionation using 24-h de-etiolated seedlings of WT LhcB2-GFP (Fig. 3A). Crude membrane fractions (P) and soluble fractions (S) were isolated, using a hypotonic buffer (HM) or HM buffer containing a higher concentration of ionic salts (2 M NaCl). The large subunit of Rubisco (RbcL, a stroma protein marker) was enriched in soluble fractions, while the endogenous LhcB2 (a thylakoid membrane protein marker) existed in the crude membrane fractions (Fig. 3A). Partial LhcB2-GFP and dLhcB2-GFP were present in the S fraction when HM buffer was used, indicating that they were distributed in the stroma (Fig. 3A). There was still a large portion of LhcB2-GFP and dLhcB2-GFP in the P fractions, and washing with 2 M NaCl buffer did not increase their proportion into the S fractions, suggesting that those were either associated with the membrane fractions or aggregated (Fig. 3A).
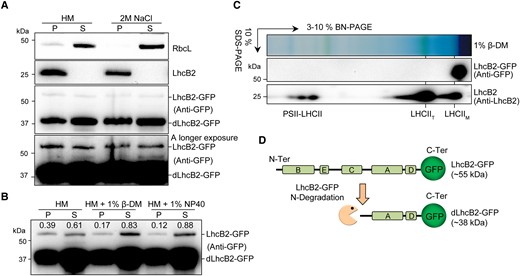
The heterogeneous LhcB2-GFP is not assembled into antenna complexes. A) Subcellular fractionation of LhcB2-GFP and dLhcB2-GFP in 24-h de-etiolated WT LhcB2-GFP. The soluble stroma fraction (S) was indicated by RbcL and the insoluble fraction (P) by endogenous LhcB2. B) The membrane-associated LhcB2-GFP were solubilized with 2 mild nonionic detergents n-dodecyl-β-D-maltoside (1% β-DM) and Nonidet P-40 (1% NP40). The accumulation of LhcB2-GFP in the solubilized and pellet fractions was relatively quantified with signal intensities. C) Thylakoid membrane of 24-h de-etiolated WT LhcB2-GFP was solubilized with 1% β-DM and resolved on 1D BN-PAGE and the 2D SDS-PAGE. The accumulation of LhcB2-GFP and LHCII complexes was detected with the anti-GFP antibody and the anti-LhcB2 antibody, respectively. D) The diagram of the N-terminal degradation of LhcB2-GFP.
To check if LhcB2-GFP and dLhcB2-GFP were associated with thylakoid membranes, we used 2 mild nonionic detergents n-dodecyl-β-D-maltoside (β-DM) and Nonidet P-40 (NP40) to solubilize membrane proteins. In contrast to dLhcB2-GFP, more than half the amount of LhcB2-GFP in the P fraction (0.22 of 0.39 by β-DM, and 0.27 of 0.39 by NP40) were solubilized into the S fractions (Fig. 3B). This suggested that LhcB2-GFP could be associated with thylakoid membranes, and dLhcB2-GFP was mainly in the stroma. However, there were still partial LhcB2-GFP and dLhcB2-GFP insoluble in 1% β-DM or 1% NP40 (Fig. 3B). These demonstrated that the solubilized LhcB2-GFP were targeted onto thylakoid membranes, and the insoluble LhcB2-GFP and dLhcB2-GFP may form protein aggregates.
The chimeric LhcB2-GFP is dysfunctional and degraded to dLhcB2-GFP through N-terminal degradation
The high level of dLhcB2-GFP suggested that LhcB2-GFP is not functioning properly as endogenous LhcB2. We investigated the assembly of membrane-associated LhcB2-GFP (Fig. 3C). Solubilized membrane proteins of 24-h de-etiolated WT LhcB2-GFP were resolved on 1D blue native PAGE (BN-PAGE) and followed by 2D SDS-PAGE and detected with the anti-GFP antibody and the anti-LhcB2 antibody, respectively. The endogenous LhcB2 forms multiple protein complexes as reported (Järvi et al. 2011), including LHCII monomer (LHCIIM), LHCII trimer (LHCIIT), and PSII-LHCII supercomplexes (PSII-LHCII) (Fig. 3C). However, the membrane-associated LhcB2-GFP were unable to be assembled into large functional LHCII antenna protein complexes and present as monomers (Fig. 3C). On 1D BN-PAGE, the apparent migration of LHCIIM was around 66 kDa due to the assembly of photosynthetic pigments (Maekawa et al. 2015). The size of LhcB2-GFP (∼55 kDa) was smaller than LHCIIM (Fig. 3C), indicating that the LhcB2-GFP was not associated with chlorophylls. Based on the reported structure of LHC in spinach (Spinacia oleracea), LhcB2 proteins harbor 5 helices A to E, among which helices B, C, and A are transmembrane helices, spanning across the thylakoid membrane (Fig. 3D) (Liu et al. 2004). Thus, an N-terminal degradation of LhcB2-GFP was proposed, and chloroplast proteases are most likely involved in degrading the dysfunctional LhcB2-GFP (Fig. 3D).
Loss of cpSRP54 affects the translocation of LhcB2-GFP to thylakoid membranes and the degradation of LhcB2-GFP to dLhcB2-GFP
To check if the chimeric LhcB2-GFP is targeted to the thylakoid by cpSRP54, we crossed pga4 mutants with WT LhcB2-GFP to generate pga4 LhcB2-GFPs. During 24-h de-etiolation, pga4 LhcB2-GFPs showed almost completely delayed greening phenotypes and reduced accumulation of photosynthetic pigments, compared with those in WT LhcB2-GFP (Fig. 4, A and B). These indicated that cpSRP54 is required for chloroplast development during de-etiolation. The accumulation of LhcB2-GFP and dLhcB2-GFP were analyzed in 24-h de-etiolated WT LhcB2-GFP and pga4-1 LhcB2-GFP (Fig. 4C). Strikingly, LhcB2-GFP was largely accumulated in de-etiolated pga4-1 LhcB2-GFP compared with those in de-etiolated WT LhcB2-GFP (Fig. 4C). On the other hand, the level of dLhcB2-GFP was sharply reduced in de-etiolated pga4-1 LhcB2-GFP compared with that in de-etiolated WT LhcB2-GFP (Fig. 4C). The accumulation of LhcB2 in pga4-1 LhcB2-GFP was comparable to that in WT LhcB2-GFP (Fig. 4C). Similar observations on the accumulation of LHCPs had been reported in fifty-four chloroplast homologue (cpsrp54/ffc) mutants (Amin et al. 1999). As chlorophylls were severely reduced, it was suggested that the accumulated LhcB2 are apoproteins in pga4-1 LhcB2-GFP.
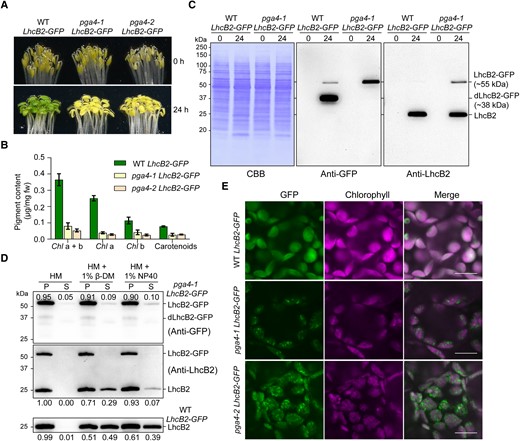
Loss of cpSRP54 affects the translocation of LhcB2-GFP to thylakoid membranes in A. thaliana. A) Phenotypes of etiolated and 24-h de-etiolated WT LhcB2-GFP, pga4-1 LhcB2-GFP, and pga4-2 LhcB2-GFP. B) The accumulation of photosynthetic pigments in 24-h de-etiolated seedlings shown in (A). Error bars represent s.d. from 3 biological replicates. Chl a + b, total chlorophyll concentration; Chl a, chlorophyll a concentration; Chl b, chlorophyll b concentration. C) The accumulation of LhcB2-GFP and dLhcB2-GFP in 24-h de-etiolated WT LhcB2-GFP and pga4-1 LhcB2-GFP. Protein loading was normalized to equal fresh tissue weight and confirmed by a Coomassie Brilliant Blue-stained PVDF membrane (CBB). D) The solubility of LhcB2-GFP and LhcB2 in 24-h de-etiolated pga4-1 LhcB2-GFP. Mild nonionic detergents 1% β-DM and 1% NP40 were used to solubilize membrane proteins. The anti-GFP antibody and the anti-LhcB2 antibody were used, respectively. The accumulation of LhcB2-GFP and LhcB2 in the P and S fractions was relatively quantified with signal intensities. E) The detection of GFP and chlorophyll fluorescence in 24-h de-etiolated WT LhcB2-GFP, pga4-1 LhcB2-GFP, and pga4-2 LhcB2-GFP. DIC for differential interference contrast images. Bars, 10 μm.
We next examined the solubility of LhcB2-GFP and LhcB2 in 24-h de-etiolated pga4-1 LhcB2-GFP. Most of LhcB2-GFP (about 90%) may present as protein aggregates in the P fraction after 1% β-DM or 1% NP40 treatment in de-etiolated pga4-1 LhcB2-GFP (Fig. 4D). Similarly, a higher relative level of detergent-resistant LhcB2 (71% with 1% β-DM, 93% with 1% NP40) was accumulated in pga4-1 LhcB2-GFP, in comparison with that (51% with 1% β-DM, 61% with 1% NP40) in WT LhcB2-GFP (Fig. 4D). The level of detergent-dissolved LhcB2 in WT LhcB2-GFP is higher than that in pga4-1 LhcB2-GFP (Fig. 4D). These indicated that both LhcB2-GFP and endogenous LhcB2 were clients of cpSRP54 for thylakoid membrane targeting, and cpSRP54 prevents their aggregations.
As LhcB2-GFP was highly detected in pga4-1 LhcB2-GFP, we next checked the localization of LhcB2-GFP in chloroplasts. The GFP fluorescence in 24-h de-etiolated seedlings was monitored with a confocal microscope. Surprisingly, it was shown that LhcB2-GFP formed discrete foci surrounding chlorophyll fluorescence in 24-h de-etiolated pga4-1 LhcB2-GFP and pga4-2 LhcB2-GFP (Fig. 4E). These discrete foci supported that over-accumulated LhcB2-GFP formed protein aggregates in pga4 LhcB2-GFPs in vivo. These together indicated that cpSRP54 is involved in targeting the chimeric LhcB2-GFP to thylakoids, where potential proteases for the N-terminal degradation of LhcB2-GFP to dLhcB2-GFP are localized.
Thylakoid FtsH protease is required for LhcB2-GFP degradation
Thylakoid FtsH is the most abundant AAA protease on thylakoid membranes. Reduced activity of thylakoid FtsH caused by mutations in VAR2/AtFtsH2 led to var2 alleles with different extents of variegated rosette leaves. The var2-5 is a weaker allele in leaf variegation compared with the var2-4 (Chen et al. 2000; Yu et al. 2004). To find out if thylakoid FtsH is involved in the degradation of LhcB2-GFP, we obtained var2-4 LhcB2-GFP and var2-5 LhcB2-GFP via genetic crosses. In contrast to the weaker allele var2-5, var2-4 LhcB2-GFP showed a visible pale-green phenotype at 24-h de-etiolation, similar to that observed in pga4 LhcB2-GFPs (Figs. 5, A and B, and 4A). At the transcript level, it was shown that both LhcB2 and LhcB2-GFP were upregulated by 24-h illumination in all 3 lines (Fig. 5C).
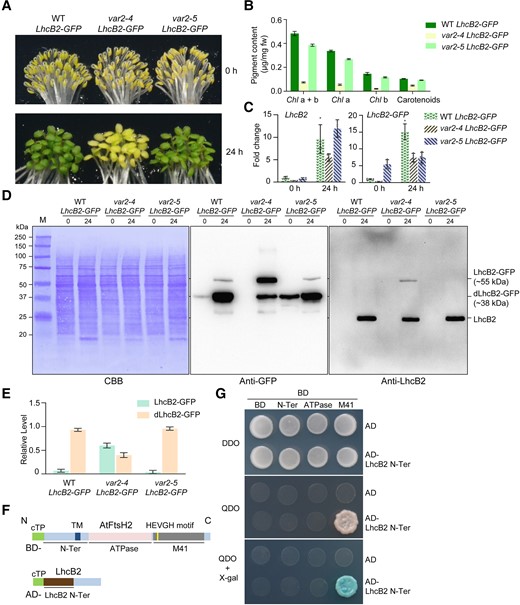
Thylakoid FtsH is required for the N-terminal degradation of LhcB2-GFP during de-etiolation. A) Phenotypes of etiolated and 24-h de-etiolated A. thaliana WT LhcB2-GFP, var2-4 LhcB2-GFP, and var2-5 LhcB2-GFP. B) The accumulation of photosynthetic pigments in 24-h de-etiolated seedlings shown in (A). Error bars represent s.d. from 3 biological replicates. Chl a + b, total chlorophyll concentration; Chl a, chlorophyll a concentration; Chl b, chlorophyll b concentration. C) The accumulation of LhcB2 and LhcB2-GFP transcripts in etiolated and 24-h de-etiolated seedlings shown in (A). RT-qPCR analyses were performed, and fold changes were calculated using PP2A as the reference gene. Data are means ± s.d. of 4 biological replicates. D) The accumulation of LhcB2-GFP and dLhcB2-GFP in 24-h de-etiolated WT LhcB2-GFP, var2-4 LhcB2-GFP, and var2-5 LhcB2-GFP. Protein loadings were normalized to equal fresh tissue weight and confirmed by a Coomassie Brilliant Blue-stained PVDF membrane (CBB). E) Quantitative analyses of the relative level of LhcB2-GFP and dLhcB2-GFP in (D). The total signal intensity of LhcB2-GFP and dLhcB2-GFP detected by the anti-GFP antibody was defined as 1.0 in each genotype. Error bars represent s.d. from 2 biological replicates. F) Schematic representation of the N-terminus of LhcB2 (LhcB2 N-Ter, used as prey, fused to AD) and domains of VAR2/AtFtsH2 (used as baits, fused to BD) for yeast two-hybrid assays. The LhcB2 N-Ter was deduced from the relative size of dLhcB2-GFP. cTP for chloroplast transit peptide, TM for the putative transmembrane domain, ATPase for the ATPase domain, and M41 for the zinc-dependent protease domain of VAR2/AtFtsH2. AD, the DNA-Activation Domain vector; BD, the DNA-Binding Domain vector. G) The M41 domain of VAR2/AtFtsH2 interacts with the N-terminus of LhcB2 in the yeast two-hybrid assay. DDO, double dropout SD medium lacking leucine and tryptophan; QDO, quadruple dropout SD medium lacking adenine, histidine, leucine, and tryptophan. QDO plates supplemented with X-α-Gal for a higher stringency.
We further analyzed the accumulation of LhcB2-GFP and dLhcB2-GFP in 24-h de-etiolated WT LhcB2-GFP, var2-4 LhcB2-GFP, and var2-5 LhcB2-GFP. Less dLhcB2-GFP and more LhcB2-GFP were accumulated in 24-h de-etiolated var2-4 LhcB2-GFP compared with those in WT LhcB2-GFP and var2-5 LhcB2-GFP (Fig. 5D). The relative accumulation ratio of dLhcB2-GFP and LhcB2-GFP was further quantified using the immunoblotting signal intensities (Fig. 5E). To further confirm thylakoid FtsH is involved in the N-terminal degradation of LhcB2-GFP, we performed yeast two-hybrid assays using the N-terminus of LhcB2-GFP as a prey, and different domains of VAR2/AtFtsH2 as baits (Fig. 5F). It was shown that the N-terminus of LhcB2-GFP interacted with the M41 protease domain of VAR2/AtFtsH2 in yeasts (Fig. 5G). These together indicated that thylakoid FtsH is the most likely protease involved in the degradation of LhcB2-GFP to dLhcB2-GFP.
The ATPase domain determines the degradation of LhcB2-GFP to dLhcB2-GFP
The AAA family proteases use ATPase domains to unfold substrate protein for sequential degradation achieved by its protease domains. All reported amino acid substitution mutation in var2 alleles occurs in the ATPase domain of VAR2/AtFtsH2 (Sakamoto et al. 2004; Zhang et al. 2010). Although both var2-5 (P320L) and var2-3 (G267D) contain missense mutations in the ATPase domain of VAR2/AtFtsH2, the var2-3 showed a more severe leaf variegation phenotype compared with the var2-5 (Chen et al. 2000). This prompted us to investigate the role of the ATPase domain in the degradation of LhcB2-GFP using var2-5 and var2-3 and its impact on plant phenotype (Fig. 6). At 24-h de-etiolation, the var2-3 mutant showed a conspicuous pale-green phenotype, resembled the null mutant var2-4 (Fig. 6A). We further analyzed the accumulation of VAR2/AtFtsH2 in those var2 alleles during 24-h de-etiolation (Fig. 6B). Consistent with previous findings in rosette leaves (Chen et al. 2000), all 3 var2 alleles had reduced levels of VAR2/AtFtsH2 compared with WT at 24-h de-etiolation (Fig. 6B). Although the var2-3 contained a relatively higher level of VAR2/AtFtsH2 than the var2-5, it showed a more severe pale-green phenotype than var2-5 during 24-h de-etiolation (Fig. 6, A and B). This indicated that AtFtsH2G267D in var2-3 was less active compared with AtFtsH2P320L in var2-5. In var2-3 LhcB2-GFP at 24-h de-etiolation, a higher ratio of LhcB2-GFP/dLhcB2-GFP was also observed, resembling that in var2-4 LhcB2-GFP (Fig. 6, C and D, and Supplemental Fig. S6). This indicated that the ATPase domain of VAR2/AtFtsH2 determines the degradation of LhcB2-GFP to dLhcB2-GFP.

The ATPase domain is required for the degradation of LhcB2-GFP. A) The phenotype of 24-h de-etiolated A. thaliana WT, var2-3, var2-4, and var2-5. B) The accumulation of VAR2/AtFtsH2 in 24-h de-etiolated WT, var2-3, var2-4, and var2-5. C) The accumulation of LhcB2-GFP and dLhcB2-GFP in 24-h de-etiolated WT LhcB2-GFP, var2-3 LhcB2-GFP, var2-4 LhcB2-GFP, and var2-5 LhcB2-GFP. The uncropped original figures are shown in Supplemental Fig. S6. D) The relative levels of LhcB2-GFP and dLhcB2-GFP in (C).
The accumulated LhcB2-GFP in var2-3 and var2-4 also form aggregates
We next examined the accumulation of LhcB2-GFP in cotyledons of var2-3 LhcB2-GFP, var2-4 LhcB2-GFP, and var2-5 LhcB2-GFP in vivo (Fig. 7A). In WT LhcB2-GFP, the GFP fluorescence was dispersed in chloroplasts and overlapped with the chlorophyll fluorescence, indicating the chloroplast localization of LhcB2-GFP and dLhcB2-GFP (Fig. 7A). Consistent with those observed in pga4 LhcB2s, GFP fluorescence was detected as discrete foci surrounding chlorophyll fluorescence in 24-h de-etiolated var2-3 LhcB2-GFP and var2-4 LhcB2-GFP (Figs. 7A and 4E). However, little foci were observed in the weaker allele var2-5 LhcB2-GFP (Fig. 7A).
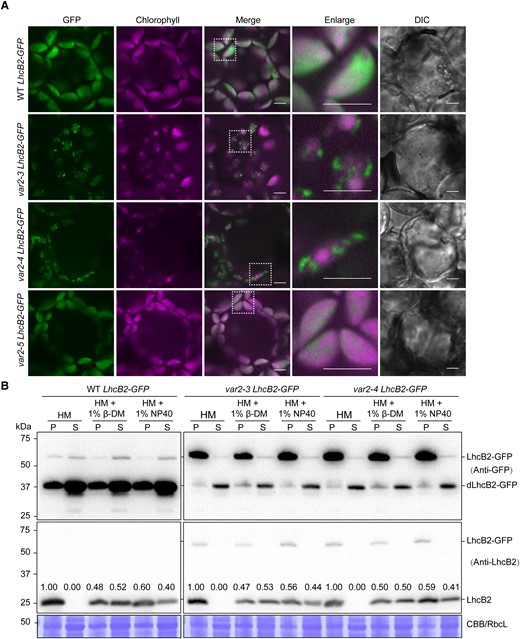
The accumulated LhcB2-GFP in var2-3 and var2-4 form aggregates. A) The detection of GFP and chlorophyll fluorescence in 24-h de-etiolated A. thaliana WT LhcB2-GFP, var2-3 LhcB2-GFP, var2-4 LhcB2-GFP, and var2-5 LhcB2-GFP. DIC for differential interference contrast images. White boxes indicated enlarged areas. Bars, 5 μm. B) The solubility of LhcB2-GFP in 24-h de-etiolated WT LhcB2-GFP, var2-3 LhcB2-GFP, and var2-4 LhcB2-GFP. Two mild nonionic detergents n-dodecyl-β-D-maltoside (1% β-DM) and Nonidet P-40 (1% NP40) were used to solubilize membrane proteins. The anti-GFP antibody and the anti-LhcB2 antibody were used, respectively. The accumulation of LhcB2 in the P and S fractions was relatively quantified with signal intensities. The stroma protein RbcL was shown by the Coomassie Brilliant Blue-stained PVDF membrane (CBB). S, the soluble fraction; P, the insoluble fraction.
To reveal the nature of these GFP aggregates, we tried chloroplast fractionation using 24-h de-etiolated seedlings of WT LhcB2-GFP, var2-3 LhcB2-GFP, and var2-4 LhcB2-GFP (Fig. 7B). In WT LhcB2-GFP, LhcB2 was present in the P fraction, or partially solubilized in the S fraction by using either 1% β-DM or 1% NP-40 (Fig. 7B). Meanwhile, a large amount of dLhcB2-GFP was present in the S fraction in the HM buffer or HM buffer containing detergents, indicating that they were mainly in the soluble stroma fraction (Figs. 7B and 3B). Consistently, partial LhcB2-GFP was solubilized when nonionic detergents were used in WT LhcB2-GFP, indicating LhcB2-GFP was associated with thylakoid membranes (Figs. 7B and 3B). In contrast, most of the accumulated LhcB2-GFP in var2-3 LhcB2-GFP and var2-4 LhcB2-GFP were resistant to nonionic detergents (Fig. 7B). There were still some dLhcB2-GFP and endogenous LhcB2 that were not solubilized by nonionic detergents (Figs. 7B and 3B). The ratio of detergent-dissolved LhcB2 to detergent-resistant LhcB2 was not affected in var2-3 LhcB2-GFP or var2-4 LhcB2-GFP compared with that in the WT LhcB2-GFP (Fig. 7B). These together indicated that LhcB2-GFP are prone to form protein aggregates in both var2 LhcB2-GFPs and pga4 LhcB2-GFPs.
Other chloroplast proteases are involved in the accumulation of LhcB2-GFP
Chloroplasts contain numerous proteases for chloroplast PQC (Nishimura et al. 2017; Sun et al. 2021). We checked if other chloroplast proteases are involved in the accumulation of LhcB2-GFP and degradation of LhcB2-GFP to dLhcB2-GFP, including the ATP-independent metalloprotease Ethylene-dependent Gravitropism-deficient and Yellow-green1 (EGY1) (Chen et al. 2005), a putative thylakoid-localized M41 metalloprotease Virescent3 (VIR3) (Qi et al. 2016), the chloroplast envelope-localized AtFtsH12 (Kikuchi et al. 2018), and the stroma-localized serine protease Casein lytic proteinase (Clp) (Bouchnak and van Wijk 2022). Accordingly, we crossed WT LhcB2-GFP with related mutants evr3-1 (Qi et al. 2020), vir3-1, pga1-1 (a hypomorphic allele of AtFtsH12) (Li et al. 2022), and clpr1-2 (Koussevitzky et al. 2007). During 24-h de-etiolation, the accumulation of LhcB2-GFP and dLhcB2-GFP varied in those mutants (Supplemental Fig. S7). Loss of thylakoid proteases EGY1/EVR3 or VIR3 does not affect the degradation of LhcB2-GFP to dLhcB2-GFP (Supplemental Fig. S7). Interestingly, the accumulation of both LhcB2-GFP and dLhcB2-GFP was severely reduced in pga1-1 and clpr1-2. The reduced accumulation of LhcB2-GFP in pga1-1 was consistent with a previous observation using chloroplast-targeting GFP driven by the chloroplast transit peptide of Ferredoxin-NADP+ Reductase (Li et al. 2022). Previous studies showed that AtFtsH12 is involved in the translocation of chloroplast proteins (Kikuchi et al. 2018). The negative genetic interaction between pga1-1 and clpr1-2 indicated a possible synergistic function between AtFtsH12 and ClpR1 in chloroplast protein homeostasis (Li et al. 2022). However, the mechanism(s) for the reduced accumulation of LhcB2-GFP and dLhcB2-GFP in pga1-1 and clpr1-2 is still not well elucidated.
PGA4 is a suppressor locus of var2 leaf variegation
As both FtsH and cpSRP54 are involved in the degradation of LhcB2-GFP, we analyzed the genetic interaction between pga4-1 and var2 mutants in this process. Moreover, the pga4-2 mutant was obtained from an activation tagging mutagenesis pool of var2-5, indicating that PGA4 is likely a new suppressor locus for the leaf variegation phenotype of var2. The single mutant pga4-1 showed pale-green rosette leaves, while var2 mutants showed variegated rosette leaves (Fig. 8, A to C). The double mutant var2-5 pga4-1 was obtained, in which the leaf variegation of var2-5 was masked by pga4-1 (Fig. 8A). In addition, pga4-1 could also suppress var2-4 (Fig. 8B). The binary vector ProcpSRP54:cpSRP54-GFP was used to recover the double mutant var2-4 pga4-1 to var2-4 (Fig. 8C). These confirmed that the pga4-1 mutant is a genetic suppressor of var2.
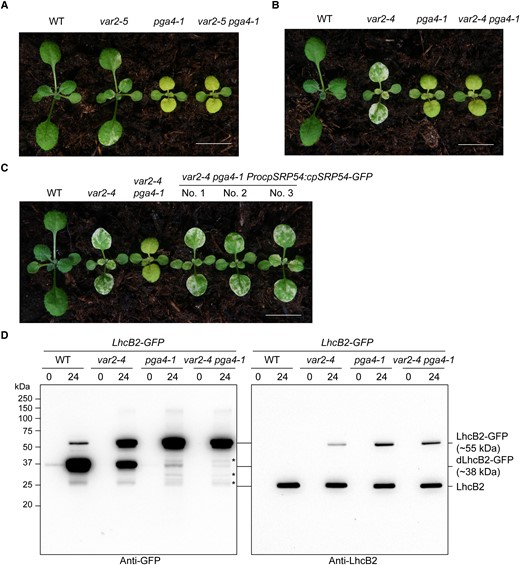
The A. thaliana pga4-1 mutant is a var2 suppressor. A) Representative WT, var2-5, pga4-1, and var2-5 pga4-1. B) Representative WT, var2-4, pga4-1, and var2-4 pga4-1. C) Complementation of the double mutant var2-4 pga4-1 to var2-4 with the binary construct ProcpSRP54:cpSRP54-GFP. Representative 2-wk-old seedlings were shown. Bars, 1.0 cm in (A–C). D) The accumulation of LhcB2-GFP and dLhcB2-GFP in 24-h de-etiolated WT LhcB2-GFP, var2-4 LhcB2-GFP, pga4-1 LhcB2-GFP, and var2-4 pga4-1 LhcB2-GFP. Asterisks indicate other possible degraded LhcB2-GFP products in pga4-1 LhcB2-GFP and var2-4 pga4-1 LhcB2-GFP.
We further checked the accumulation of LhcB2-GFP and dLhcB2-GFP in the double mutant var2-4 pga4-1 LhcB2-GFP during de-etiolation. The accumulation of dLhcB2-GFP was even more reduced in var2-4 pga4-1 LhcB2-GFP when compared with that in pga4-1 LhcB2-GFP (Fig. 8D). This suggested that PGA4 is epistatic to VAR2/AtFtsH2 in the degradation of LhcB2-GFP and the formation of leaf variegation. Interestingly, we also observed some other faint degraded products of LhcB2-GFP, which became relatively obvious in pga4-1 LhcB2-GFP and var2-4 pga4-1 LhcB2-GFP (Fig. 8D). The accumulation of those degraded products was comparable between pga4-1 LhcB2-GFP and var2-4 pga4-1 LhcB2-GFP, indicating the existence of other proteases to clean LhcB2-GFP aggregates.
Discussion
Using the dysfunctional LhcB2-GFP as a heterogeneous marker to understand the translocation of thylakoid membrane proteins
The light-harvesting chlorophyll a/b binding (LHC) proteins, encoded by the nuclear genome, are the most abundant thylakoid membrane proteins (Schünemann 2004). The expression of LHCPs was used as markers for understanding the anterograde and retrograde regulation of chloroplast development (Susek et al. 1993; Jarvis and Lopez-Juez 2013). To understand chloroplast protein homeostasis, we systematically isolated pale green Arabidopsis (pga) mutants (Li et al. 2022). In this work, we reported that PGA4 encodes cpSRP54 (Fig. 1). To further dissect the function of cpSRP54 in chloroplast protein homeostasis, we generated a stable transgenic marker line to express a chimeric LhcB2-GFP resembling the endogenous LhcB2 (Fig. 2). Our biochemical assays showed that the chimeric LhcB2-GFP is targeted into thylakoid membranes during de-etiolation (Fig. 3). Interfered by the tightly folded GFP, the membrane-associated LhcB2-GFP is not able to be incorporated into LHCII complexes and further degraded to a predominant form dLhcB2-GFP in WT LhcB2-GFP (Figs. 2 and 3). Nevertheless, this chimeric LhcB2-GFP and the degraded form dLhcB2-GFP provided a heterogeneous unassembled protein marker for protein translocation to thylakoids. Using pga4 LhcB2-GFPs, we demonstrated that both cpSRP54-dependent and cpSRP54-independent translocation pathways exist in cotyledons, and the former might be functional predominantly for LhcB2-GFP.
Surprisingly, the dLhcB2-GFP is sharply reduced in pga4-1 LhcB2-GFP compared with those in WT LhcB2-GFP (Fig. 4). Moreover, the accumulated LhcB2-GFP formed insoluble protein aggregates and discrete GFP fluorescent foci in pga4 mutants. Without chlorophyll pigments, some of those accumulated LhcB2 could be aggregated apoproteins, of which the proportion being insoluble to mild detergents in pga4-1 LhcB2-GFP is relatively higher than those in WT LhcB2-GFP. These provided in vivo evidence that cpSRP54 could prevent aggregations of LHC apoproteins (Schünemann et al. 1998; Yuan et al. 2002; Goforth et al. 2004). The translocation of unassembled LhcB2-GFP to thylakoid membranes is a prerequisite for its thylakoid-associated degradation to dLhcB2-GFP, and this scenario may explain the discrepancy in accumulations of photosynthetic pigments and endogenous LhcB2 during de-etiolation in pga4-1 LhcB2-GFP. However, the degradation of insoluble endogenous LhcB2 in pga4-1 LhcB2-GFP requires further experimental support, as we could not observe any LhcB2 C terminal fragments using the LhcB2 antibody. Although we did not have direct evidence to support that the degradation of LhcB2-GFP is consistent with the endogenous LhcB2, the discrete LhcB2-GFP fluorescent foci in pga4-1 LhcB2-GFP could be used as a heterogeneous marker to dissect the regulatory network in chloroplast PQC interconnecting with cpSRP54.
Using the degradation of LhcB2-GFP to dLhcB2-GFP to dissect chloroplast PQC during de-etiolation
FtsH is a prevailing membrane-localized AAA family protease that can degrade misfolded or unassembled polypeptides for membrane PQC in prokaryotes and organelles of eukaryotes (Janska et al. 2013). In photosynthetic species, it is generally accepted that the photo-damaged core protein D1 of PSII complexes is a bona fide substrate of thylakoid FtsH proteases (Nixon et al. 2010; Kato et al. 2012). However, our understanding of FtsH-mediated thylakoid membrane PQC in land plants during de-etiolation is still limited. The var2 mutants, with green cotyledons when grown under normal conditions, showed conspicuous delayed de-etiolation phenotypes, indicating the indispensable role of FtsH in thylakoid membrane PQC during de-etiolation. Our genetic and biochemical evidence strongly supported that thylakoid FtsH is involved in the degradation of LhcB2-GFP to dLhcB2-GFP during de-etiolation. The use of different var2 alleles further indicated that the ATPase activity of thylakoid FtsH determines the degradation of LhcB2-GFP.
We showed that thylakoid FtsH degrades the LhcB2-GFP to dLhcB2-GFP, providing a trackable marker protein to dissect FtsH-mediated thylakoid membrane PQC during de-etiolation. The degradation of this heterogeneous substrate LhcB2-GFP supported that cpSRP54 and thylakoid FtsH complex work sequentially for thylakoid membrane PQC (Fig. 9). Similar to those in pga4-1 LhcB2-GFP, endogenous LhcB2 was highly accumulated in var2-4 LhcB2-GFP and var2-4 pga4-1 LhcB2-GFP. Thus, it is reasonable to assume that the rapid turnover of misfolded or unassembled subunits is essential to avoid the formation of toxic aggregates during de-etiolation because thylakoid membrane-localized photosynthetic proteins are mostly hydrophobic. Moreover, we observed that the LhcB2-GFP was degraded to other types of fragments in pga4-1 LhcB2-GFP and var2-4 pga4-1 LhcB2-GFP, supporting that some stroma-localized chaperones or proteases were also involved in degrading LhcB2-GFP or dLhcB2-GFP aggregates. It was observed that the accumulation of Clp, Chaperonin 60 (CPN60), and chloroplast Heat Shock Protein 70 (cpHSP70) was increased in cpspr54/ffc or var2 mutants (Amin et al. 1999; Rutschow et al. 2008; Dogra et al. 2019). These may participate in the degradation of misfolded/unassembled membrane proteins that accumulated in the stroma, possibly compensating for the loss of cpSRP54 and FtsH.
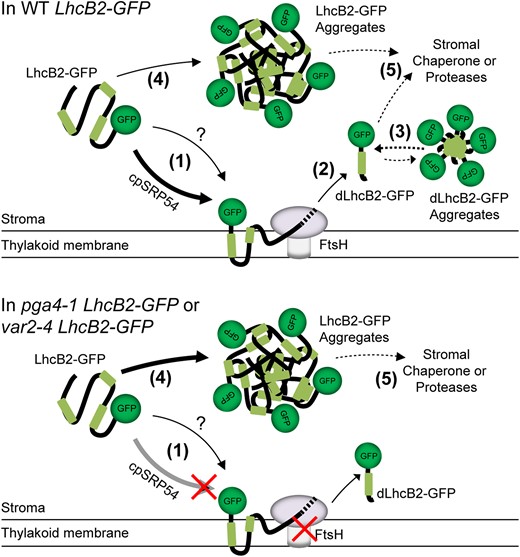
A working model showing A. thaliana cpSRP54 and thylakoid FtsH modulates N-terminal degradation of LhcB2-GFP. In WT LhcB2-GFP, LhcB2-GFP is translocated to the thylakoid membrane via the SRP54-dependent pathway (1) and degraded by the thylakoid FtsH. The question mark indicates SRP54-independent translocation pathways. When encountering the stable GFP structure, FtsH stops degradation and releases dLhcB2-GFP to stroma (2). The released dLhcB2-GFP may form dLhcB2-GFP aggregates (3). In pga4-1 LhcB2-GFP or var2-4 LhcB2-GFP, disruption of either the cpSRP54-dependent translocation or the thylakoid FtsH activity could prevent the degradation of LhcB2-GFP to dLhcB2 on thylakoid membrane, which in turn lead to the formation of LhcB2-GFP aggregates in the stroma (4). Other chaperones or proteases may be involved in the degradation of LhcB2-GFP or dLhcB2-GFP aggregation in the stroma (5).
The partially degraded LhcB2-GFP indicates the N-terminal processing activity of thylakoid FtsH
The most characterized degradation process mediated by thylakoid FtsH is the D1 degradation during the PSII repair cycle under high light conditions. FtsH is required for the processive degradation of the full D1 or the N-terminal fragments of D1 generated by Deg proteases (Komenda et al. 2007; Kato et al. 2012, 2018). var2 mutants contain high levels of full D1 under high light stress (Bailey et al. 2002; Kato et al. 2012; Qi et al. 2020; Wang et al. 2022). In cyanobacteria, the N-terminal D1 sequence and FtsH proteases determine the stability of D1 (Komenda et al. 2007). Similar to D1 degradation, the degradation of LhcB2-GFP to dLhcB2-GFP is initiated at its N-terminus but ceased before approaching the stable structure of GFP protein. Thus, the accumulation of LhcB2-GFP and dLhcB2-GFP provided an easy-trackable substrate and product to analyze the N-terminal degradation of thylakoid FtsH under nonphotoinhibitory light conditions for future studies.
Instead of being fully degraded, the accumulation of dLhcB2-GFP fragments raises a fundamental question if thylakoid FtsH proteases have protein processing activity at physiological conditions (Okuno and Ogura 2013). Other FtsH homologs from E. coli and yeast mitochondria can not only degrade 1 entire protein processively but also perform protein modifications by protein N or C terminal processing in vitro or in vivo (Okuno et al. 2006; Walker et al. 2007; Bonn et al. 2011; Chauleau et al. 2011). The remaining dLhcB2-GFP demonstrated the potential N-terminal processing activity of thylakoid FtsH, which is consistent with their prokaryotic homologs. If this N-terminal processing activity is conserved in the thylakoid FtsH, 1 plausible scenario is that thylakoid FtsH is required for processing, instead of full degradation of certain proteins that are essential for thylakoid membrane biogenesis and chloroplast development. Although the FtsH is one of the best characterized AAA family proteases, we still know little about its potential substrates during de-etiolation, and how it determines chloroplast development during de-etiolation. Future work on the identification of thylakoid FtsH substrates will address this point.
Reduced thylakoid membrane protein loading may alleviate the var2 leaf variegation phenotype
One intriguing feature of var2 mutants is the variegated rosette leaves with normal-appearing green sectors and albino sectors with abnormal chloroplasts. Systematic identification of genetic modifiers of the variegation phenotype of var2 unraveled numerous loci that interact with VAR2/AtFtsH2 genetically. The identification of SVR8/RPL24, SVR9/IF3, and SVR11/EF-Tu indicated that weakened chloroplast translation could suppress the leaf variegation of var2 (Liu et al. 2013; Zheng et al. 2016; Liu et al. 2019). Nonetheless, the molecular mechanism is still not well understood. Here, we found that pga4 mutants are var2 suppressors (Fig. 8). Besides the translocation of LHC proteins to thylakoid membranes, it was reported recently that cpSRP54 interacts with chloroplast ribosomes to navigate the insertion of de novo synthesized nascent proteins, such as the PSII reaction center protein D1 (Ziehe et al. 2018; Hristou et al. 2019). Given the fact that a group of SVRs are involved in chloroplast translation, it was deduced that the defects in cpSRP54-mediated translocation of chloroplast-translated nascent proteins caused suppression of the var2 leaf variegation. In addition, D1 is vulnerable to light, and the thylakoid FtsH is involved in the degradation of photo-damaged D1 (Nixon et al. 2010). The identification of pga4-1 provided us with a scenario that cpSRP54 and thylakoid FtsH complex may work coordinately to the membrane protein loading and degradation balance, especially the 2 most abundant pigment-binding photosynthetic proteins LhcB2 and D1, and this membrane protein homeostasis may further influence the leaf variegation phenotype and chloroplast development defects in var2 mutants.
Taken together, we presented a model that cpSRP54 and thylakoid FtsH could determine the N-terminal degradation of LhcB2-GFP sequentially on the thylakoid membrane (Fig. 9). In WT LhcB2-GFP, the heterogeneous LhcB2-GFP will be targeted to thylakoid membranes predominantly by the cpSRP54-dependent protein translocation pathway (1). The chimeric LhcB2-GFP is dysfunctional and subjected to N-terminal degradation mediated by thylakoid FtsH. The FtsH degrades LhcB2-GFP, initiated at the N-terminus and ceases before approaching the stable GFP protein, and releases the dLhcB2 into the stroma (2). The ATPase domain of FtsH provides essential driving forces to unfold LhcB2-GFP for further degradation performed by the protease domain. Alternatively, the lower abundance of dLhcB2-GFP in the SRP54 null mutant pga4-1 LhcB2-GFP indicated that a minor fraction of LhcB2-GFP is transported to thylakoid membranes by cpSRP54-independent pathways. The stroma-localized LhcB2-GFP and dLhcB2 may form protein aggregates, resistant to mild nonionic detergents (3 and 4). In pga4 LhcB2-GFPs or var2 LhcB2-GFPs, the mutations of either cpSRP54-dependent translocation, or the activity of thylakoid FtsH could prevent the degradation of LhcB2-GFP to dLhcB2, which in turn form protein aggregates. Also, some stromal chaperones or proteases would be involved in the complete degradation of LhcB2-GFP and dLhcB2-GFP to free amino acids to maintain chloroplast protein homeostasis (5).
Materials and methods
Plant materials and growth conditions
All Arabidopsis (Arabidopsis thaliana) strains used in this study are the Columbia ecotype (Col), except for the Landsberg erecta ecotype (Ler) to generate the mapping population. Arabidopsis mutants var2-3, var2-4, var2-5, pga1-1, vir3-1, evr3-1, clpr1-2 are described in results. The pga4-1 mutant was isolated from an EMS mutagenesis pool and backcrossed with Col 7 times. The pga4-2 mutant was recovered from an activation tagging mutagenesis pool in var2-5 background and backcrossed with Col 3 times (Yu et al. 2008). Seeds were surface sterilized and stratified at 4 °C for 2 d before planting. For de-etiolation assay, sterilized seeds were grown on 1/2 MS media containing 1% (w/v) Bacto agar in the dark for 3 d at 22 °C and transferred to continuous light (∼100 µmol m−2 s−1 photons) in a growth chamber for indicated periods. For chemical treatments, seedlings were grown on 1/2 MS media containing 0.5 mM lincomycin (Lin) or 5 μM norflurazon (NF), respectively. Plants for other purposes were grown on commercial soil mix (Pindstrup) under continuous light (∼100 µmol m−2 s−1 photons) at 22 °C.
Map-based cloning of PGA4
Map-based cloning was performed to identify the PGA4 locus. The pga4-1 mutant was crossed with the Ler ecotype to generate the F2 mapping population. Bulked segregant analysis located PGA4 to a region near the marker F8F6#1 on chromosome 5. Additional molecular markers based on Indel or SNP polymorphisms were designed for fine mapping.
Construction of vectors and generation of transgenic lines
Genomic sequences of AtLhcB2.2 with endogenous promoter regions (2089 bp), and genomic sequences of cpSRP54 with endogenous promoter regions (1353 bp), were cloned into the binary vector pCambia1300-GFP to generate ProLhcB2:gLhcB2-GFP and ProcpSRP54:cpSRP54-GFP. Transgenic lines were generated by the Agrobacterium tumefaciens-mediated floral dip method (Clough and Bent 1998). Transgenic lines were screened on 1/2 MS media containing 25 mg L−1 hygromycin. The var2 or pga4 LhcB2-GFP lines were obtained by genetic crosses with WT LhcB2-GFP, and F3 generations carrying the hygromycin-resistant marker without segregation were used for experiments. Primers for vectors and genotyping are listed in Supplemental Table S1.
RNA extraction and quantitative RT-PCR (RT-qPCR)
Total RNAs were extracted from de-etiolated seedlings using the TRIzol reagent (15596026, Thermo Fisher Scientific). cDNAs were synthesized from 1.0 μg total RNA with the UEIris RT mix with DNase (All-in-one) (R2020, US Everbright). qPCRs were performed using 2 × ChamQ SYBR qPCR Master Mix (Q311-02, Vazyme). Primers for RT-qPCRs are listed in Supplemental Table S1.
Protein extraction and immunoblotting analyses
The separation of soluble and membrane fractions was performed as described (Hou et al. 2021), with some modifications. De-etiolated seedlings were weighed and ground in Hepes-MgCl2 (HM) buffer (10 mM Hepes-KOH pH7.6, 5 mM MgCl2), with or without 2 M NaCl, 1% (v/v) n-dodecyl-β-D-maltoside, or 1% (v/v) Nonidet P-40 if required. Supernatant (S) and pellet (P) were separated by centrifugation at 20,000 × g for 15 min at 4 °C. Pellet was further resuspended with the same volume of HM buffer. Both S and P fractions were diluted with the same volume of 2 × SDS sample buffer (0.125 M Tris-HCl pH6.8, 4% (w/v) SDS and 20% (v/v) glycerol, 1% (v/v) β-mercaptoethanol) for further SDS-PAGE and immunoblotting. To extract total proteins, fresh plant seedlings were weighed and ground in liquid nitrogen and incubated with 2 × SDS sample buffer at 65 °C for 1 h. After centrifugation at 18,000 × g for 10 min, the supernatant was collected and loaded on 12% SDS-PAGE containing 8 M urea for electrophoresis. Protein loading was normalized to equal fresh tissue weight. After being transferred to PVDF membranes (0.22 µm, Millipore), immunoblotting analyses were performed with standard protocols. The anti-GFP antibody (TaKaRa, 632381), the anti-LhcB2 antibody (Agrisera, AS01003), the anti-VAR2/AtFtsH2 antibody (Qi et al. 2016), and the anti-RbcL antibody (Agrisera, AS03037) were used. The quantification of signal intensity was carried out by the Image Lab software (Bio-Rad).
Blue native PAGE
The preparation of BN-PAGE gel was performed as described (Järvi et al. 2011). De-etiolated WT LhcB2 seedlings were weighed and homogenized in liquid nitrogen. HM buffer was used to suspend the homogenate, and Supernatant (S) and pellet (P) were separated by centrifugation at 20,000 × g for 15 min at 4 °C. Thylakoid membranes from the pellet fraction were solubilized with 1% (w/v) β-DM in 25BTH20G buffer (25 mM Bis-Tris-HCl pH7.0 and 20% glycerol). The β-DM solubilized fraction was collected by centrifugation at 20,000 × g for 10 min at 4 °C and resolved on 3% to 10% 1D BN-PAGE gel. Excised gel lanes from the 1D BN-PAGE gel were incubated in 2 × SDS sample buffer with gentle shaking at room temperature for 30 min for denaturation. The 1D gel was further applied to 10% SDS-PAGE gel for 2D electrophoresis.
Recombinant protein expression in E. coli
The coding regions for LhcB2, ΔN-LhcB2, and LhcB2-ΔC were cloned into the pGEX4T-1 vector. Expression of GST-LhcB2, GST-ΔN-LhcB2, GST-LhcB2-ΔC in E. coli BL21 (DE3) was induced with 0.1 mM isopropylthio-β-galactoside (IPTG) at 37 °C for 4 h. Primers for the vector construction are listed in Supplemental Table S1.
Quantification of chlorophyll content
To measure the chlorophyll content, de-etiolated seedlings were harvested, weighed, and ground in liquid nitrogen. Chlorophyll was extracted by 95% (v/v) ethanol with gentle rotation for 24 h at 4 °C. Tissue debris was removed by centrifugation at 19,000 × g for 20 min at 4 °C. The content of chlorophyll and carotenoids was calculated as described (Wang et al. 2018b).
Yeast two-hybrid assays
The yeast (S. cerevisiae) strain Y2HGold and the Matchmaker Gold Yeast Two-Hybrid System (Clontech) were used for the yeast two-hybrid assay. The coding region for the N-terminus of LhcB2 without chloroplast transit peptide (amino acid residues 42 to 158) was cloned into pGADT7, the DNA-Activation Domain (AD) vector for prey. The coding regions for the N-terminal domain (amino acid residues 47–220), the ATPase domain (amino acid residues 221–466), and the M41 protease domain (amino acids residues 473–671) of VAR2/AtFtsH2 were cloned into pGBKT7, the DNA-Binding Domain (BD) vector for bait. The AD- and BD-vectors were co-transformed into Y2HGold cells and selected on the double dropout SD medium (DDO, lacking leucine and tryptophan). Protein interactions were verified by grown colonies on the quadruple dropout SD medium (QDO, lacking adenine, histidine, leucine, and tryptophan), and QDO medium supplemented with 100 µg mL−1 X-α-gal for high stringency. Primers are listed in Supplemental Table S1.
Confocal microscopy
GFP fluorescence and chlorophyll auto-fluorescence images were collected using a spinning disk confocal microscope (Revolution-XD, Andor) and a 100× oil immersion objective. The GFP fluorescence was excited at 488 nm and captured with a 525/50 bandpass emission filter. The Chlorophyll fluorescence was excited at 637 nm and captured with a 670/30 bandpass emission filter. The same excitation intensity and exposure time were used for different genotypes. The LhcB2-GFP lines were examined after 24-h de-etiolation, and cotyledons were used directly for confocal microscopy imaging. Images were processed by the Fiji ImageJ software.
Accession numbers
Sequence data used in this study can be found in The Arabidopsis Information Resource (TAIR) with the following accession numbers: LhcB2.2, AT2G05070; VAR2/AtFtsH2, AT2G30950; cpSRP54, AT5G03940; AtPP2A, AT1G69960.
Acknowledgments
We thank Dr Ningjuan Fan from the Teaching and Research Core Facility at the College of Life Sciences, Northwest A&F University, for technical assistance in using the confocal microscope.
Author contributions
Y.Q. and Y.L. conceived and designed research plans; Y.Q. and F.Y. supervised the experiments; Y.L., B.L., X.W., J.W., P.W., and J.Z. performed experiments; Y.Q. and F.Y. analyzed the data; Y.L. and Y.Q. wrote the manuscript with contributions from all the authors. Y.L. and B.L. contributed equally.
Supplemental data
The following materials are available in the online version of this article.
Supplemental Figure S1. Isolation of two pga4 mutants.
Supplemental Figure S2. Accumulation of cpSRP54-GFP in 24-h de-etiolated pga4-1 ProcpSRP54:cpSRP54-GFP.
Supplemental Figure S3. Supporting information for Figure 2.
Supplemental Figure S4. Supporting information for Figure 3.
Supplemental Figure S5. The LhcB2 antibody recognizes the N-terminus of LhcB2.
Supplemental Figure S6. Supporting information for Figure 6.
Supplemental Figure S7. Accumulation of LhcB2-GFP in other chloroplast protease mutants.
Supplemental Table S1. Primers used in this study.
Funding
This work was supported by grants from the National Natural Science Foundation of China (31970656 and 31741010 to Y.Q.).
Data availability
The data that support the findings of this study are available from the corresponding author upon reasonable request.
References
Author notes
These authors contributed equally to this article.
The author responsible for distribution of materials integral to the findings presented in this article in accordance with the policy described in the Instructions for Authors (https://dbpia.nl.go.kr/plphys/pages/General-Instructions) is Yafei Qi.
Conflict of interest statement. None declared.