-
PDF
- Split View
-
Views
-
Cite
Cite
Weihao Wang, Yuying Wang, Tong Chen, Guozheng Qin, Shiping Tian, Current insights into posttranscriptional regulation of fleshy fruit ripening, Plant Physiology, Volume 192, Issue 3, July 2023, Pages 1785–1798, https://doi.org/10.1093/plphys/kiac483
- Share Icon Share
Abstract
Fruit ripening is a complicated process that is accompanied by the formation of fruit quality. It is not only regulated at the transcriptional level via transcription factors or DNA methylation but also fine-tuned after transcription occurs. Here, we review recent advances in our understanding of key regulatory mechanisms of fleshy fruit ripening after transcription. We mainly highlight the typical mechanisms by which fruit ripening is controlled, namely, alternative splicing, mRNA N6-methyladenosine RNA modification methylation, and noncoding RNAs at the posttranscriptional level; regulation of translation efficiency and upstream open reading frame-mediated translational repression at the translational level; and histone modifications, protein phosphorylation, and protein ubiquitination at the posttranslational level. Taken together, these posttranscriptional regulatory mechanisms, along with transcriptional regulation, constitute the molecular framework of fruit ripening. We also critically discuss the potential usage of some mechanisms to improve fruit traits.
Introduction
Fleshy fruits are an essential part of the human diet, providing sugars, organic acids, dietary fibers, and other health-supporting nutrients. The fruit-ripening process entails a series of remarkable alterations that consist of softening, accumulation of pigments, emission of aroma volatiles, and biosynthesis of characteristic nutrients (Giovannoni et al., 2017). These distinct biological sub-processes are highly coordinated by regulating the expression of genes. According to differential respiratory patterns during fruit ripening, fleshy fruits can be classified into two categories: climacteric fruit and nonclimacteric fruit. Climacteric fruit feature a typical respiratory peak with an ethylene burst, whereas nonclimacteric fruit lack a substantial burst in both respiration rate and ethylene production (Cherian et al., 2014). Being typical models of climacteric fruit and nonclimacteric fruit, respectively, tomato (Solanum lycopersicum) and strawberry (the octaploid Fragaria × ananassa or the diploid Fragaria vesca) have facilitated the study of fruit ripening in recent years. The rapidly expanding availability of sequenced fruit reference genomes, such as those for apple (Malus domestica), banana (Musa acuminata), orange (Citrus cinensis), and so on, have further promoted our understanding of fruit ripening and its underlying regulatory mechanisms. Great advances have been made regarding phytohormones (Fenn and Giovannoni, 2021), transcription factors (Chen et al., 2020), DNA methylation (Tang et al., 2020), and histone modifications (Lü et al., 2018), which collectively constitute the integrated regulatory network of fruit ripening. Those regulatory factors mainly affect gene expression at the transcriptional level. However, posttranscriptional controls are also critical fundamental players in gene expression and can complement transcriptional regulation. The specialized interpretation of posttranscriptional control is known to target the regulation of RNAs. However, in more generalized cases, posttranscriptional control includes translational and posttranslational controls as well (Zhou et al., 2015; Wang et al., 2017; Cai et al., 2018; Tognacca and Botto, 2021). Here, as Figure 1 shows, we aim to provide an overview of posttranscriptional, translational, and posttranslational mechanisms that are fundamental regulatory layers of the fruit-ripening process according to recent advances, and go on to discuss some notable issues for future studies to consider.
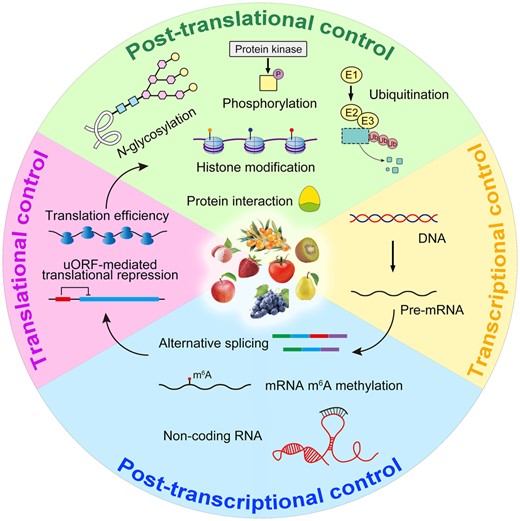
Summary of gene expression control exercised at multi-faceted levels during the fruit ripening process. Pre-mRNA: precursor messenger RNA.
Posttranscriptional regulation of fruit ripening
Regulation of fruit ripening by alternative splicing
Alternative splicing (AS) of pre-mRNAs is prevalent in plants. AS is a key regulatory mechanism for modulating gene expression and it contributes to greater transcriptome diversity (Deng and Cao, 2017). AS events can be categorized into several types: alternative 5′-splicing site, alternative 3′-splicing site, exon skipping, intron retention, mutually exclusive exon, and other more complex patterns (Ule and Blencowe, 2019). High-throughput sequencing technologies, especially of the full-length transcriptome, have enabled the identification of AS events in dozens of fruits, including tomato (Sun and Xiao, 2015), strawberry (Li et al., 2017), citrus (Terol et al., 2016), grape (Vitis vinifera) (Jiang et al., 2017), watermelon (Citrullus lanatus) (Saminathan et al., 2015), sweet cherry (Prunus avium) (Xanthopoulou et al., 2022), and olive (Olea europaea) (Rao et al., 2019). Intron retention as the predominant pattern of AS is conserved across fruit species.
Besides overall changes in AS events, AS of some individual genes could provide a more profound understanding of its pivotal role in fruit ripening. The banana MYB16L transcription factor, which is involved in starch degradation during fruit ripening, undergoes AS and generates a nonfunctional truncated isoform named MYB16S. Although MYB16S itself lacks transcriptional activity, it can inactivate the functional MYB16L by forming MYB16S–MYB16L heterodimers via protein–protein interactions (Jiang et al., 2021). Different varieties of strawberries harbor different splicing mechanisms for the polygalacturonase (PG) gene, generating an active form PG1 or an inactive form T-PG. In soft-texture cultivars, PG1 is the predominant isoform, but in firm-texture cultivars the expression of T-PG exceeds that of PG1 (Villarreal et al., 2008). The yellow-fruited tomato yft2 undergoes a different splicing mechanism for Phytoene synthase 1 (SlPSY1) pre-mRNA than red-fruited tomatoes, thereby generating a nonfunctional SlPSY1 protein that impairs carotenoid synthesis (Chen et al., 2019). Similarly, the Anthocyanin 2 like gene, which encodes an R2R3 MYB transcription factor, experiences distinct splicing patterns between cultivated and wild tomato species; hence, this is the reason why some cultivated tomatoes harbor low levels of anthocyanins (Colanero et al., 2019). Two isoforms of Isopentenyl diphosphate isomerase 1 (SlIDI1) with distinct subcellular localization were identified in tomato, suggesting that the regulatory mechanism of SlIDI1 relies on AS during fruit ripening (Zhou et al., 2022a). A UV-damaged DNA-binding protein 1 (DDB1) isoform derived by AS that is 15-bp longer than the normal isoform has been identified in tomato and designated DDB1+15. Transgenic tomato overexpression of DDB1 rather than DDB1+15 led to a decreased size in almost all organs including fruit, which suggests a role for DDB1 in controlling organ size via AS (Liu et al., 2012). The above studies described several ways by which AS can govern fruit traits. As shown in Figure 2A, the truncated or extended protein formed by AS is functionally different from the normal protein, enabling it to participate in the control of the ripening process.
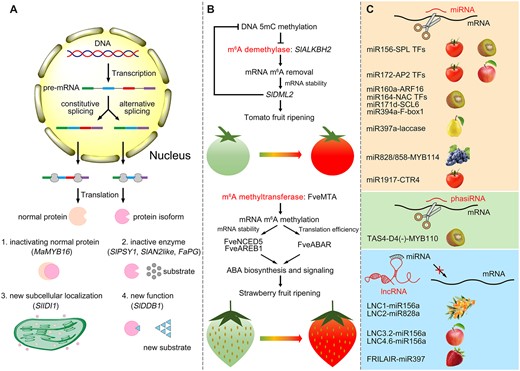
Posttranscriptional mechanisms that can regulate the fruit ripening process. A, One precursor mRNA can produce two or more transcripts via AS. The ensuing protein isoforms translated from these alternative transcripts are functionally different. B, Regulatory roles of mRNA m6A modification in controlling tomato and strawberry fruit ripening. In tomato, the m6A demethylase SlALKBH2 undoes the m6A modification of the SlDML2 transcript, thus stabilizing SlDML2. SlDML2 further erases DNA 5mC methylation, and promotes the transcription of SlALKBH2. In strawberry, the m6A methyltransferase FveMTA deposits m6A modifications to enhance mRNA stability or TE, especially for those mRNAs involved in ABA biosynthesis and signaling. C, Noncoding RNAs regulate fruit ripening. MiRNA and phasiRNA can directly cleave their target mRNAs. Long noncoding RNAs often act as the target mimics of miRNAs to protect mRNAs. Ma, Musa acuminate; Sl, Solanum lycopersicum; Fa, Fragaria × ananassa; Fve, F. vesca; pre-mRNA, precursor messenger RNA.
Regulation of fruit ripening by mRNA m6A methylation
N6-methyladenosine RNA modification (m6A) was first identified in the 1970s and is recognized as the earliest reported type of mRNA modification (Desrosiers et al., 1974). Since then, m6A has been proven to affect aspects of mRNA metabolism, such as mRNA stability, translation efficiency (TE), AS, and nuclear retention/export (Liang et al., 2020a). In plants, m6A plays a crucial role in their various developmental processes and stress responses (Zhou et al., 2022b).
Recently, studies have revealed that mRNA m6A methylation modulates fruit ripening in tomato and strawberry through distinct mechanisms (Figure 2B). During tomato fruit ripening, the m6A demethylase SlALKBH2 (AlkB homolog 2) is involved in the m6A demethylation of DEMETER-like DNA demethylase 2 (SlDML2) mRNA, enhancing its stability. SlDML2 encodes a DNA demethylase that affects the transcriptional expression level of SlALKBH2, generating a feedback loop between DNA methylation and mRNA m6A methylation to regulate the fruit ripening of tomato (Zhou et al., 2019). In strawberry, m6A methyltransferases Methyltransferase A (FveMTA) and FveMTB but not demethylases are necessary for the normal ripening of strawberry fruit. FveMTA promotes the m6A modification of key genes in the abscisic acid (ABA) pathway (9-cis-epoxycarotenoid dioxygenase 5, FveNCED5; ABA-responsive element-binding protein 1, FveAREB1; ABA receptor, FveABAR), which increases their mRNA stability or TE. For strawberry, m6A methylation regulates its fruit ripening in an ABA-dependent manner (Zhou et al., 2021). Besides, the m6A distribution during strawberry fruit ripening is not consistent with that during tomato fruit ripening. More m6A modifications are enriched in the coding sequence in strawberry and these may contribute to its specific regulatory mechanism. The distinct regulatory mechanisms of m6A methylation from these two model fruits suggest that its action might be not conserved across species in controlling their fruit ripening process.
Regulation of fruit ripening by noncoding RNA
In the eukaryotic transcriptome, a large portion of the transcripts consist of noncoding RNAs (ncRNAs) devoid of any potential protein-coding capacity though they may nonetheless have regulatory functions. The ncRNAs can be categorized into microRNA (miRNA), small interfering RNA (siRNA), phased siRNA (phasiRNA), circular RNA, and long noncoding RNA (lncRNA) (Yu et al., 2019). In the past decade, due to the popularization of high-throughput sequencing technologies, there have been substantial advances in the genome-wide identification of ncRNAs in various fruit crops, such as apple (Xia et al., 2012), citrus (Ke et al., 2019), strawberry (Tang et al., 2021), kiwifruit (Actinidia deliciosa) (Wang et al., 2020a), and tomato (Zhu et al., 2015; Zuo et al., 2020). Recently, a series of ncRNAs, especially small RNAs and lncRNAs, that act at the posttranscriptional level have been functionally characterized in fruit ripening and fruit quality formation (Figure 2C).
Overexpression of sly-miR156a in tomato can cause severe phenotypic alterations throughout the whole plant, including its fruit size and yield. Six SBP-box transcription factors are targets of sly-miR156a and this might be responsible for the phenotypes of the transgenic plant (Zhang et al., 2011). In kiwifruit, miR164 contributes to fruit ripening by targeting two NAC-type transcription factors that directly regulate the expression of ripening-related genes (Wang et al., 2020a). miR172 targets AP2 transcription factors to influence multiple traits including fruit size, in both apple and tomato (Yao et al., 2015; Chung et al., 2020). mi397a regulates stone cell lignification by posttranscriptionally inhibiting the expression of laccase genes in pear (Pyrus bretschneideri) fruit (Xue et al., 2019). miR828 and miR858 function as positive regulators of anthocyanin and flavanol accumulation by halting the expression of VvMYB114 in grapes (Tirumalai et al., 2019). miR1917 participates in tomato fruit development and ripening by repressing Constitutive triple response 1-like protein kinase 4, which encodes a component of ethylene signaling (Yang et al., 2020). Recently, a study of red-fruited kiwifruit revealed that MYB10-mediated anthocyanin accumulation occurs only in the inner pericarp, while MYB110 is responsible for anthocyanin accumulation throughout the entire fruit. MYB110 is directly cleaved by a phasiRNA AcTAS4-D4(-); however, MYB10 escapes this cleavage and is indirectly upregulated by miR156b, miR160a, miR171d, and miR394a, which can cleave the suppressors of MYB10 (Wang et al., 2022a). Due to the conservation of sequences and targets of miRNAs, roles of miRNAs in controlling fruit ripening have been constantly identified in recent years. Usually, miRNAs inhibit the expression of the target gene in two major ways: transcript cleavage and translation repression (Yu et al., 2019). Transcript cleavage is the only confirmed way by which miRNA controls fruit ripening; instances of translation repression by miRNA have yet to be demonstrated in the fruit ripening process.
Compared with mRNAs or small RNAs, lncRNAs show little sequence conservation among different species. lncRNAs have more complex regulatory mechanisms than either miRNAs or phasiRNAs. Plant lncRNAs can serve as scaffolds by physically interacting with other molecules, as well as endogenous target mimics of miRNAs, precursors of siRNAs, and templates to be translated into small peptides (Yu et al., 2019). So far, serving as endogenous target mimics of miRNAs is the only reported posttranscriptional manner by which lncRNAs can regulate fruit ripening and fruit quality. In sea buckthorn (Hippophae rhamnoides), two lncRNAs, LNC1 and LNC2, are involved in anthocyanin accumulation and act as decoys that mimic the targets for miR156a and miR828a to regulate the expression of Squamosa-promoter-binding protein-like 9 (SPL9) and MYB114, respectively (Zhang et al., 2018a). Similarly, in apple, MLNC3.2 and MLNC4.6 also act as target mimics of miR156a to, respectively, modulate the expression of SPL2-like and SPL33 during light-induced anthocyanin biosynthesis (Yang et al., 2019). Furthermore, in strawberry, the lncRNA FRILAIR promotes fruit ripening by serving as the target mimic of miR397 to prevent the cleavage of Laccase 11a (Tang et al., 2021). Although lncRNAs can also operate at the transcriptional level to activate target gene expression in apple (Ma et al., 2021; Yu et al., 2022), in most cases, lncRNAs tend to function as endogenous target mimics of miRNAs, to indirectly protect mRNAs from cleavage by miRNAs at the posttranscriptional level during fruit ripening.
Translational regulation of fruit ripening
Regulation of fruit ripening by altering the TE
Translation occurs when the mRNA sequences are decoded into amino acids for protein synthesis. The synthesis rate of a protein is proportional to both the abundance and TE of the corresponding mRNA (Figure 3A). Translational control supervises the TE of mRNAs, and plays an essential role in regulating gene expression (Hershey et al., 2012). The ability to now perform ribosome profiling is a milestone for studying translational control because this facilitates the genome-wide survey of translation events (Ingolia et al., 2009). Since the introduction of ribosome profiling, burgeoning research into translational regulation in animals, plants, and microbes has ensued (Ingolia et al., 2019). Many translational regulatory mechanisms in plants were identified in just this past decade (Pajerowska-Mukhtar et al., 2012; Merchante et al., 2015; Xu et al., 2017a, 2017b; Zhang et al., 2018b; Yoo et al., 2020). Nevertheless, how TE is regulated during fruit ripening is still poorly understood.
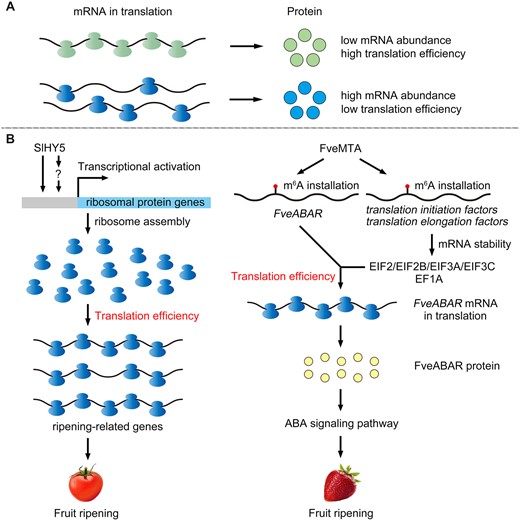
TE is a translational mechanism capable of regulating fruit ripening. A, The protein level is modulated by both the mRNA’s abundance and its TE. B, Tomato transcription factor SlHY5 can directly or indirectly regulate the expression of ribosomal protein genes, to further influence the ribosome pool, which guarantees the TE of various ripening-related genes during the fruit ripening process. In strawberry, FveMTA can install m6A modification onto the FveABAR transcript to promote its TE. In addition, FveMTA also installs m6A modification onto those mRNAs encoding translation initiation factors and translation elongation factors which could improve their mRNA stability and further promote the TE of FveABAR. Sl, Solanum lycopersicum; Fve, F. vesca; mRNA, messenger RNA.
As shown in Figure 3B, Elongated hypocotyl 5 (SlHY5), a basic leucine zipper (bZIP) transcription factor, regulates fruit ripening at the transcriptional level, as expected. Yet SlHY5 also targets ribosomal proteins to impact the TE of ripening-related genes at the translational level during the ripening of tomato fruit (Wang et al., 2021). As mentioned above, the m6A methyltransferase FveMTA promotes m6A methylation of mRNAs, and thus positively regulates fruit ripening in strawberry. The MTA-mediated m6A installation mediates the TE of ripening-related transcripts; specifically, the changes in TE are directly modulated via m6A installation in the target transcripts, but indirectly modulated by m6A-mediated regulation of translation initiation factors and elongation factors (Zhou et al., 2021). The translation is a complex process in which many proteins and RNAs are involved. Therefore, TE may be jointly influenced by many factors. To date, ribosome profiling has yet to be successfully applied using fruit samples. Accordingly, a genome-wide understanding of the translational control during fruit ripening still eludes us.
Regulation of fruit ripening by uORF-mediated translation repression
The upstream open reading frame (uORF) is a functionally specific regulatory element that figures prominently in translational regulation. Prevalent in eukaryotes, the uORF is derived from the 5′-leading sequence of mRNA and is located upstream of the protein-coding main ORF (mORF). Functionally, the uORF modulates the translation initiation of the mORF by sequestering ribosomes, with translation initiation being the rate-limiting step of translation (Zhang et al., 2019). Simply put, the translation of the uORF inhibits the translation of its corresponding mORF (Figure 4A).
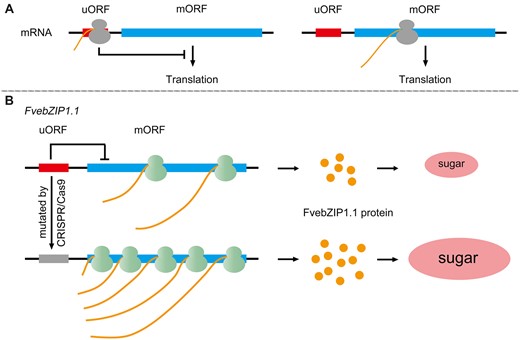
The uORF is a kind of translational regulatory element having high specificity. A, Translation of the uORF inhibits the translation of its corresponding protein-coding mORF. B, In strawberry, uORF mutations generated by CRISPR/Cas9 genome-editing system in the FvebZIP1.1 locus can produce a higher level of FvebZIP1.1 protein, resulting in a greater sugar accumulation in strawberry fruit.
In tomato, SlbZIP1 regulates sugar accumulation via uORF-mediated sucrose-induced repression of translation in the fruit. Overexpression of SlbZIP1 without uORFs can significantly enhance the sugar content of transgenic tomato fruit, suggesting a potential strategy to enhance fruit quality by modifying the uORF (Sagor et al., 2016). Later, Xing et al. disrupted the uORF of FvebZIP1.1, the putatively orthologous gene of SlbZIP1 in strawberry, using the clustered regularly interspaced short palindromic repeats (CRISPR) system (Xing et al., 2020). Mutations in the uORF destroyed the uORF-mediated translational inhibiting effect, with transgenic strawberry plants displaying normal growth and development, but an apparent increase in their sugar content (Figure 4B). The uORF-mediated translational regulation is not only a mechanism capable of high specificity to control gene expression but also a promising potential target for genetic engineering to improve fruit traits.
Posttranslational regulation of fruit ripening
Regulation of fruit ripening by histone modifications
Several recent studies have shown that histone acetylation and methylation play crucial roles during the fruit-ripening process (Figure 5A). Silencing the HD2-type histone deacetylase gene SlHDT3 inhibits the ripening of tomato fruit (Guo et al., 2017a). Conversely, knockdown of the other two deacetylase genes belonging to RPD3/HDA1 subfamily, SlHDA1 or SlHDA3, promotes tomato fruit ripening (Guo et al., 2017b, 2018). The ripening repressor Ethylene responsive factor F12 (SlERF.F12) along with the co-repressor TOPLESS protein 2 (TPL2) together recruit both SlHDA1 and SlHDA3 to assemble a protein complex which can reduce the level of histone acetylation at the promoter regions of ripening-related genes, and thus repress their transcription (Deng et al., 2022). The conserved role of the ERF–TPL–HDA complex in preventing fruit from ripening also exists in apple. MdERF4 interacts with MdTPL4, and recruits MdHDA19 to form a tripartite complex that directly represses the expression of 1-aminocyclopropane-1-carboxylate synthase 3a, by decreasing the degree of histone acetylation, to further reduce ethylene production (Hu et al., 2020, 2022). The effects of histone acetylation marks on fruit ripening seem inconsistent; however, histone acetylation perhaps serves as a fine-tuning factor in the delicate process of fruit ripening.
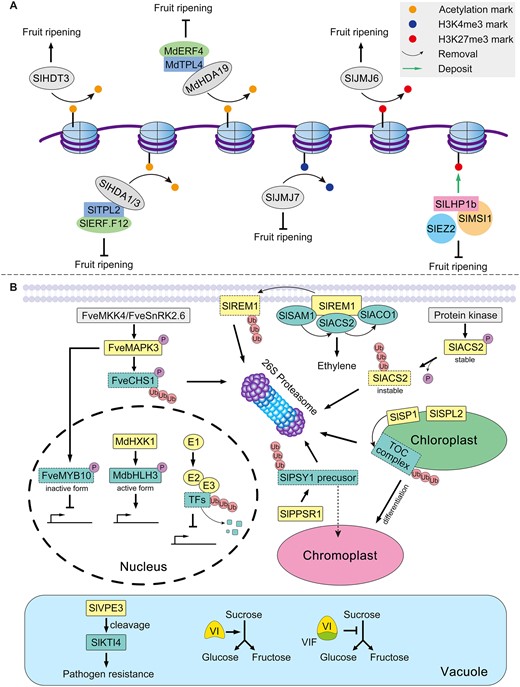
Posttranslational mechanisms that can regulate the fruit ripening process. A, Different histone modifications differ functionally in how they influence fruit ripening. Histone acetylation can either accelerate or decelerate the ripening of fruit. Histone H3K4me3 promotes fruit ripening, whereas histone H3K27me3 inhibits fruit ripening. B, Important posttranslational events involved in fruit ripening. The membrane protein SlREM1 directly binds to SlSAM1, SlACS2, and SlACO1 to increase ethylene production. Meanwhile, SlREM1 undergoes ubiquitin-mediated degradation. In cytosol, SlACS2 is regulated by phosphorylation and ubiquitination. SlACS2 is stabilized via phosphorylation by a protein kinase, while dephosphorylated SlACS2 is unstable and then degraded by the ubiquitin–26S proteasome pathway. E3 ubiquitin ligase SlPPSR1 mediates the degradation of SlPSY1 precursor protein via ubiquitination, to maintain a steady-state level of mature SlPSY1 protein in the chromoplast of tomato. FveMAPK3 negatively regulates anthocyanin accumulation of strawberry and acts downstream of FveMKK4 and FveSnRK2.6. FveMAPK3 can phosphorylate both FveCHS1 and FveMYB10 to inactivate their involvement in anthocyanin accumulation of strawberry. In the nucleus, MdHXK1 can phosphorylate MdbHLH3 to activate its transcriptional activity and promote anthocyanin biosynthesis in apple. Ubiquitination-driven degradation of transcription factors is a common way by which the ripening and quality of fruit is regulated. The E3 ubiquitin ligases SlSP1 and SlSPL2 locate to chloroplast outer envelope membranes where they participate in tomato fruit ripening. Furthermore, SlSP1 is responsible for the ubiquitin-mediated degradation of the TOC complex to promote the chloroplast-to-chromoplast transition. In the vacuole, SlVPE3 cleaves SlKTI4 to regulate the resistance of fruit to pathogens. A vacuolar invertase inhibitor can abolish vacuolar invertase activity due to protein–protein interactions, thus affecting sucrose metabolism during fruit ripening. Sl: Solanum lycopersicum; Fve: F. vesca; Md: M. domestica.
Histone lysine methylation mainly occurs at K4, K9, K27, and K36 of histone H3. The methylation of H3K4 or H3K36 is associated with gene activation, whereas that of H3K9 or H3K27 leads to gene suppression (Kouzarides, 2007). A tomato histone demethylase, SlJMJ7, negatively regulates fruit ripening by directly erasing H3K4me3 from numerous ripening-related genes, precluding their expression (Ding et al., 2022). Overexpression of another tomato histone demethylase, SlJMJ6, which specifically targets H3K27 methylation, accelerates fruit ripening; the transcription of numerous ripening-related genes is upregulated once H3K27me3 is removed (Li et al., 2020). The polycomb repressive complex 1 (PRC1) and PRC2, consisting of polycomb group proteins, also participate in regulating the fruit-ripening process via histone modification. PRC2 trimethylates the histone H3 at Lys27, which is functionally conserved across plant species (Mozgova and Hennig, 2015). The functioning of Multicopy suppressor of IRA1 (SlMSI1) and Enhancer of zeste 2 (SlEZ2), subunits of PRC2, in fruit ripening has been investigated in tomato. Overexpression of SlMSI1 blocks the ripening process, whereas silencing hastens fruit ripening (Liu et al., 2016). Further, knockdown of SlEZ2 not only alters fruit ripening but also causes severe developmental disorders to the whole plant (Boureau et al., 2016). Like heterochromatin protein 1b (SlLHP1b), a tomato PRC1-like protein, was shown to repress fruit ripening as well (Liang et al., 2020b). SlLHP1b can bind to the epigenetic mark H3K27me3 in vivo, to stabilize the repressive histone mark. Accordingly, H3K27me3 levels of ripening-related genes (ACS2; ACS4; PG2a; Ripening inhibitor [RIN]) decrease in the RNAi fruit but increase in overexpressing fruit. Moreover, because SlLHP1b can interact with SlMSI1, PRC1, and PRC2 might work synergistically to regulate fruit ripening. Compared with other histone modifications, the repressive epigenetic mark of H3K27me3 exercises a conserved role in regulating the crucial ripening-related genes in both fleshy and dry fruits (Lü et al., 2018). Currently, the trimethylation of H3K27 has mainly been studied in tomato. Nonetheless, the functions of genes involved in the metabolism of H3K27me3, like SlJMJ6, SlLHP1b, SlMSI1, and SlEZ2, may be conserved in the ripening process of other fruits.
Regulation of fruit ripening by phosphorylation
Protein phosphorylation is the most widespread posttranslational modification (PTM) that occurs in plants. According to the recently compiled statistics, nearly three-quarters of the identified PTM sites are phosphorylation sites (Willems et al., 2019). Phosphorylation often results in the protein’s structural change, thus affecting its activity, protein–protein interactions, and subcellular localization (Schulze, 2010). The 1-aminocyclopropane-1-carboxylic acid synthase (ACS) is recognized as the rate-limiting enzyme in the biosynthesis of ethylene. The tomato ACS2 gene is the most abundant ACS gene during tomato fruit ripening (Liu et al., 2015) and is known to contribute to this process (Oeller et al., 1991). SlACS2 experiences phosphorylation and dephosphorylation at the posttranslational level (Figure 5B). Whereas phosphorylation can stabilize SlACS2, dephosphorylation renders it susceptible to degradation (Kamiyoshihara et al., 2010). The phosphorylation of ACS1 also occurs in banana fruit ripening (Choudhury et al., 2012), implicating a conserved phosphorylation mechanism of ACS in the ripening process of climacteric fruits.
Phosphorylation also occurs in the nuclei. As Figure 5B shows, apple hexokinase MdHXK1 mediates the phosphorylation and stabilization of the transcription factor MdbHLH3, resulting in anthocyanin accumulation (Hu et al., 2016). Mitogen-activated protein kinase 4 (MdMAPK4) enables the phosphorylation of MdMYB1 and MdERF17, thus enhancing their transcriptional activities to promote apple fruit peel coloration in response to light (Yang et al., 2021; Wang et al., 2022b). It was recently reported that strawberry coloration under low temperature is regulated by protein phosphorylation (Figure 5B), in which FveMAPK3 was identified as the center of low temperature-inhibited anthocyanin accumulation. FveMAPK3 locates downstream of MAPK kinase 4 (FveMKK4) and sucrose nonfermenting 1-related kinase 2.6 (FveSnRK2.6), where it becomes activated by FveMKK4- and FveSnRK2.6-mediated phosphorylation. This activated FveMAPK3 can subsequently phosphorylate the transcription factor FveMYB10, a positive regulator in the control of anthocyanin biosynthesis, which reduces its transcriptional activity. Meanwhile, FveMAPK3 phosphorylates the crucial enzyme, chalcone synthase 1 (FveCHS1) in the anthocyanin biosynthesis pathway to promote its proteasome-mediated degradation. Through these two mechanisms, FveMAPK3 suppresses the coloration of strawberry fruit exposed to low temperature (Mao et al., 2022). The effects of protein phosphorylation on protein stability and function are inconsistent. As the most abundant PTM in plants, phosphorylation may be finely regulated according to the process of fruit ripening and local environmental changes. In this way, it can help fruit crops cope with a suite of complicated situations.
Regulation of fruit ripening by ubiquitination
Ubiquitination is a common PTM in eukaryotic cells that results in the selective degradation of target proteins. Ubiquitination controls many essential aspects of plant development, both vegetative and reproductive, including the fruit-ripening process (Miricescu et al., 2018). Ubiquitin is covalently attached to substrate protein via the sequential activity of ubiquitin-activating enzyme (E1), ubiquitin-conjugating enzyme (E2), and ubiquitin ligase (E3). Ubiquitinome analysis can identify ubiquitinated proteins with their corresponding ubiquitination sites in a global view of cellular proteins (He et al., 2020). RIN is a well-known transcription factor that governs the fruit ripening of tomato (Vrebalov et al., 2002). Wang et al. mined the ubiquitinome to uncover altered ubiquitinated proteins in nuclei that were regulated by RIN in ripening tomato fruit (Wang et al., 2014). This study discovered that critical components of the ubiquitin–proteasome system were involved in regulating the tomato fruit-ripening process. The utility of the ubiquitinome for evaluating the potential involvement of protein ubiquitination in the ripening process should be investigated in other fruits.
Plant genomes usually harbor several E1s, dozens of E2s, and hundreds of E3s (Zhou et al., 2018). A banana E1, Ubiquitin-activating enzyme (MaUBA), can interact with the transcription factor MaMADS1 in the nuclei: MaUBA and MaMADS1 are co-stimulated by ethylene and co-suppressed by 1-methylcyclopropene, suggesting a role of MaUBA in banana fruit ripening (Liu et al., 2013). Using transient silencing or an overexpression assay, the tomato E2s (SlUBC32 and SlUBC41) and strawberry E2s (FaUBC76 and FaUBC78) were discovered to regulate fruit ripening (Wang et al., 2014; Li et al., 2022). Compared with E1s and E2s, the E3s evidently comprise a larger protein family in plants in which they interact specifically with target proteins and are responsible for their substrates’ ubiquitination. Much investigation into the ubiquitination of the functional substrate proteins and the corresponding E3s have revealed their involvement in many aspects of fruit ripening, such as pigmentation (Li et al., 2016; Tang et al., 2016; An et al., 2019; Xiong et al., 2019; Ren et al., 2021), nutrient accumulation (Zhang et al., 2020a, 2020b), and the biosynthesis, signaling, and response of phytohormones (Yang et al., 2010; Hu et al., 2019; ShAn et al., 2020; An et al., 2021; Wei et al., 2021; Yu et al., 2021; Song et al., 2022).
The ubiquitination of transcription factors is a common mechanism by which certain aspects of plant physiology and pathology are governed, and this applies to fruit ripening as well (Adams and Spoel, 2018; Blázquez et al., 2020). For example, BT2 is an important component of cullin-RING E3 ligase complexes, and apple BT2 (MdBT2) has been systematically studied in recent years. MdBT2 responds to nitrogen deficiency, wounding, drought, light, and ABA to regulate the respective stability of several transcription factors (e.g. MYB1, bZIP44, WRKY40, ERF38, and TCP46), and thereby determine anthocyanin accumulation in apple fruit (An et al., 2020). Interestingly, as Figure 5B shows, degradation of a membrane protein remorin 1 (SlREM1) mediated by the ubiquitin–proteasome system can also affect tomato fruit ripening (Cai et al., 2018). SlREM1 can physically interact with S-adenosylmethionine synthetase 1 (SlSAM1), SlACS2, and 1-aminocyclopropane-1-carboxylic acid oxidase 1 (SlACO1), all of which are key enzymes in ethylene biosynthesis, to promote ethylene production during fruit ripening. Furthermore, SlREM1 is also degraded by ubiquitin-mediated proteolysis to prevent the continuous induction of ethylene. Plastids are abundant in tomato fruit, undergoing a specialized form of chloroplast-to-chromoplast differentiation during ripening to drive the accumulation of carotenoids. However, plastid protein degradation mediated by the ubiquitin–proteasome system is rarely reported in plants. Chloroplast outer membrane-protein degradation mediated by the ubiquitin–proteasome system was first described for Arabidopsis (Arabidopsis thaliana) (Ling et al., 2012, 2019). Later, a similar mechanism, named the chloroplast-associated protein degradation proteolytic pathway, which is triggered by the plastid ubiquitin E3 ligase SP1 and its homolog SPL2, was identified in tomato (Ling et al., 2021) (Figure 5B). Although both SP1 and SPL2 are necessary for the normal ripening of tomato fruit, SP1 is further responsible for chromoplast differentiation during this process. SlPSY1, the rate-limiting enzyme in carotenoid biosynthesis pathway, locates to the plastids where it is especially crucial for phytoene accumulation in tomato fruit (Nisar et al., 2015). The ubiquitination-mediated degradation of the PSY1 precursor in the cytoplasm, but not that of mature PSY1 in the plastids, regulates carotenoid biosynthesis during tomato fruit ripening (Wang et al., 2020b) (Figure 5B). A RING-type E3 ligase Plastid protein sensing RING E3 ligase 1 (PPSR1) interacts with PSY1 precursor and mediates the latter’s ubiquitination, resulting in its degradation. Besides, protein degradation via ubiquitin–proteasome system also occurs in many other sub-processes of fruit ripening. The ubiquitination of regulatory proteins (e.g. transcription factors) and some pivotal structural proteins (e.g. SlPSY1) is pivotal for normal fruit ripening. Many of these mechanisms seem evolutionarily conserved across plant species. In some cases, ubiquitination and phosphorylation work synergistically to modulate fruit ripening.
Regulation of fruit ripening by other posttranslational mechanisms
Apart from the PTMs described above that can modulate the fruit-ripening process, other regulatory mechanisms, such as N-glycosylation, protein–protein interaction, and protease cleavage, could also provide insight into fruit-ripening control at the posttranslational level. Tomato α-mannosidase (α-Man) and β-D-N-acetylhexosaminidase (β-Hex) are two ripening-specific N-glycan processing enzymes; that is, they respectively cleave the terminal α-mannose and N-acetyl-D-hexosamine residues of N-glycans. Transgenic research has shown that suppressing the expression of α-Man and β-Hex delays fruit softening and prolongs fruit shelf life (Meli et al., 2010). In the nonclimacteric fruit of capsicum (Capsicum annuum), the functions of α-Man and β-Hex in controlling fruit softening are consistent with those in tomato (Ghosh et al., 2011), implying their conserved role in both kinds of fruits. Besides the N-glycan processing enzymes, N-glycosylation of particular proteins also provides evidence in support of its important role during fruit ripening. Xyloglucan endotransglycosylase/hydrolases 1 (XTH1) participates in the fruit ripening process of Fragaria chiloensis, a species of strawberry. Because the biochemical properties and protein stability of FcXTH1 are affected by a deglycosylation treatment, N-glycosylation of FcXTH1 is considered essential for its biological function (Méndez-Yañez et al., 2017). Two ripening-related enzymes in tomato fruit, vacuolar invertase, and polygalacturonase 2A, undergo N-glycosylation during fruit ripening as well (Osteryoung et al., 1990; Tauzin et al., 2014). Recently, a large-scale N-glycoproteome analysis was done to investigate differentially expressed N-glycoproteins and their N-glycosites during tomato fruit ripening. This powerful strategy revealed the landscape of N-glycoproteins during fruit ripening, strengthening our understanding of the potential functions of N-glycoproteins in regulating fruit ripening (Zhang et al., 2020c).
Inhibitor proteins specifically suppress the activities of their target proteins via protein–protein interactions. For example, it is known that invertase inhibitors confer a posttranslational mechanism to silence invertase activity, thereby altering sugar metabolism (Figure 5B). In plants, they can be divided into cell wall and vacuolar invertase inhibitors based on their subcellular localizations and distinctly targeted invertases (Juge, 2006). In tomato, the cell wall invertase inhibitor specifically reduces cell wall invertase activity, while the vacuolar invertase inhibitor interferes with vacuolar invertase exclusively, with both types involved in sugar accumulation in tomato fruit (Jin et al., 2009; Qin et al., 2016). Similarly, vacuolar invertases in pear and peach also influence fruit sugar metabolism by interacting with their respective vacuolar invertases (Ma et al., 2020; Wang et al., 2020c).
The ORANGE (OR) gene is responsible for carotenoid accumulation in various horticultural crops, such as cauliflower (Brassica oleracea), carrot (Daucus carota), and melon (Cucumis melo) fruit (Lu et al., 2006; Tzuri et al., 2015; Ellison et al., 2018). OR encodes a DnaJ cysteine-rich domain-containing protein that locates to the plastids and is currently considered the only known bona fide regulator of chromoplast biogenesis (Sun et al., 2022). In Arabidopsis, AtOR can physically interact with AtPSY in plastids, stabilizing AtPSY to regulate carotenoid biosynthesis (Zhou et al., 2015). Whether this mechanism also applies to fruit crops awaits experimental investigation and validation.
The tomato vacuolar processing enzyme 3 (SlVPE3), a cysteine protease, reportedly regulates fruit ripening in addition to disease resistance (Wang et al., 2017). A comparative proteome analysis detected differentially expressed proteins after silencing SlVPE3. Many ripening-related proteins reduced in abundance were identified in SlVPE3 RNAi fruit, suggesting a role for SlVPE3 in regulating fruit ripening; further, SlVPE3 can directly cleave SlKTI4, a serine proteinase inhibitor, increasing fruit resistance to the fungus Botrytis cinerea (Figure 5B). Although the regulatory role of proteinases in fruit ripening is poorly understood, it nonetheless deserves our attention because proteinases are a huge protein family containing hundreds or even thousands of members in many plant species.
Conclusions
Fruit ripening is a complicated physiological and biological process; its regulation is driven by many levels in a multi-faceted way (Figure 1). According to existing studies, some posttranscriptional regulatory mechanisms are conserved across plant species, such as miRNA-mediated suppression, uORF-mediated translational repression, H3K27me3-mediated repression, ubiquitination-mediated degradation, and so on. These mechanisms, especially those discovered in model fruits, may help us understand the common changes that occur during the ripening process of other fruits. Yet some posttranscriptional regulatory mechanisms seem unique to certain species, for example, the functioning of m6A methylation and lncRNAs. These specialized mechanisms provide new insights into the fruit-ripening process of a given species. With the rapid development of gene-editing technologies and a rising, strong demand by consumers for high-quality fruit, a promising way to improve fruit quality is by manipulating the special regulatory mechanisms of crucial metabolites through transgene-free CRISPR/Cas9 technology. As detailed in “Outstanding Questions,” there are many unresolved problems. However, the rapid development of core technologies and great efforts of researchers worldwide will help us fill these gaps in knowledge in the coming future.
Fruit ripening has been studied extensively at the transcriptional level in recent years. Many transcription factors as well as 5-methylcytosine (5mC) DNA methylation at various gene loci have been identified and proven to be responsible for transcriptional regulation of gene expression during the ripening process.
A series of studies have demonstrated that posttranscriptional, translational, and posttranslational control also play important roles in fruit ripening and fruit quality formation.
Regulatory modes of AS, noncoding RNAs, RNA modification, TE, uORF-mediated translational repression, and protein modification all function after transcription. They tune gene expression at the posttranscriptional level during fruit ripening in a manner that is conserved across species or unique to each.
Do posttranscriptional controls work independently of or synergistically with transcriptional control to form a regulatory network?
What is the effect of m6A methylation on ncRNAs? Do m6A methylated ncRNAs have altered function or stability?
Given that uORF genome-wide identification is difficult during the fruit-ripening process, how can we improve ribosome profiling to effectively apply it to fruit material? Furthermore, might we discover small ORFs or uORFs within lncRNAs as already found in animals? How do translated lncRNAs operate in the regulation of fruit ripening?
Do histone modifications co-transcriptionally affect the m6A installation on renascent RNAs during fruit ripening?
How can posttranscriptional mechanisms be used to improve desirable fruit traits in breeding programs?
S.T. directed the design of the review. W.W. wrote the manuscript and prepared the figures. S.T. revised the manuscript. Y.W., T.C., and G.Q. provided critical discussions.
The author responsible for distribution of materials integral to the findings presented in this article in accordance with the policy described in the Instructions for Authors (https://dbpia.nl.go.kr/plphys/pages/general-instructions) is Shiping Tian ([email protected]).
Acknowledgments
We sincerely apologize to colleagues whose works have not been cited because of space limitation.
Funding
This work was supported by the Beijing Natural Science Foundation (6212024), the National Natural Science Foundation of China (31930086 and 32172638), and the Youth Innovation Promotion Association CAS (2019083).
References
Author notes
Conflict of interest statement. The authors declare that they have no conflict of interest.