-
PDF
- Split View
-
Views
-
Cite
Cite
Qi Luo, Wei Wei, Ying-ying Yang, Chao-jie Wu, Jian-ye Chen, Wang-jin Lu, Jian-fei Kuang, Wei Shan, E3 ligase MaNIP1 degradation of NON-YELLOW COLORING1 at high temperature inhibits banana degreening, Plant Physiology, Volume 192, Issue 3, July 2023, Pages 1969–1981, https://doi.org/10.1093/plphys/kiad096
- Share Icon Share
Abstract
Banana (Musa acuminata) fruit ripening under high temperatures (>24 °C) undergoes green ripening due to failure of chlorophyll degradation, which greatly reduces marketability. However, the mechanism underlying high temperature-repressed chlorophyll catabolism in banana fruit is not yet well understood. Here, using quantitative proteomic analysis, 375 differentially expressed proteins were identified in normal yellow and green ripening in banana. Among these, one of the key enzymes involved in chlorophyll degradation, NON-YELLOW COLORING 1 (MaNYC1), exhibited reduced protein levels when banana fruit ripened under high temperature. Transient overexpression of MaNYC1 in banana peels resulted in chlorophyll degradation under high temperature, which weakens the green ripening phenotype. Importantly, high temperature induced MaNYC1 protein degradation via the proteasome pathway. A banana RING E3 ligase, NYC1-interacting protein 1 (MaNIP1), was found to interact with and ubiquitinate MaNYC1, leading to its proteasomal degradation. Furthermore, transient overexpression of MaNIP1 attenuated MaNYC1-induced chlorophyll degradation in banana fruits, indicating that MaNIP1 negatively regulates chlorophyll catabolism by affecting MaNYC1 degradation. Taken together, the findings establish a post-translational regulatory module of MaNIP1–MaNYC1 that mediates high temperature-induced green ripening in bananas.
Introduction
Fruit color is a key sensory quality characteristic that influences freshness perception and consumer purchase intention, and it can be affected by environmental stimuli such as light, temperature, and humidity (Lee et al. 2014; Rolando et al. 2015). Chlorophyll degradation is the leading cause of fruit degreening, which in turn determines fruit skin color and external appearance (Santos 2004; Momose and Ozeki 2013). With global warming and climate change, heat stress has emerged as one of the most important causes of crop quality deterioration and yield loss. As a classical climacteric fruit, bananas (Musa acuminata, AAA group), the most popularly consumed fresh fruit worldwide, are usually harvested at the green mature stage, and then they are artificially ripened with ethylene (ETH) to become golden yellow in peels before marketing (Shan et al. 2020; Wu et al. 2022). However, when the ambient temperature increases above 24 °C, banana fruit fails to degreen, and the peels remain green even after the pulps have achieved fully softening, thereby leading to a green ripening phenotype (Seymour et al. 1987). Green ripened banana fruits are usually of poor quality and unmarketability, resulting in severe economic losses for the banana industry. This abnormal color development caused by high temperature is due to the suppression of chlorophyll degradation, and it is not related to a lack of carotenoid pigments (Blackbourn et al. 1990; Drury et al. 1999; Yang et al. 2009). However, the response of banana fruit to high temperature appears to be somewhat unusual compared with other fruits, such as tomato (Solanum lycopersicum), mango (Mangifera indica), and orange (Citrus sinensis), in which chlorophyll degradation and peel color change are promoted by heat stress (Ogura et al. 1975; Cohen 1978; Medlicott et al. 1986). In addition, when compared with pear (Pyrus communis) and avocado (Persea americana), which discolor as the temperature rises to 40 °C (Maxie et al. 1974; Eaks 1978), banana shows green ripening symptoms at a range of temperatures (>24 °C), which are theoretically not high enough to inhibit enzymatic reactions. Therefore, understanding the molecular regulation of inhibited chlorophyll catabolism during green ripening in bananas will help improve our understanding of heat stress on chlorophyll degradation in plants.
NON-YELLOW COLORING 1 (NYC1), an NADPH-dependent chlorophyll b reductase, plays an important role in chlorophyll catabolic pathway responsible for the conversion of chlorophyll b to chlorophyll a (Kusaba et al. 2007; Tanaka and Tanaka 2011). Functionally, NYC1 has been characterized in model plants such as Arabidopsis (Arabidopsis thaliana) and rice (Oryza sativa), where NYC1 gene expression knockdown leads to a stay-green phenotype with high chlorophyll b content (Sato et al. 2009; Jibran et al. 2015). As a key component of the chlorophyll catabolic enzyme system, the regulatory mechanism of NYC1 has received much attention over the past few years. For example, transcription factors (TFs) ETHYLENE-INSENSITIVE 3 (EIN3) and ORESARA1 (ORE1, an NAC TF) were identified as direct transcriptional activators of NYC1 during ETH-triggered chlorophyll degradation and senescence in leaves (Qiu et al. 2015). In abscisic acid (ABA)-induced leaf senescence, NYC1 is directly upregulated by several bZIP TFs, such as ABA-RESPONSIVE ELEMENT-BINDING FACTOR 2/3/4 (ABF2/3/4), ABA-INSENSITIVE 5 (ABI5), and ENHANCED EM LEVEL (EEL), subsequently leading to chlorophyll degradation (Sakuraba et al. 2014; Gao et al. 2016). In addition, MYC2/3/4 cooperate with NAC019/055/072 to target NYC1, which promotes chlorophyll degradation in jasmonate-induced leaf senescence (Zhu et al. 2015). In plants, developmentally regulated metabolic pathways are usually affected at multiple levels, including transcriptional, post-transcriptional, translational, and post-translational regulation (Dubos et al. 2010; Millard et al. 2019). Besides transcriptional regulation, the post-translational regulation of NYC1 is still unknown.
Ubiquitin (Ub) proteasome-mediated protein degradation plays a key role in post-translational regulation in the eukaryotic cell; it controls the selective degradation of intracellular proteins and participates in almost all life activities of plants and animals (Nandi et al. 2006; Xu and Xue 2019). The Ub-proteasome system (UPS) consists of Ub, Ub-activating enzyme (E1), Ub-conjugating enzyme (E2), Ub ligase (E3), and the 26S proteasome, among which E3 ligase is undoubtedly the most important component because of its specific recognition and ubiquitination of the substrate (Ji and Kwon 2017). Several E3 ligases along with their mediated protein ubiquitination and degradation play important regulatory roles in fruit quality formation and deterioration. For instance, tomato TF Golden2-like1 (SlGLK2), which determines chlorophyll accumulation and chloroplast development in fruit by inducing photosynthesis gene expression, is recognized by the Cullin4 (CUL4)-Damaged DNA Binding Protein 1 (DDB1)-based E3 ligase complex for proteasomal degradation (Tang et al. 2016). In addition, Plastid Protein Sensing RING E3 ligase 1 (PPSR1) interacts with Ub-conjugating enzyme E2 N (SlUBC32) to promote the ubiquitination and degradation of phytoene synthase 1 (PSY1), the main rate-limiting enzyme in the carotenoid biosynthetic pathway, thereby modulating carotenoid accumulation during fruit ripening (Wang et al. 2020). More importantly, light induces the red coloration in apple (Malus pumila) fruits by inhibiting CONSTITUTIVE PHOTOMORPHOGENIC 1-mediated ubiquitination and degradation of MdMYB1, an MYB TF that directly activates UDP-glucose flavonoid 3-O-glucosyl transferase (MdUFGT) and dihydroflavonol 4-reductase (MdDFR) genes to promote anthocyanin biosynthesis (Li et al. 2012; Kang et al. 2022), suggesting that E3 ligases are involved in the light regulation of apple color quality. In addition, some E3 ligases in rice and Arabidopsis play a key role in plant tolerance to cold and heat stresses (Liu et al. 2016; Kim et al. 2019; Wang et al. 2019; Xu and Xue 2019). E3 Ub ligase MYB30-INTERACTING E3 LIGASE 1 (MdMIEL1) negatively regulates both cold tolerance and anthocyanin accumulation in apple fruit by degrading MdMYB308L, thus inhibiting the activation of C-repeat-binding factor 2 (MdCBF2) and MdDFR genes by MdMYB308L (An et al. 2020). However, the direct involvement of E3 ligases in fruit quality under high temperature is still unknown.
In this study, quantitative proteomic analysis was performed, and many differentially expressed proteins were identified in normal yellow and green ripening banana fruits. The chlorophyll catabolic enzyme MaNYC1 exhibited a reduced protein level when banana fruit was green ripening at high temperature. Transient overexpression of MaNYC1 in banana peels promoted chlorophyll degradation under high temperature to weaken the green ripening phenotype. More importantly, MaNYC1 was ubiquitinated by the RING E3 ligase NYC1-interacting protein 1 (MaNIP1), causing MaNYC1 degradation via the 26S proteasome pathway. Furthermore, MaNIP1-mediated MaNYC1 degradation was facilitated under high temperature, thereby attenuating MaNYC1 promoted chlorophyll degradation. These findings unravel an important regulatory cascade involving the MaNIP1–MaNYC1 complex that modulates high temperature-induced chlorophyll degradation, which may explain green ripening in bananas under high temperature. This study enriches the understanding of temperature-related fruit quality deterioration at the post-translational level.
Results
MaNYC1 is involved in high temperature-induced green ripening in banana
Banana fruit ripened at 20 °C started to turn yellow on 3 d and completely degreened after 5 d, but no obvious yellowing was observed even on 6 d when banana fruits were ripened at 30 °C, indicating that green ripening occurs at banana ripened at high temperature (Fig. 1A). The change in peel color showed a rapid increase in color index (CI) in fruits ripened at 20 °C compared with those ripened at 30 °C (Fig. 1B). Consistent with the CI change, the total chlorophyll contents declined at both temperatures, but the rate of degradation was greater at 20 °C than at 30 °C (Fig. 1C). In particular, the original chlorophyll degraded by 89.2% in banana fruits ripened at 20 °C and by 60.9% in fruits ripened at 30 °C on 6 d (Fig. 1C). Thus, high temperature induces the development of green-ripened bananas because of chlorophyll retention.
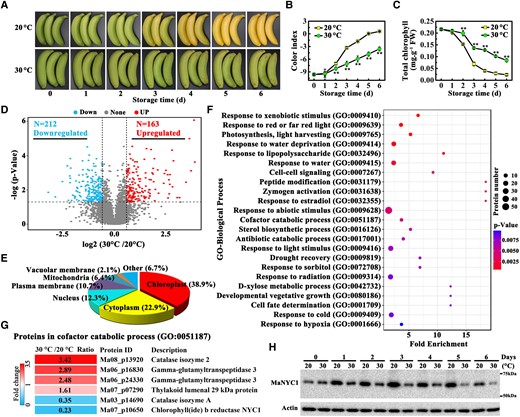
Proteome analysis identifying differentially expressed proteins in banana fruits ripened at 20 and 30 °C. A) Appearance of banana peels during ripening at 20 and 30 °C. Changes in CI B) and total chlorophyll content C) during fruit ripening. The total chlorophyll content was expressed as the mg g−1 fresh weight (FW) of fruit peel. Error bars indicate Se from 6 biological replicates. Asterisks highlight significant differences in banana peels at 20 and 30 °C (Student's t-test, **P < 0.01). D) Volcano plot of the proteins quantified in label-free quantitative proteomic analysis. E) Subcellular location classification of differentially expressed proteins in banana fruits at 20 and 30 °C. F) GO functional classification of proteins differentially expressed in banana fruits ripened at 20 and 30 °C. Biological process terms enriched in the identification group are ranked based on fold change with P < 0.01. Complete proteomics data are shown in Supplemental Table S1. G) Heatmap of differentially expressed proteins enriched in the cofactor catabolic process (G0:0051187) term. H) Western blot validation of differential expression of MaNYC1 protein in banana fruits during ripening at 20 and 30 °C. Equal amount of total protein (30 µg) per lane from each time point sample was subjected to western blotting using anti-MaNYC1 antibody. Actin was used as the loading control.
To define a comprehensive landscape of proteins involved in high temperature-induced green ripening, label-free quantitative mass spectrometry (MS)-based proteomic analysis was performed in banana peels. Because the color differences between fruits ripened at 20 and 30 °C were visible from 3 d, this time point was chosen for proteomic analysis. Using cutoff values based on P value ≤0.05 and fold change ≥1.5 or ≤0.67, 375 differentially expressed proteins in banana fruits ripened at 20 °C versus 30 °C were identified by quantitative proteomic analysis using 3 biological replicates; of these, 212 were downregulated and 163 were upregulated in fruits ripened at 30 °C (Fig. 1D and Supplemental Table S1). Subcellular localization prediction showed that most differentially expressed proteins localized in the chloroplast, accounting for 38.9% of the total differential proteins (Fig. 1E). Gene Ontology (GO) analysis revealed that differentially expressed proteins were involved in a wide range of biological processes, such as the catabolic process, response to abiotic stress, vegetative growth, and cell signaling (Fig. 1F). Interestingly, within the differential proteins in the cofactor catabolic process (GO:0051187), one of the key enzymes involved in chlorophyll degradation was MaNYC1, whose accumulation was repressed by 77% at 30 °C compared with 20 °C (Fig. 1G), thus it was selected for further investigation.
To analyze the potential association between MaNYC1 and green ripening of banana fruit, western blotting was used to assess MaNYC1 protein accumulation in banana peels during ripening at both 20 and 30 °C. The MaNYC1 protein levels in the fruits at 20 °C increased initially during ripening, reached a peak on Day 3, and then gradually decreased (Fig. 1H). Notably, MaNYC1 protein accumulation levels in fruits were markedly lower at 30 °C than at 20 °C throughout the whole ripening stages tested (Fig. 1H). These findings suggest that MaNYC1 is involved in high temperature-induced degreening in banana fruits at the protein level.
MaNYC1 overexpression weakens high temperature-inhibited chlorophyll catabolism
Due to the lack of banana plant transformation, the biological function of MaNYC1 in high temperature-caused green ripening was investigated using transient overexpression assay in banana peels. Successful MaNYC1-HA overexpression was confirmed by western blotting (Fig. 2, A and B). Compared with the empty vector, transient overexpression of MaNYC1 in fruit peels resulted in a distinct degreening phenotype near the injection point in 5 d after ETH treatment at 30 °C (Fig. 2A), as checked by the higher CI value in the MaNYC1-injected area than that in the empty injected area (Fig. 2C). Consistently, the total chlorophyll content was significantly decreased in the MaNYC1-overexpressing peel of fruit under high temperature (Fig. 2C). Collectively, MaNYC1 overexpression attenuates the effect of high temperature on green ripening, presumably by recovering chlorophyll degradation.
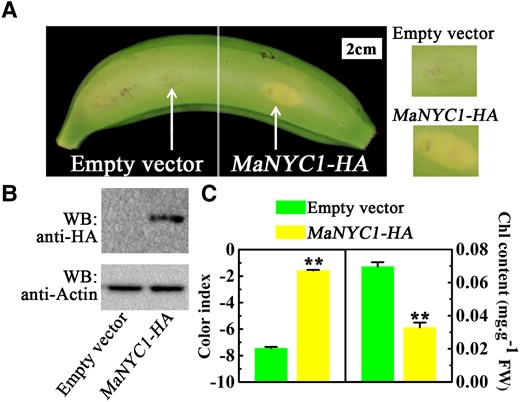
Transient overexpression of MaNYC1 weakens high temperature-inhibiting chlorophyll catabolism. A) Appearance of banana fruits infiltrated with empty vector (left side of fruit) and MaNYC1-HA (right) on Day 5 under 30 °C. Right panels show magnified views around the injection sites. B) Western blot detection of banana peels infiltrated with empty vector and MaNYC1-HA. Fruit peel tissues at the injection region were used for protein detection. Total proteins were extracted and immuno-blotted with anti-HA monoclonal antibody. Actin was used as the loading control. C) Changes in CI and total chlorophyll content of peels as shown in A). The total chlorophyll content was expressed as the mg g−1 FW of fruit peel. Error bars indicate Se from 6 biological replicates (Student's t-test, **P < 0.01).
High temperature induces proteasomal degradation of MaNYC1
UPS-mediated proteolysis is a dominant post-translational modification mechanism in all eukaryotic cells, which has a fundamental function in controlling the abundance of important regulatory proteins (Kerscher et al. 2006). To examine whether high temperature-repressed MaNYC1 protein abundance is ascribed to UPS-mediated degradation, banana fruits which were treated with the proteasomal inhibitor MG132 and then incubated at 20 and 30 °C were used for immunoblotting assays with anti-MaNYC1 antibody. MG132 treatment resulted in maintaining MaNYC1 protein abundance at 30 °C compared with the fruit stored at 30 °C without MG132 treatment, but it had no effect on this protein at 20 °C (Fig. 3A), suggesting that high temperature induces MaNYC1 degradation via the 26S proteasome pathway. Moreover, the co-immunoprecipitation (Co-IP) assay of banana peel tissue was performed with anti-MaNYC1 antibody, and the immunoblot assay with anti-Ub antibody showed that a higher amount of high-molecular mass forms of MaNYC1, corresponding to polyubiquitinated MaNYC1 (Ub(n)-MaNYC1), was detected in banana fruit stored at 30 °C than at 20 °C (Fig. 3B), demonstrating that high temperature facilitates MaNYC1 ubiquitination. These observations suggest that high temperature (e.g. 30 °C) promotes MaNYC1 ubiquitination and degradation via the UPS pathway.
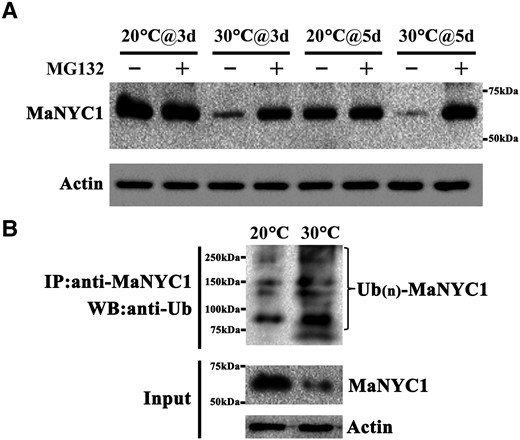
High temperature induces MaNYC1 ubiquitination and proteasomal degradation. A) High temperature induces degradation of MaNYC1 via the 26S proteasome. Banana fruits were treated with the proteasomal inhibitor MG132 and ripened at 20 and 30 °C. An equal amount of total protein per lane from each time point sample was subjected to western blotting using anti-NYC1 antibody, and actin was used as the loading control. B) High temperature promotes MaNYC1 ubiquitination. Endogenous MaNYC1 protein was immunoprecipitated with anti-MaNYC1 antibody from banana fruits ripened at 30 and 20 °C. The ubiquitylated MaNYC1 protein was detected using anti-Ub antibody, and actin was used as the loading control.
RING-type E3 ligase MaNIP1 interacts with MaNYC1 and promotes its ubiquitination and degradation
Immunoprecipitation combined with MS (IP–MS) analysis was performed to investigate the potential protein-interacting partners of MaNYC1. Notably, a RING-type Ub E3 ligase was identified (Ma10_p07000), MaNIP1 (NYC1-interacting protein 1), which showed interaction with MaNYC1 according to the IP-MS data (Supplemental Table S2). The interaction between MaNYC1 and MaNIP1 was then confirmed by yeast two-hybrid (Y2H) assay. Yeast cells cotransforming MaNYC1 and MaNIP1 grew well on the medium without Leu, Trp, His, and Ade, and turned blue in the presence of the chromogenic substrate α-Gal, indicating that MaNYC1 interacts with MaNIP1 in yeast cells (Fig. 4A). Their interaction in living plant cells was subsequently confirmed by split-luciferase and Co-IP assays. In the split-luciferase assay, the recombined luciferase activity was observed in Nicotiana benthamiana (N. benthamiana) leaves coexpressing the MaNYC1-N-terminus of luciferase (nLUC) and the C-terminus of luciferase (cLUC)-MaNIP1, whereas no LUC activity was detected in the negative control combinations (Fig. 4B). In the Co-IP assay, MaNIP1-His was immunoprecipitated with MaNYC1-green fluorescent protein (GFP) but not with GFP alone (Fig. 4C). These data demonstrate the interaction between MaNYC1 and MaNIP1.
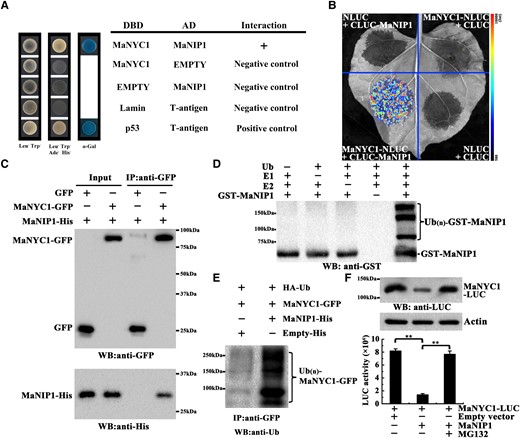
MaNIP1 interacts with MaNYC1 and mediates its ubiquitination and degradation. A) Y2H assay for the interaction between MaNYC1 and MaNIP1. Yeast cells cotransformed with DBD-MaNYC1 and AD-MaNIP1 were grown on selective medium and stained with α-Gal to test protein interaction. B) Protein–protein interactions between MaNYC1 and MaNIP1 in split-luciferase assays in N. benthamiana leaves. MaNYC1-NLUC was coexpressed with CLUC-MaNIP1, whereas MaNYC1-NLUC/CLUC-empty vector, NLUC-empty vector/CLUC-MaNIP1, and NLUC-empty vector/CLUC-empty vector were used as negative controls. Luciferase activity was recorded with a charge-coupled device camera. C) Co-IP assays of MaNIP1–MaNYC1 interaction. MaNYC1-GFP and MaNIP1-His, or Empty-GFP and MaNIP1-His, were transiently expressed in N. benthamiana leaves and immunoprecipitated with anti-GFP antibody. Immunoprecipitated samples and input controls were detected with anti-GFP and anti-His antibody, respectively. D) E3 Ub ligase activity of MaNIP1. Recombinant GST-MaNIP1 fusion protein was incubated in the presence or absence of Ub, E1 Ub-activating enzyme (E1), and E2 Ub-conjugating enzyme (E2). The reactions were analyzed by immunoblotting using anti-GST antibody. E3 Ub ligase activity of GST-MaNIP1 was only detected in the presence of E1, E2, and Ub. E) In vivo MaNYC1 ubiquitination by MaNIP1. Ubiquitylated proteins in total protein extracts from N. benthamiana leaves transiently expressing HA-Ub, MaNYC1-GFP, and MaNIP1-His or Empty-His with different combinations were captured with anti-GFP antibody. The ubiquitylated MaNYC1 protein was detected using anti-Ub antibody. F) Proteasome-mediated degradation assay of MaNYC1. MaNYC1 fused with LUC was coexpressed with MaNIP1 or empty vector in N. benthamiana leaves in the presence or in the absence of proteasome inhibitor (MG132). The MaNYC1 protein level was analyzed by western blotting using anti-LUC antibody (top panel). The MaNYC1 protein level was quantified by measuring luciferase activity (bottom panel). Data are mean ± Se of 6 biological replicates (Student's t-test, **P < 0.01).
Most C3HC4-type RING finger proteins possess E3 Ub ligase activity (Ma et al. 2009). MaNIP1 harbors a C3HC4 RING finger domain and displays considerable similarity (49.3%) to Arabidopsis C3HC4-type RING finger protein AT4G22250 (Supplemental Fig. S1). In vitro ubiquitination assay revealed that MaNIP1 had Ub ligase activity, as it could promote self-ubiquitination (Fig. 4D and Supplemental Fig. S2). Thus, we hypothesized that MaNIP1 might ubiquitinate MaNYC1. To test this hypothesis, in vivo ubiquitination assay was performed by coexpressing MaNYC1-GFP and MaNIP1-His in N. benthamiana leaves. After immunoprecipitation with anti-GFP beads, anti-Ub antibody was used to detect poly-ubiquitinated MaNYC1. The abundance of MaNYC1 ubiquitination was higher when MaNYC1 was coexpressed with MaNIP1-His than with Empty-His control vector, indicating that MaNIP1 mediates MaNYC1 ubiquitination (Fig. 4E). To further investigate whether MaNYC1 undergoes MaNIP1-mediated proteasomal degradation, MaNYC1-LUC and MaNIP1 were transiently expressed in N. benthamiana leaves and then treated with the proteasomal inhibitor MG132. MaNYC1 protein levels substantially declined when it was coexpressed with MaNIP1 compared with the empty control vector, but this decline was substantially reduced by MG132 (Fig. 4F). Quantifying luciferase activity also showed that the presence of MaNIP1 led to MaNYC1 degradation, whereas adding MG132 attenuated this effect (Fig. 4F). Collectively, these results demonstrate that MaNIP1 targets MaNYC1 for ubiquitination, thereby degrading MaNYC1 via the 26S proteasome pathway.
MaNIP1 is involved in high temperature-induced proteasomal degradation of MaNYC1
To determine whether MaNIP1 participates in high temperature-induced proteasomal MaNYC1 degradation, western blot analysis was performed. MaNIP1 protein levels increased obviously in fruit ripening at 30 °C compared with 20 °C (Fig. 5A). Furthermore, the relative protein level of MaNIP1 was inversely correlated with MaNYC1 (R = −0.562, P < 0.05; Fig. 5B), implying that MaNIP1 might negatively regulate MaNYC1 stability. Moreover, a Co-IP assay of banana peel tissue performed with anti-MaNYC1 antibody showed that a higher amount of high-molecular mass form of MaNYC1, which represents polyubiquitination of MaNYC1 (Ub(n)-MaNYC1), was detected in banana fruit ripened at 30 °C than at 20 °C (Fig. 5C). In contrast with the decrease in the MaNYC1 protein level, the MaNIP1 protein level was noticeably increased in the anti-MaNYC1 antibody immunoprecipitated complex in fruits at 30 °C compared with fruits at 20 °C (Fig. 5C), suggesting that MaNIP1 participates in high temperature-induced ubiquitination of MaNYC1 for proteasomal degradation.
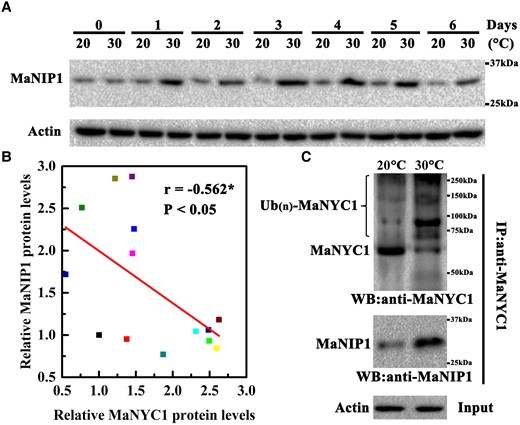
MaNIP1 is involved in high temperature-induced ubiquitination and degradation of MaNYC1. A) Changes in MaNIP1 protein accumulation in banana fruits during ripening at 20 and 30 °C. Equal amount of total protein (30 µg) per lane from each time point sample was subjected to western blotting using anti-MaNIP1 antibody. Actin was used as the loading control. B) Significant correlation between MaNYC1 and MaNIP1 protein levels. The relative protein levels of MaNYC1 and MaNIP1 were quantified based on western blotting by densitometric analysis using Image J software. Asterisk (*) represents significant correlation at 0.05 level determined by SPSS Statistics 20. C) MaNIP1 participates in high temperature-promoted ubiquitination of MaNYC1. MaNYC1 protein in the total proteins extracted from banana fruits ripened at 20 and 30 °C was immunoprecipitated with anti-MaNYC1 antibody. The ubiquitinated MaNYC1 protein was detected using anti-MaNYC1 antibody. The presence of MaNIP1 in the immunoprecipitated complex was analyzed by anti-MaNIP1 antibody. Actin was used as the loading control.
MaNIP1 attenuates MaNYC1-induced chlorophyll degradation in banana fruit
To dissect the role of MaNIP1 in chlorophyll degradation in bananas, it was transiently overexpressed in banana peels. When banana fruit were ripened at 20 °C, transient overexpression of MaNIP1 in banana peels led to a green phenotype near the injection point, compared with the empty vector control (Fig. 6, A and B). Concomitantly, a lower CI and higher chlorophyll contents were found in the MaNIP1-injected areas (Fig. 6C). In the case of banana ripening at 30 °C, transient overexpression of MaNYC1 caused a distinct yellowing phenotype, whereas the phenotype was abolished by coexpression with MaNIP1 (Fig. 6, D and E). Consistent with this observation, MaNYC1 overexpression led to increased CI and decreased total chlorophyll contents, whereas this effect was blocked in the presence of MaNIP1 overexpression (Fig. 6F). These results together support the notion that MaNIP1 attenuates the activation of MaNYC1-mediated chlorophyll degradation and degreening in banana fruit.
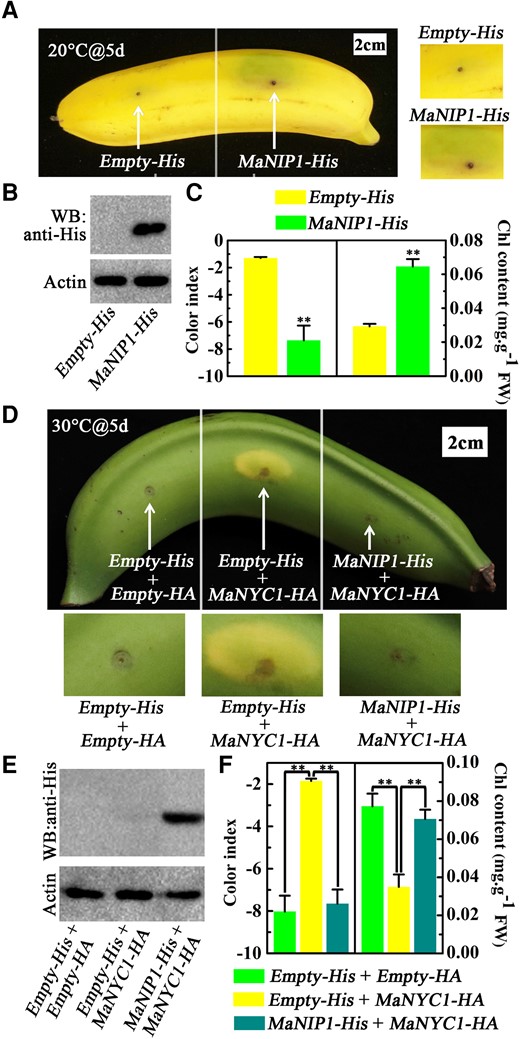
Attenuation of MaNIP1 in MaNYC1-induced chlorophyll degradation. Transient overexpression of MaNIP1 in banana fruits inhibits chlorophyll degradation. A) Appearance of banana fruits infiltrated with empty vector (left) and MaNIP1-His (right) under 20 °C on Day 5. Right panels show magnified views around the injection sites. B) Western blot assays of banana peels infiltrated with empty vector and MaNIP1-His. MaNIP1-His protein was detected by anti-His antibody. Actin was used as the loading control. C) Changes in CI and total chlorophyll content of banana peels as shown in A). MaNIP1 attenuates MaNYC1-induced chlorophyll degradation. D) Appearance of banana fruits coexpressing Empty-His and Empty-HA vectors (left, negative control), MaNYC1-HA and Empty-His (middle), or MaNYC1-HA with MaNIP1-His (right). After injection, fruits were allowed to ripen at 30 °C for 5 d. Bottom panels show magnified views around the injection sites. E) Western blotting showing MaNIP1-His protein level in banana peels infiltrated with different vectors. F) Changes in CI and total chlorophyll content of banana peels as shown in D). In C) and F), the total chlorophyll content was expressed as the mg g−1 FW of fruit peel. Error bars indicate Se from 6 biological replicates (Student's t-test, **P < 0.01).
Discussion
Terrestrial plants commonly experience heat stress due to global warming, which leads to growth limitation, yield loss, or even the whole plant death. As for fruit ripening, high temperature has become one of the most severe abiotic stresses restricting quality formation (Ruiz-nieves et al. 2021). Degreening, caused by rapid chlorophyll degradation, is a normal process associated with fruit ripening and thus determines fruit appearance quality. In most cases, heat stress promotes chlorophyll degradation and degreening (Wang et al. 2018; Janni et al. 2020). However, it can be delayed remarkably or abolished completely in some cases, such as banana fruit (Thomas and Howarth 2000). In banana, the lowest temperature that suppresses degreening is 24 °C, and this temperature is not high enough to inhibit enzymatic reactions. Therefore, banana fruit chlorophyll degradation in response to moderate heat stress might be regulated by a hitherto unknown regulatory mechanism. Herein, we discussed and identified a molecular mechanism regulating chlorophyll degradation in banana fruit under high temperature.
Chlorophyll degradation is a highly regulated metabolic process that requires the coordinated action of multiple enzymes (Kuai et al. 2017). Genes encoding chlorophyll catabolic enzymes are involved in green ripening of bananas at high temperatures. At the transcript level, the expression of chlorophyll catabolic genes was substantially inhibited in fruits held at 30 °C compared with fruits stored at 20 °C (Yang et al. 2009; Du et al. 2014). However, the protein levels of chlorophyll catabolic enzymes in green ripening bananas have not been reported. In this study, 375 differentially expressed proteins were identified at the proteomic level in normal yellow and green ripened banana fruits (Fig. 1, A–D and Supplemental Table S1). Among these, MaNYC1 protein accumulation displayed a reduced level when banana fruit was ripened at 30 °C, which corresponds well with the phenotype of green ripening under high temperature (Fig. 1, F–H). Mutation in NYC1 blocks chlorophyll degradation and thus leads to the stay-green phenotype during natural leaf senescence and seed maturation (Sato et al. 2009; Nakajima et al. 2012). Similarly, transient overexpression of MaNYC1 in banana peels reduced the chlorophyll content and weakened the inhibition of degreening at 30 °C (Fig. 2), demonstrating the function of MaNYC1 in chlorophyll degradation in banana fruit. Based on previous reports and current findings, it seems plausible that high temperature inhibits the function of chlorophyll catabolic enzymes at both transcript and protein levels, which in turn causes green ripening of bananas. In addition to heat stress, chlorophyll loss has also been reported to be associated with other stresses, such as drought stress (Ozfidan et al. 2013; Silva et al. 2020). Our present study found that dehydration response pathway (GO:0009414, GO:0009415, and GO:0009819) was significantly enriched in the quantitative proteomic comparison between normal yellow and green ripening bananas (Fig. 1F). Thus, it will be interesting to pursue whether high temperatures affect chlorophyll degradation by causing water loss.
In the past few years, great progress has been made in exploring the transcriptional regulation of the chlorophyll degradation pathway (Kuai et al. 2017), but little is known about the translational and post-translational regulation. Protein ubiquitination is an important post-translational modification that results in protein degradation (Smalle and Vierstra 2004; Sadanandom et al. 2012). Thus, the fact that ripening of banana at 30 °C induced MaNYC1 ubiquitination and degradation via the 26S proteasome pathway (Fig. 2), suggests that MaNYC1 is regulated by UPS-mediated post-translational modification under high temperature. E3 Ub ligases, as the major components of UPS, are responsible for substrate specificity in ubiquitination (Sullivan et al. 2003). The RING-type Ub E3 ligase SP1 mediates the ubiquitination and degradation of chloroplast outer envelope membrane components to regulate chloroplast protein import (Ling et al. 2019), suggesting that E3 ligases are involved in the regulation of chloroplast-related metabolism. In this study, a heat-induced RING-type Ub E3 ligase MaNIP1 was identified as a MaNYC1-interacting protein, and it promoted ubiquitination-mediated proteasomal degradation of MaNYC1 (Figs 4 and 5), thus negatively regulating chlorophyll degradation in banana peels (Fig. 6). Importantly, this study demonstrates that MaNIP1 participates in 30 °C-induced MaNYC1 ubiquitination (Fig. 5, B and C). Taken together, the data of the present study reveal that 30 °C suppressed MaNYC1-induced chlorophyll degradation by activating MaNIP1-mediated MaNYC1 degradation. Thus, we inferred that E3 Ub ligases may play an important role in chlorophyll degradation and thus regulate fruit color change under temperature stress. Notably, the biological functions of MaNIP1 homologous proteins in other plants, especially in important horticultural crops, are still unknown, which deserves further investigation.
Changes in cellular protein levels are usually determined by protein stability as well as by the efficiency of gene expression (Xu and Xue 2019; Kidokoro et al. 2022). A previous study showed that high temperature represses MaNYC1 expression in banana fruits (Yang et al. 2009). Similarly, the gene expression of MaNYC1 was markedly lower in the fruits ripened at 30 °C than those at 20 °C (Supplemental Fig. S3), which was consistent with the phenotypes of green ripening under high temperatures (Fig. 1, A–C), suggesting that MaNYC1 is also transcriptionally regulated. During tomato and citrus fruit ripening, chlorophyll catabolic genes are transcriptionally regulated by several TFs, such as CitERF13 (Yin et al. 2016) and NOR-like 1 (Gao et al. 2018). However, the upstream transcriptional regulators of MaNYC1 are still unknown and remain to be elucidated further.
Based on the findings, we proposed a model describing the molecular basis of high temperature-induced repression of chlorophyll degradation and green ripening in bananas (Fig. 7). At normal ripening temperature (e.g. 20 °C), MaNYC1 is activated and induces chlorophyll degradation, thereby promoting banana peel degreening during ripening. When banana fruit is ripened at high temperature (e.g. 30 °C), MaNIP1 protein expression is induced. MaNIP1 interacts with and ubiquitinates MaNYC1, which leads to MaNYC1 proteasomal degradation. As a result, chlorophyll degradation processes are inhibited, and banana fruit fails to develop a fully yellow peel. Taken together, the findings establish a dynamic regulatory module of MaNIP1–MaNYC1 that regulates high temperature-induced repression of chlorophyll degradation and green ripening in bananas, which provides insights into the post-translational regulatory mechanism underlying fruit quality deterioration under high temperature stress.
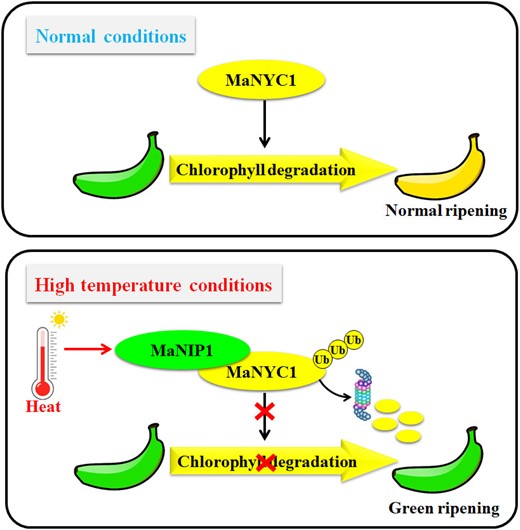
A proposed working model for the function of the MaNIP1–MaNYC1 complex in high temperature-induced green ripening in banana. At normal condition (20 °C), MaNYC1 induces chlorophyll degradation following normal ripening. Under high temperature (30 °C), MaNIP1 levels increase, and MaNIP1 promotes the ubiquitination and proteasomal degradation of MaNYC1 protein, which leads to the repression of chlorophyll degradation in peels and causes green ripening in banana.
Materials and methods
Plant materials and treatments
Pre-climacteric banana (M. acuminata, AAA group, cv. Cavendish) fruits at 75% to 80% maturation were obtained from a local commercial plantation near Guangzhou, China. Ripening of banana fruit was initiated using 100 µL L–1 ETH for 24 h. Fruits were allowed to ripen at 20 or 30 °C in polyethylene bags (0.01 mm thickness) for 6 d, as described by Wu et al. (2019). Fruit before ripening initiation functioned as Day 0 samples common to the 20 and 30 °C treatments. At each sampling time, the CI and chlorophyll contents were recorded according to previously reported methods (McGuire 1992; Yang et al. 2009). Six biological replicates were measured for all samples.
Label-free proteome quantification
Day 3 samples of 20 and 30 °C treatments were chosen for proteomic analysis. Label-free proteome quantification was performed by PTM BIO Co., Ltd (Hangzhou, China). Total proteins were extracted from banana fruit tissues with trichloroacetic acid precipitation as previously reported (Wang et al. 2006). The protein concentration was determined with a bicinchoninic acid assay kit (Merck) according to the manufacturer's instructions. Three biological replicates of the proteomic analysis were performed. For digestion, the protein solution was reduced with 5 mM dithiothreitol for 30 min at 56 °C and alkylated with iodoacetamide (11 mM) in darkness for 15 min. Trypsin was added at 1:50 trypsin-to-protein mass ratio for the first digestion overnight and 1:100 trypsin-to-protein mass ratio for a second 4 h digestion. Tryptic peptides were dissolved in solvent A (0.1% [v/v] formic acid and 2% [v/v] acetonitrile in water) and directly loaded onto a homemade reversed-phase analytical column (30 cm length, 100 μm i.d.). Peptides were separated with a gradient from 6% to 24% solvent B (0.1% formic acid in acetonitrile) in 70 min, 24% to 35% over 14 min, 35% to 80% in 3 min, and held at 80% for the last 3 min, all at a constant flow rate of 450 nL min−1 on a nanoElute UHPLC system (Bruker Daltonics). The peptides were subjected to capillary source followed by timsTOF Pro (Bruker Daltonics) MS. The electrospray voltage applied was 1.75 kV. Precursors and fragments were analyzed using a time-of-flight detector with an MS/MS scan range from 100 to 1,700 m/z. The timsTOF Pro was operated in parallel accumulation–serial fragmentation (PASEF) mode. Precursors with charge states 0 to 5 were selected for fragmentation, and 10 PASEF–MS/MS scans were acquired per cycle. The resulting MS/MS data were analyzed by MaxQuant software (v1.6.6.0) against the banana genome hub (http://banana-genome-hub.southgreen.fr/).
Gene cloning and sequence analysis
RNA was extracted according to the hot borate method with minor modifications (Xiao et al. 2013). cDNA was generated using the reverse transcription kit (Vazyme) in accordance with the manufacturer's protocol. Full-length MaNYC1 or MaNIP1 was cloned by polymerase chain reactions and verified by sequencing. Amino acid sequence alignment of MaNIP1 with other C3HC4-type RING proteins was performed using the ClustalW software.
Western blot analysis
To produce anti-MaNYC1 and anti-MaNIP1 antibodies, His-tagged proteins were expressed in the Escherichia coli strain BM Rosetta (DE3) and purified by Ni-NTA agarose (GE), followed by separation by sodium dodecyl sulfate–polyacrylamide gel electrophoresis (SDS-PAGE). Antibodies were prepared and purified by Hangzhou HuaAn Biotechnology Co., Ltd (Hangzhou, China) as described previously (Shan et al. 2020). Proteins from banana fruits were extracted as described above and separated by SDS-PAGE. After electrophoresis, the protein was electro-transferred onto a nitrocellulose membrane (0.45 µm, Thermo Scientific) using a Bio-Rad transfer apparatus. Western blot analysis was performed using anti-MaNYC1 or anti-MaNIP1 antibody with secondary goat anti-rabbit IgG peroxidase antibody (Thermo Scientific). Specificities of anti-MaNYC1 and anti-MaNIP1 antibodies are described in Supplemental Figs S4 and S5, respectively.
Transient expression analysis in banana peels
The experimental procedure for transient expression analysis in banana peels was performed as described previously (Wei et al. 2020). In brief, the open reading frame of MaNYC1 was inserted into the pCXUN-HA vector, and MaNIP1 was fused with the 6× His tag (GTGATGGTGATGGTGATG) and inserted into the pCXUN vector. The resultant vectors were transferred into Agrobacterium tumefaciens and injected into mature green banana fruit peels. Transformed fruits were treated with 100 µL L–1 ETH on Day 1 after infiltration and held at 20 or 30 °C and 90% relative humidity for 5 d. Peel tissues around the injection sites were used for the measurement of CI and chlorophyll content.
Co-IP and ubiquitination assays
Proteins were extracted from banana fruits with 50 mM Tris–HCl buffer, pH 7.4, as described previously (Shan et al. 2020). The protein extract was mixed with anti-MaNYC1 antibody overnight, protein A agarose beads (Roche) were added, and the suspension was incubated for 4 h to capture protein complexes. After incubation, the beads were collected and protein complexes were separated. To identify MaNYC1-interacting proteins, protein bands were excised from the SDS-PAGE gel and digested with sequencing grade trypsin (Promega). Peptides were reconstituted in formic acid and analyzed by LC–MS/MS. To verify the MaNYC1–MaNIP1 interaction and MaNYC1 ubiquitination in banana fruits, IPed proteins were analyzed by western blotting using anti-MaNIP1, anti-MaNIP1, and anti-Ub (Sigma) antibodies.
Co-IP and ubiquitination assays were also performed in N. benthamiana leaves as described previously (Fan et al. 2017). The full-length MaNYC1 and MaNIP1 coding sequences were cloned into the vectors pEAQ-GFP and pEAQ-His, respectively, and the resulting vectors were coinfiltrated into N. benthamiana leaves via A. tumefaciens. For proteasome inhibition, leaves were infiltrated with 10 μ M MG132 (Merck) solution for 12 h, and proteins were then extracted using the above method. The protein complexes were immunoprecipitated with anti-GFP antibody and were subjected to western blot analysis using anti-His antibody (Abcam) and anti-GFP antibody (Abcam) for the Co-IP assay, and anti-Ub antibody (Sigma) for the ubiquitination assay.
E3 Ub ligase activity assay
The full-length cDNA of MaNIP1 was inserted into the pGEX-4T-1 vector in frame with GST Tag. The GST-MaNIP1 fusion protein was expressed in the E. coli strain BM Rosetta (DE3) and purified using Glutathione-Sepharose 4B (TaKaRa). Five hundred nanograms of GST-MaNIP1 recombinant protein were incubated for 2 h in the presence or in the absence of 50 ng of human E1 (Boston Biochem), 250 ng of human E2 (Boston Biochem), and 2 mg of Ub (Boston Biochem). The reaction products were subjected to western blotting using anti-GST (Abcam) antibody.
Y2H assay
The yeast strain Y2HGold and Clontech Matchmaker Gold Yeast two-hybrid (Y2H) systems were used for Y2H assays as reported previously (Shan et al. 2020). MaNYC1 and MaNIP1 were subcloned into pGBKT7 and pGADT7 vectors, respectively. Y2HGold yeast cells transformed with DBD-MaNYC1 and AD-MaNIP1 were grown on a selective medium, and the possible protein–protein interactions were detected on the basis of their growth status and α-galactosidase activity. Plasmids, pGADT7-T-antigen and pGBKT7-lamin, or pGADT7-T-antigen and pGBKT7-p53, were cotransformed into yeast as negative and positive interaction controls, respectively.
Split-luciferase assay
MaNYC1 was fused to the N-terminal half of luciferase of plasmid pCAMBIA1300-NLUC, whereas MaNIP1 was fused to the C-terminal half of luciferase of plasmid pCAMBIA1300-CLUC. These resultant vectors, as well as empty plasmids, were transformed into A. tumefaciens strain GV3101 by the freeze–thaw method. On Day 3 after infiltration, luciferase luminescence was detected by a chemiluminescence imager with a cooled charge-coupled device camera (Bio-Rad).
In vivo protein degradation assay
MaNYC1 fused with LUC was coexpressed with MaNIP1 in N. benthamiana leaves following agroinfiltration as described above. The transformed leaves were incubated with 10 μ M MG132 or distilled water for 12 h before harvesting, and the leaf total proteins were isolated using a protein extraction kit (Bangfei, Beijing, China) and analyzed by western blotting using anti-LUC antibody. LUC activity was determined with the luciferase reporter gene assay kit (Yeasen, Shanghai, China). Each luciferase assay was performed 6 times.
Statistical analysis
All data were analyzed by SPSS Statistics software 19.0 (IBM Corp., Armonk, NY, USA) and presented as mean ± Se of 3 or 6 biological replicates. Statistical significance of differences between mean values was determined by Student's t-test (P < 0.05 or 0.01).
Primers
The primer details are listed in Supplemental Table S3.
Accession numbers
Sequence data from this article can be found in the banana genome database (https://banana-genome-hub.southgreen.fr/) under accession numbers MaNYC1 (Ma07_p10650) and MaNIP1 (Ma10_p07000).
Acknowledgments
We would like to thank Dr George P. Lomonossoff at the John Innes Centre, Norwich Research Park, for the generous gift of pEAQ vectors.
Author contributions
W.S. conceived and designed the research. Q.L., W.W., and W.S. performed the experiments. Q.L., JF.K., WJ.L., YY.Y, C.J.W, and W.S. analyzed the data. Q.L., J.F.K., and W.S. wrote and revised the manuscript. All authors discussed the manuscript.
Supplemental data
The following materials are available in the online version of this article.
Supplemental Figure S1. Structural features of MaNIP1 protein.
Supplemental Figure S2. The prokaryon-expressed and purified GST-MaNIP1 recombinant protein was visualized on a Coomassie blue-stained SDS-PAGE.
Supplemental Figure S3. The expression of MaNYC1 in banana peel during fruit ripening at 20 and 30 °C.
Supplemental Figure S4. Western blot analysis of the specificity of anti-MaNYC1 antibody.
Supplemental Figure S5. Western blot analysis of the specificity of anti-MaNIP1 antibody.
Supplemental Table S1. Proteins identified by label-free quantitative proteomic analysis.
Supplemental Table S2. Identification of MaNYC1-interacting proteins in IP–MS.
Supplemental Table S3. Summary of primers used in this study.
Funding
This study was supported by the National Key Research and Development Program of China (grant no. 2022YFD2100103), National Natural Science Foundation of China (grant nos. 32072279, 31830071, 31701652, and 31372111), the China Agriculture Research System of MOF and MARA (grant no. CARS-31), and the Natural Science Foundation of Guangdong Province, China (grant no. 2017A030310353).
References
Author notes
The author responsible for distribution of materials integral to the findings presented in this article in accordance with the policy described in the Instructions for Authors (https://dbpia.nl.go.kr/plphys/pages/General-Instructions) is Wei Shan ([email protected]).
Conflict of interest statement. The authors declare no conflict of interest.