-
PDF
- Split View
-
Views
-
Cite
Cite
Alba Arabia, Paula Muñoz, Núria Pallarés, Sergi Munné-Bosch, Experimental approaches in studying active biomolecules modulating fruit ripening: Melatonin as a case study, Plant Physiology, Volume 192, Issue 3, July 2023, Pages 1747–1767, https://doi.org/10.1093/plphys/kiad106
- Share Icon Share
Abstract
Phytohormones are naturally occurring small organic molecules found at low concentrations in plants. They perform essential functions in growth and developmental processes, from organ initiation to senescence, including fruit ripening. These regulatory molecules are studied using different experimental approaches, such as performing exogenous applications, evaluating endogenous levels, and/or obtaining genetically modified lines. Here, we discuss the advantages and limitations of current experimental approaches used to study active biomolecules modulating fruit ripening, focusing on melatonin. Although melatonin has been implicated in fruit ripening in several model fruit crops, current knowledge is affected by the different experimental approaches used, which have given different and sometimes even contradictory results. The methods of application and the doses used have produced different results in studies based on exogenous applications, while different measurement methods and ways of expressing results explain most of the variability in studies using correlative analyses. Furthermore, studies on genetically modified crops have focused on tomato (Solanum lycopersicum L.) plants only. However, TILLING and CRISPR methodologies are becoming essential tools to complement the results from the experimental approaches described above. This will not only help the scientific community better understand the role of melatonin in modulating fruit ripening, but it will also help develop technological advances to improve fruit yield and quality in major crops. The combination of various experimental approaches will undoubtedly lead to a complete understanding of the function of melatonin in fruit ripening in the near future, so that this knowledge can be effectively transferred to the field.
Introduction
Fruit ripening entails multiple coordinated physiological, biochemical, and organoleptic modifications that affect the color, flavor, aroma, and texture of ripe fruits (Giovannoni et al. 2017). Metabolic changes such as starch and cell-wall degradation, chlorophyll loss, as well as the accumulation of free sugars, carotenoids, anthocyanins, and aromas provide the main desirable attributes of fruits (Prasanna et al. 2007). Ripening constitutes a complex physiological process that is highly regulated by both environmental and endogenous signals. Although different biomolecules are involved in modulating ripening, phytohormones (Box 1) and their interactive network represent one of the main endogenous regulators determining the final fruit quality. Phytohormones are small organic molecules that are present at low concentrations in plants and play a key role in signaling. Therefore, the presence of a receptor triggering a cellular signaling cascade and hormonal action are required, together with the short- and/or long-distance transport of the hormones (Davies 2004; Santner et al. 2009). Furthermore, it is not the action of a single phytohormone, but the crosstalk between multiple hormones that regulates a physiological process, making this event even more complex (Fenn and Giovanoni 2021).
Antioxidant: enzyme or low-molecular-weight compound that prevents the oxidation of essential cellular components
Biostimulant: a substance or microorganism that stimulates natural processes in plants to improve either water or nutrient use efficiency, tolerance to abiotic stress, or crop quality and yield
Causation: the relationship between cause and effect; causality
Correlation: statistical measure that expresses the extent to which two variables are linearly related
Derivatization: process of chemically altering an analyte or analytes
Hormonal action: physiological function exerted by a hormone as a result of recognition of a given hormone by its receptor and the subsequent activation of a signaling pathway leading to the activation or repression of gene expression
Hormonal crosstalk: interaction between two or more hormones or hormone pathways in cell signaling at the molecular level
Matrix: components of a sample other than the analyte of interest
Phytohormone: naturally occurring organic compound found at nano- or micromolar concentrations that has a hormonal action at short and/or long distances in plants
Plant growth regulator: chemical used to modify any plant growth or developmental process, such as altering fruit ripening
Veraison: change of color of grape berries that characterizes the onset of ripening
Melatonin (N-acetyl-5-methoxytryptamine) is a multifunctional and pleiotropic indolamine synthesized from tryptophan, with serotonin an essential metabolic intermediary (Back et al. 2016). Since the first report of the presence of melatonin in plants almost three decades ago (Dubbels et al. 1995), much progress has been made in improving our knowledge on its physiological functions in plants. Its presence has been reported in more than 50 plant species (Nawaz et al. 2016), and its involvement in fruit development and ripening has been proposed in several plant models (Arnao and Hernández-Ruiz 2019; Wang et al. 2020b; Ze et al. 2021). However, there is still apparently inconclusive information about its function in the regulation of fruit ripening due to several different and/or controversial results varying according to species, tissue, and method of study.
To unravel and gain a deeper insight into the function of various regulatory biomolecules (Box 2), including melatonin, studies have been performed using different experimental approaches (Fig. 1). First, exogenous applications of a biomolecule can determine if it has a promoting or inhibitory role in a given physiological process. However, this response is dose-dependent and may differ depending on the method of application and the developmental stage at which the biomolecule is applied, resulting in different phenotypes and, consequently, different interpretations of its role. Second, an evaluation of the endogenous concentration of the molecule under study is highly needed to quantify organ and tissue levels, even at different points of time throughout the process, to establish possible causation. Finally, generating specific transgenic lines by genetic engineering based on the silencing or overexpression of genes related to the biosynthesis or signal transduction of the molecule under study is required to determine the degree of relevance of the molecule in the process. The use of only one approach could lead to inconclusive results, making it difficult to draw critical conclusions and transfer them to the field.
Phytohormones are small organic molecules synthesized de novo by plants. They have the ability to regulate physiological processes at much lower concentrations than nutrients or vitamins (<1 µM). Phytohormones act as chemical messengers, coordinating multiple cellular processes (Davies 2004). They can be transported to their site of action by transporters to regulate their distribution. Likewise, the effects of the hormones are determined by the sensitivity or responsiveness of plant tissues to hormones. Thus, to be considered a plant hormone, the presence of receptors and signal-transduction components are needed. These receptors must show a specific and reversible binding of the hormone, consequently inducing a biological response. Melatonin is a natural indoleamine molecule that was identified in plants in 1995 (Dubbels et al. 1995). Since then, much progress has been made in determining the melatonin biosynthetic routes as well as the products of its catabolism (Back et al. 2016; Lee and Back 2021). Its presence has been detected in a wide variety of plant species, organs, and tissues, thus making it ubiquitous (Nawaz et al. 2016). Furthermore, melatonin is involved in the regulation of several physiological plant processes (Lee et al. 2014; Sun et al. 2015; Zhang et al. 2018; Ye et al. 2020; Gholami et al. 2022). Recently, a melatonin putative receptor, Cand2/PMTR1, was identified in A. thaliana. It localizes to the plasma membrane, interacting with the alpha subunit of the G protein (GPA1) (Wei et al. 2018). Despite this, the signal transduction mechanism of melatonin has not been identified yet. Furthermore, there is still a lack of knowledge about fruit melatonin receptors, as well as the melatonin signaling process and the mechanisms for melatonin transport. Melatonin can be classified as a hormone-like compound, but we still do not know enough about it to consider it a hormone in fruit ripening regulation (see Box 2 Figure).

Regulatory plant molecules, their chemical structure, and classification as hormones, hormone-like compounds, and other regulatory molecules.
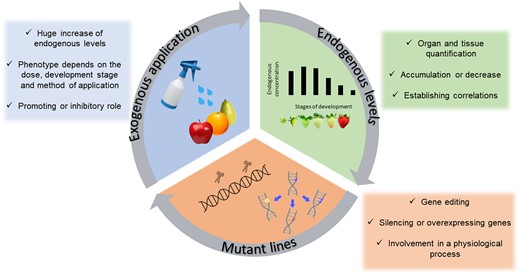
Diagrammatic representation of the three experimental approaches used to study active biomolecules in plants.
Here, we discuss different experimental methods determining the function of active molecules in modulating fruit ripening, considering their advantages and limitations, and focusing on the example of melatonin. In addition to describing the experimental approaches, we will discuss the need to include, or at least take into consideration, all the information from the three experimental approaches to achieve a complete and better understanding of the physiological importance of melatonin. An integration of all this knowledge will be essential in boosting technological applications in agrifood biotechnology and agronomy to improve fruit yield and quality.
Exogenous applications: spray and pray
Plant growth regulators have been classically used for a plethora of biotechnological applications since their discovery. More recently, they have been replaced by plant biostimulants in modern biotechnology (Rouphael and Colla 2020; Giordano et al. 2022). Melatonin is a good example of the worldwide popularity of biostimulants. However, since its use, there has been a focus on “what melatonin does” instead of “how it does it”, overlooking its mechanisms of action. Nowadays, important advances are being made in discovering the physiological role of melatonin in plants, which will undoubtedly be used to achieve a more suitable use of this biostimulant (Yakhin et al. 2017; Sudiro et al. 2022).
Over the last few years, several studies based on exogenous applications have been performed to study the biostimulatory effect of melatonin, revealing that it is a signal molecule involved in the regulation of numerous physiological processes (Murch et al. 2009; Lee et al. 2014; Sun et al. 2015; Arnao and Hernández-Ruiz 2020; Ye et al. 2020). Multiple experiments have demonstrated that the exogenous application of melatonin enhances several traits of interest in plants such as productivity (Liu et al. 2016), resistance to biotic and abiotic factors (Aghdam and Fard 2017; Gholami et al. 2022), tolerance to heavy metals (Nawaz et al. 2018), as well as improvements in fruit quality and shelf-life (Zhang et al. 2018). Several studies have carried out exogenous applications of melatonin both in pre- and postharvest fruits. In this regard, melatonin application has been described to contribute to the regulation of biochemical and physiological changes that preserve many quality attributes, enhancing postharvest shelf-life, and inducing changes in the ripening rate (Table 1).
Exogenous applications of melatonin carried out during pre- and postharvest of fruits
Fruit . | Developmental stage . | Method . | Applied concentration (M) . | Main effects . | References . |
---|---|---|---|---|---|
Preharvest | |||||
Apple | Green | Spray | 5×10−4 | Accelerated ripening (ethylene) and improved quality (↑ fruit size, weight, sugars, and firmness) | Verde et al. (2022) |
Cherry | Green large size | Spray | 10−5 | Delayed ripening (↓ anthocyanins) | Tijero et al. (2019) |
During fruit growth cycle | Spray | 10−4 | Maintained postharvest quality properties | Carrión-Antolí et al. (2022) | |
Grape | Pre-veraison | Spray | 4×10−4 | Increased fruit weight (mechanism unclear) | Meng et al. (2015) |
Onset of veraison | Dipping, 10 s | 10−4 | Enhanced antioxidant capacity (↑ anthocyanins, phenols, flavonoids, and proanthocyanidins) | Xu et al. (2017) | |
60 d after full bloom | Dipping, 10 s | 10−4 | Accelerated ripening (↑ ABA, H2O2 and ethylene) | Xu et al. (2018) | |
70 d after full bloom | Dipping, 5 s | 5×10−5 | Accelerated ripening (↑ ethylene), improved quality (phenylpropanoids and lipids) | Ma et al. (2021) | |
During veraison | Spray | 10−4 | Improved quality (↑ sugars, N, K, Cu, Fe, Zn) | Xia et al. (2021) | |
Pear | Anthesis | Spray | 10−4 | ↑ Parthenocarpy | Liu et al. (2018b) |
20 d after pollination | Spray | 10−4 | Increased fruit weight and quality (↑ photosynthetic efficiency and ↑ sugars) | Liu et al. (2019c) | |
100 d after blooming | Spray | 5×10−5; 2×10−4 | Increased anthocyanins and flavonols concentrations | Sun et al. (2021b) | |
Strawberry (Fragaria × ananassa) | Light green | Injection | 10−5 | Limited effect on ripening | Mansouri et al. (2021) |
Light green | Injection | 10−3 | Accelerated ripening (↑ ABA and H2O2) | ||
Apricot | During fruit growth cycle | Spray | 10−4 | Delayed postharvest ripening (↓ethylene) Maintained quality properties during storage | Medina-Santamarina et al. (2021a, b) |
Pomegranate | During fruit growth cycle | Spray | 10−4 | Increased fruit crop yield and quality Maintained postharvest quality properties | Medina-Santamarina et al. (2021a, b) |
Tomato | Plants at fifth true leaf stage up to fruit set | Spray | 10−4 | Improved defenses against abiotic stress (simulated acid rain) | Debnath et al. (2018) |
Postharvest | |||||
Apple | Commercial maturity | Spray | 10−3 | Delayed ripening (↓ ethylene) and maintained quality | Onik et al. (2021) |
Commercial maturity | Dipping, 10 min | 5×10−5 | Delayed ripening (↓ ethylene and respiratory intensity) and maintained quality (↑ firmness, ascorbic acid, TSS, and TA) | Fan et al. (2022b) | |
Immature green stage | Dipping, 2 h | 5×10−5 | Improved quality and stimulate ethylene production | Verde et al. (2023) | |
Banana | Mature unripe | Dipping, 2 h | 5×10−5; 2×10−5 | Delayed ripening (↓ ethylene) | Hu et al. (2017) |
Commercial maturity | Dipping, 3 min | 10−5 | Improved defenses against biotic stress (Colletotrichum musae) | Li et al. (2019) | |
Cherry | Commercial maturity | Dipping, 5 min | 10−4 | Senescence inhibition and maintained quality (↓ weight loss and decay incidence, ↑ firmness, TSS, and TA) | Wang et al. (2019a) |
Kiwi fruit | Physiological maturity | Dipping, 30 min | 10−4 | Senescence inhibition (↑ antioxidants and ↓ MDA) | Wang et al. (2019b) |
Physiological maturity | Dipping, 10 min | 10−4 | Delayed ripening (↓ ethylene, respiration rate) and maintained quality (reduced ethanol production) | Cheng et al. (2022) | |
Physiological maturity | Dipping, 10 min | 5×10−4 | Delayed ripening (↓ ethylene, respiration rate) and maintained quality (↑firmness, ascorbic acid, and TA) but reduced TSS | Luo et al. (2022) | |
Lychee fruit | Commercial maturity | Dipping, 5 min | 4×10−4 | Senescence inhibition (↓ H2O2, O2−, and MDA) | Zhang et al. (2018) |
Mango | Mature green | Dipping, 1 h | 5×10−4 | Delayed ripening (↓ ethylene and ABA) | Liu et al. (2020) |
70% matured | Dipping, 30 min | 2·10−4 | Delayed ripening and maintained membrane integrity (↓ H2O2 and MDA) | Dong et al. (2021) | |
Papaya | Breaker | Dipping, 2 h | 4×10−4 | Improved defenses against biotic stress (Colletotrichum spp.) | Fan et al. (2022a) |
Peach | Commercial maturity | Dipping, 10 min | 10−4 | Senescence inhibition (↓ ROS) | Gao et al. (2016) |
Pear | Commercial maturity | Dipping, 12 h | 10−4 | Senescence inhibition (↓ ethylene) | Liu et al. (2019b) |
Before breaker | Dipping, 1 h | 2·10−4 | ↑anthocyanins and anthocyanin biosynthesis genes ↑ H2O2 | Sun et al. (2021a) | |
Commercial maturity | Dipping, 6 h | 10−4 | Improved defenses against biotic stress (Botryosphaeria dothidea) | Wang et al. (2022) | |
Plum | Commercial maturity | Dipping | 10−4; 10−3 | Improved cold tolerance (h ascorbic acid, phenols, and antioxidant activity) | Bal (2019) |
Commercial maturity | Spray | 10−4 | Improved post-harvest life (↑ anthocyanin and phenol content) | Arabia et al. (2022) | |
Green mature | Dipping, 1 h | 5×10−5; 10−4 | Delayed ripening (↑ firmness, ↓ respiration rate, and ethylene) | Yan et al. (2022) | |
Pomegranate | Commercial maturity | Dipping, 15 min | 10−4 | Improved cold tolerance (↓ MDA and H2O2) | Jannatizadeh (2019) |
Strawberry (Fragaria × ananassa) | Commercial maturity | Dipping, 5 min | 10−4 | Improved cold tolerance (↑ GABA-T enzyme activity) | Aghdam and Fard (2017) |
Commercial maturity | Dipping, 5 min | 10−4; 10−3 | Senescence inhibition and improved cold tolerance (↑ phenols and flavonoids, ↓ MDA and H2O2) | Liu et al. (2018a, b) | |
Commercial maturity | Dipping, 5 min | 10−4 | Senescence inhibition (↓ weight loss and decay incidence) | El-Mogy et al. (2019) | |
Tomato | Green | Dipping, 2 h | 5×10−5 | Ripening acceleration and improved quality (↑ ethylene and lycopene) | Sun et al. (2015) |
Mature green | Dipping, 5 min | 10−4 | Improved cold tolerance (↑ polyamines, proline and NO) | Aghdam et al. (2019) | |
Mature green | Dipping, 5 min | 10−4 | Improved cold tolerance (↑ H2O2 and GABA shunt pathway) | Sharafi et al. (2019) | |
Breaker | Dipping, 2 h | 5×10−5 | Improved quality (↑ αβ-carotene and lycopene) | Sun et al. (2020) | |
Mature green | Dipping, 10 min | 5×10−5 | improved quality (↑ lycopene) and increased demethylase activity | Shan et al. (2022) |
Fruit . | Developmental stage . | Method . | Applied concentration (M) . | Main effects . | References . |
---|---|---|---|---|---|
Preharvest | |||||
Apple | Green | Spray | 5×10−4 | Accelerated ripening (ethylene) and improved quality (↑ fruit size, weight, sugars, and firmness) | Verde et al. (2022) |
Cherry | Green large size | Spray | 10−5 | Delayed ripening (↓ anthocyanins) | Tijero et al. (2019) |
During fruit growth cycle | Spray | 10−4 | Maintained postharvest quality properties | Carrión-Antolí et al. (2022) | |
Grape | Pre-veraison | Spray | 4×10−4 | Increased fruit weight (mechanism unclear) | Meng et al. (2015) |
Onset of veraison | Dipping, 10 s | 10−4 | Enhanced antioxidant capacity (↑ anthocyanins, phenols, flavonoids, and proanthocyanidins) | Xu et al. (2017) | |
60 d after full bloom | Dipping, 10 s | 10−4 | Accelerated ripening (↑ ABA, H2O2 and ethylene) | Xu et al. (2018) | |
70 d after full bloom | Dipping, 5 s | 5×10−5 | Accelerated ripening (↑ ethylene), improved quality (phenylpropanoids and lipids) | Ma et al. (2021) | |
During veraison | Spray | 10−4 | Improved quality (↑ sugars, N, K, Cu, Fe, Zn) | Xia et al. (2021) | |
Pear | Anthesis | Spray | 10−4 | ↑ Parthenocarpy | Liu et al. (2018b) |
20 d after pollination | Spray | 10−4 | Increased fruit weight and quality (↑ photosynthetic efficiency and ↑ sugars) | Liu et al. (2019c) | |
100 d after blooming | Spray | 5×10−5; 2×10−4 | Increased anthocyanins and flavonols concentrations | Sun et al. (2021b) | |
Strawberry (Fragaria × ananassa) | Light green | Injection | 10−5 | Limited effect on ripening | Mansouri et al. (2021) |
Light green | Injection | 10−3 | Accelerated ripening (↑ ABA and H2O2) | ||
Apricot | During fruit growth cycle | Spray | 10−4 | Delayed postharvest ripening (↓ethylene) Maintained quality properties during storage | Medina-Santamarina et al. (2021a, b) |
Pomegranate | During fruit growth cycle | Spray | 10−4 | Increased fruit crop yield and quality Maintained postharvest quality properties | Medina-Santamarina et al. (2021a, b) |
Tomato | Plants at fifth true leaf stage up to fruit set | Spray | 10−4 | Improved defenses against abiotic stress (simulated acid rain) | Debnath et al. (2018) |
Postharvest | |||||
Apple | Commercial maturity | Spray | 10−3 | Delayed ripening (↓ ethylene) and maintained quality | Onik et al. (2021) |
Commercial maturity | Dipping, 10 min | 5×10−5 | Delayed ripening (↓ ethylene and respiratory intensity) and maintained quality (↑ firmness, ascorbic acid, TSS, and TA) | Fan et al. (2022b) | |
Immature green stage | Dipping, 2 h | 5×10−5 | Improved quality and stimulate ethylene production | Verde et al. (2023) | |
Banana | Mature unripe | Dipping, 2 h | 5×10−5; 2×10−5 | Delayed ripening (↓ ethylene) | Hu et al. (2017) |
Commercial maturity | Dipping, 3 min | 10−5 | Improved defenses against biotic stress (Colletotrichum musae) | Li et al. (2019) | |
Cherry | Commercial maturity | Dipping, 5 min | 10−4 | Senescence inhibition and maintained quality (↓ weight loss and decay incidence, ↑ firmness, TSS, and TA) | Wang et al. (2019a) |
Kiwi fruit | Physiological maturity | Dipping, 30 min | 10−4 | Senescence inhibition (↑ antioxidants and ↓ MDA) | Wang et al. (2019b) |
Physiological maturity | Dipping, 10 min | 10−4 | Delayed ripening (↓ ethylene, respiration rate) and maintained quality (reduced ethanol production) | Cheng et al. (2022) | |
Physiological maturity | Dipping, 10 min | 5×10−4 | Delayed ripening (↓ ethylene, respiration rate) and maintained quality (↑firmness, ascorbic acid, and TA) but reduced TSS | Luo et al. (2022) | |
Lychee fruit | Commercial maturity | Dipping, 5 min | 4×10−4 | Senescence inhibition (↓ H2O2, O2−, and MDA) | Zhang et al. (2018) |
Mango | Mature green | Dipping, 1 h | 5×10−4 | Delayed ripening (↓ ethylene and ABA) | Liu et al. (2020) |
70% matured | Dipping, 30 min | 2·10−4 | Delayed ripening and maintained membrane integrity (↓ H2O2 and MDA) | Dong et al. (2021) | |
Papaya | Breaker | Dipping, 2 h | 4×10−4 | Improved defenses against biotic stress (Colletotrichum spp.) | Fan et al. (2022a) |
Peach | Commercial maturity | Dipping, 10 min | 10−4 | Senescence inhibition (↓ ROS) | Gao et al. (2016) |
Pear | Commercial maturity | Dipping, 12 h | 10−4 | Senescence inhibition (↓ ethylene) | Liu et al. (2019b) |
Before breaker | Dipping, 1 h | 2·10−4 | ↑anthocyanins and anthocyanin biosynthesis genes ↑ H2O2 | Sun et al. (2021a) | |
Commercial maturity | Dipping, 6 h | 10−4 | Improved defenses against biotic stress (Botryosphaeria dothidea) | Wang et al. (2022) | |
Plum | Commercial maturity | Dipping | 10−4; 10−3 | Improved cold tolerance (h ascorbic acid, phenols, and antioxidant activity) | Bal (2019) |
Commercial maturity | Spray | 10−4 | Improved post-harvest life (↑ anthocyanin and phenol content) | Arabia et al. (2022) | |
Green mature | Dipping, 1 h | 5×10−5; 10−4 | Delayed ripening (↑ firmness, ↓ respiration rate, and ethylene) | Yan et al. (2022) | |
Pomegranate | Commercial maturity | Dipping, 15 min | 10−4 | Improved cold tolerance (↓ MDA and H2O2) | Jannatizadeh (2019) |
Strawberry (Fragaria × ananassa) | Commercial maturity | Dipping, 5 min | 10−4 | Improved cold tolerance (↑ GABA-T enzyme activity) | Aghdam and Fard (2017) |
Commercial maturity | Dipping, 5 min | 10−4; 10−3 | Senescence inhibition and improved cold tolerance (↑ phenols and flavonoids, ↓ MDA and H2O2) | Liu et al. (2018a, b) | |
Commercial maturity | Dipping, 5 min | 10−4 | Senescence inhibition (↓ weight loss and decay incidence) | El-Mogy et al. (2019) | |
Tomato | Green | Dipping, 2 h | 5×10−5 | Ripening acceleration and improved quality (↑ ethylene and lycopene) | Sun et al. (2015) |
Mature green | Dipping, 5 min | 10−4 | Improved cold tolerance (↑ polyamines, proline and NO) | Aghdam et al. (2019) | |
Mature green | Dipping, 5 min | 10−4 | Improved cold tolerance (↑ H2O2 and GABA shunt pathway) | Sharafi et al. (2019) | |
Breaker | Dipping, 2 h | 5×10−5 | Improved quality (↑ αβ-carotene and lycopene) | Sun et al. (2020) | |
Mature green | Dipping, 10 min | 5×10−5 | improved quality (↑ lycopene) and increased demethylase activity | Shan et al. (2022) |
The developmental stage during application, the method used, the concentration applied, and the main effects are shown.
ABA, abscisic acid; GABA, γ-aminobutyric acid; MDA, malondialdehyde; NO, nitric oxide; ROS, reactive oxygen species; TA, titratable acid; TSS, total soluble solids.
Exogenous applications of melatonin carried out during pre- and postharvest of fruits
Fruit . | Developmental stage . | Method . | Applied concentration (M) . | Main effects . | References . |
---|---|---|---|---|---|
Preharvest | |||||
Apple | Green | Spray | 5×10−4 | Accelerated ripening (ethylene) and improved quality (↑ fruit size, weight, sugars, and firmness) | Verde et al. (2022) |
Cherry | Green large size | Spray | 10−5 | Delayed ripening (↓ anthocyanins) | Tijero et al. (2019) |
During fruit growth cycle | Spray | 10−4 | Maintained postharvest quality properties | Carrión-Antolí et al. (2022) | |
Grape | Pre-veraison | Spray | 4×10−4 | Increased fruit weight (mechanism unclear) | Meng et al. (2015) |
Onset of veraison | Dipping, 10 s | 10−4 | Enhanced antioxidant capacity (↑ anthocyanins, phenols, flavonoids, and proanthocyanidins) | Xu et al. (2017) | |
60 d after full bloom | Dipping, 10 s | 10−4 | Accelerated ripening (↑ ABA, H2O2 and ethylene) | Xu et al. (2018) | |
70 d after full bloom | Dipping, 5 s | 5×10−5 | Accelerated ripening (↑ ethylene), improved quality (phenylpropanoids and lipids) | Ma et al. (2021) | |
During veraison | Spray | 10−4 | Improved quality (↑ sugars, N, K, Cu, Fe, Zn) | Xia et al. (2021) | |
Pear | Anthesis | Spray | 10−4 | ↑ Parthenocarpy | Liu et al. (2018b) |
20 d after pollination | Spray | 10−4 | Increased fruit weight and quality (↑ photosynthetic efficiency and ↑ sugars) | Liu et al. (2019c) | |
100 d after blooming | Spray | 5×10−5; 2×10−4 | Increased anthocyanins and flavonols concentrations | Sun et al. (2021b) | |
Strawberry (Fragaria × ananassa) | Light green | Injection | 10−5 | Limited effect on ripening | Mansouri et al. (2021) |
Light green | Injection | 10−3 | Accelerated ripening (↑ ABA and H2O2) | ||
Apricot | During fruit growth cycle | Spray | 10−4 | Delayed postharvest ripening (↓ethylene) Maintained quality properties during storage | Medina-Santamarina et al. (2021a, b) |
Pomegranate | During fruit growth cycle | Spray | 10−4 | Increased fruit crop yield and quality Maintained postharvest quality properties | Medina-Santamarina et al. (2021a, b) |
Tomato | Plants at fifth true leaf stage up to fruit set | Spray | 10−4 | Improved defenses against abiotic stress (simulated acid rain) | Debnath et al. (2018) |
Postharvest | |||||
Apple | Commercial maturity | Spray | 10−3 | Delayed ripening (↓ ethylene) and maintained quality | Onik et al. (2021) |
Commercial maturity | Dipping, 10 min | 5×10−5 | Delayed ripening (↓ ethylene and respiratory intensity) and maintained quality (↑ firmness, ascorbic acid, TSS, and TA) | Fan et al. (2022b) | |
Immature green stage | Dipping, 2 h | 5×10−5 | Improved quality and stimulate ethylene production | Verde et al. (2023) | |
Banana | Mature unripe | Dipping, 2 h | 5×10−5; 2×10−5 | Delayed ripening (↓ ethylene) | Hu et al. (2017) |
Commercial maturity | Dipping, 3 min | 10−5 | Improved defenses against biotic stress (Colletotrichum musae) | Li et al. (2019) | |
Cherry | Commercial maturity | Dipping, 5 min | 10−4 | Senescence inhibition and maintained quality (↓ weight loss and decay incidence, ↑ firmness, TSS, and TA) | Wang et al. (2019a) |
Kiwi fruit | Physiological maturity | Dipping, 30 min | 10−4 | Senescence inhibition (↑ antioxidants and ↓ MDA) | Wang et al. (2019b) |
Physiological maturity | Dipping, 10 min | 10−4 | Delayed ripening (↓ ethylene, respiration rate) and maintained quality (reduced ethanol production) | Cheng et al. (2022) | |
Physiological maturity | Dipping, 10 min | 5×10−4 | Delayed ripening (↓ ethylene, respiration rate) and maintained quality (↑firmness, ascorbic acid, and TA) but reduced TSS | Luo et al. (2022) | |
Lychee fruit | Commercial maturity | Dipping, 5 min | 4×10−4 | Senescence inhibition (↓ H2O2, O2−, and MDA) | Zhang et al. (2018) |
Mango | Mature green | Dipping, 1 h | 5×10−4 | Delayed ripening (↓ ethylene and ABA) | Liu et al. (2020) |
70% matured | Dipping, 30 min | 2·10−4 | Delayed ripening and maintained membrane integrity (↓ H2O2 and MDA) | Dong et al. (2021) | |
Papaya | Breaker | Dipping, 2 h | 4×10−4 | Improved defenses against biotic stress (Colletotrichum spp.) | Fan et al. (2022a) |
Peach | Commercial maturity | Dipping, 10 min | 10−4 | Senescence inhibition (↓ ROS) | Gao et al. (2016) |
Pear | Commercial maturity | Dipping, 12 h | 10−4 | Senescence inhibition (↓ ethylene) | Liu et al. (2019b) |
Before breaker | Dipping, 1 h | 2·10−4 | ↑anthocyanins and anthocyanin biosynthesis genes ↑ H2O2 | Sun et al. (2021a) | |
Commercial maturity | Dipping, 6 h | 10−4 | Improved defenses against biotic stress (Botryosphaeria dothidea) | Wang et al. (2022) | |
Plum | Commercial maturity | Dipping | 10−4; 10−3 | Improved cold tolerance (h ascorbic acid, phenols, and antioxidant activity) | Bal (2019) |
Commercial maturity | Spray | 10−4 | Improved post-harvest life (↑ anthocyanin and phenol content) | Arabia et al. (2022) | |
Green mature | Dipping, 1 h | 5×10−5; 10−4 | Delayed ripening (↑ firmness, ↓ respiration rate, and ethylene) | Yan et al. (2022) | |
Pomegranate | Commercial maturity | Dipping, 15 min | 10−4 | Improved cold tolerance (↓ MDA and H2O2) | Jannatizadeh (2019) |
Strawberry (Fragaria × ananassa) | Commercial maturity | Dipping, 5 min | 10−4 | Improved cold tolerance (↑ GABA-T enzyme activity) | Aghdam and Fard (2017) |
Commercial maturity | Dipping, 5 min | 10−4; 10−3 | Senescence inhibition and improved cold tolerance (↑ phenols and flavonoids, ↓ MDA and H2O2) | Liu et al. (2018a, b) | |
Commercial maturity | Dipping, 5 min | 10−4 | Senescence inhibition (↓ weight loss and decay incidence) | El-Mogy et al. (2019) | |
Tomato | Green | Dipping, 2 h | 5×10−5 | Ripening acceleration and improved quality (↑ ethylene and lycopene) | Sun et al. (2015) |
Mature green | Dipping, 5 min | 10−4 | Improved cold tolerance (↑ polyamines, proline and NO) | Aghdam et al. (2019) | |
Mature green | Dipping, 5 min | 10−4 | Improved cold tolerance (↑ H2O2 and GABA shunt pathway) | Sharafi et al. (2019) | |
Breaker | Dipping, 2 h | 5×10−5 | Improved quality (↑ αβ-carotene and lycopene) | Sun et al. (2020) | |
Mature green | Dipping, 10 min | 5×10−5 | improved quality (↑ lycopene) and increased demethylase activity | Shan et al. (2022) |
Fruit . | Developmental stage . | Method . | Applied concentration (M) . | Main effects . | References . |
---|---|---|---|---|---|
Preharvest | |||||
Apple | Green | Spray | 5×10−4 | Accelerated ripening (ethylene) and improved quality (↑ fruit size, weight, sugars, and firmness) | Verde et al. (2022) |
Cherry | Green large size | Spray | 10−5 | Delayed ripening (↓ anthocyanins) | Tijero et al. (2019) |
During fruit growth cycle | Spray | 10−4 | Maintained postharvest quality properties | Carrión-Antolí et al. (2022) | |
Grape | Pre-veraison | Spray | 4×10−4 | Increased fruit weight (mechanism unclear) | Meng et al. (2015) |
Onset of veraison | Dipping, 10 s | 10−4 | Enhanced antioxidant capacity (↑ anthocyanins, phenols, flavonoids, and proanthocyanidins) | Xu et al. (2017) | |
60 d after full bloom | Dipping, 10 s | 10−4 | Accelerated ripening (↑ ABA, H2O2 and ethylene) | Xu et al. (2018) | |
70 d after full bloom | Dipping, 5 s | 5×10−5 | Accelerated ripening (↑ ethylene), improved quality (phenylpropanoids and lipids) | Ma et al. (2021) | |
During veraison | Spray | 10−4 | Improved quality (↑ sugars, N, K, Cu, Fe, Zn) | Xia et al. (2021) | |
Pear | Anthesis | Spray | 10−4 | ↑ Parthenocarpy | Liu et al. (2018b) |
20 d after pollination | Spray | 10−4 | Increased fruit weight and quality (↑ photosynthetic efficiency and ↑ sugars) | Liu et al. (2019c) | |
100 d after blooming | Spray | 5×10−5; 2×10−4 | Increased anthocyanins and flavonols concentrations | Sun et al. (2021b) | |
Strawberry (Fragaria × ananassa) | Light green | Injection | 10−5 | Limited effect on ripening | Mansouri et al. (2021) |
Light green | Injection | 10−3 | Accelerated ripening (↑ ABA and H2O2) | ||
Apricot | During fruit growth cycle | Spray | 10−4 | Delayed postharvest ripening (↓ethylene) Maintained quality properties during storage | Medina-Santamarina et al. (2021a, b) |
Pomegranate | During fruit growth cycle | Spray | 10−4 | Increased fruit crop yield and quality Maintained postharvest quality properties | Medina-Santamarina et al. (2021a, b) |
Tomato | Plants at fifth true leaf stage up to fruit set | Spray | 10−4 | Improved defenses against abiotic stress (simulated acid rain) | Debnath et al. (2018) |
Postharvest | |||||
Apple | Commercial maturity | Spray | 10−3 | Delayed ripening (↓ ethylene) and maintained quality | Onik et al. (2021) |
Commercial maturity | Dipping, 10 min | 5×10−5 | Delayed ripening (↓ ethylene and respiratory intensity) and maintained quality (↑ firmness, ascorbic acid, TSS, and TA) | Fan et al. (2022b) | |
Immature green stage | Dipping, 2 h | 5×10−5 | Improved quality and stimulate ethylene production | Verde et al. (2023) | |
Banana | Mature unripe | Dipping, 2 h | 5×10−5; 2×10−5 | Delayed ripening (↓ ethylene) | Hu et al. (2017) |
Commercial maturity | Dipping, 3 min | 10−5 | Improved defenses against biotic stress (Colletotrichum musae) | Li et al. (2019) | |
Cherry | Commercial maturity | Dipping, 5 min | 10−4 | Senescence inhibition and maintained quality (↓ weight loss and decay incidence, ↑ firmness, TSS, and TA) | Wang et al. (2019a) |
Kiwi fruit | Physiological maturity | Dipping, 30 min | 10−4 | Senescence inhibition (↑ antioxidants and ↓ MDA) | Wang et al. (2019b) |
Physiological maturity | Dipping, 10 min | 10−4 | Delayed ripening (↓ ethylene, respiration rate) and maintained quality (reduced ethanol production) | Cheng et al. (2022) | |
Physiological maturity | Dipping, 10 min | 5×10−4 | Delayed ripening (↓ ethylene, respiration rate) and maintained quality (↑firmness, ascorbic acid, and TA) but reduced TSS | Luo et al. (2022) | |
Lychee fruit | Commercial maturity | Dipping, 5 min | 4×10−4 | Senescence inhibition (↓ H2O2, O2−, and MDA) | Zhang et al. (2018) |
Mango | Mature green | Dipping, 1 h | 5×10−4 | Delayed ripening (↓ ethylene and ABA) | Liu et al. (2020) |
70% matured | Dipping, 30 min | 2·10−4 | Delayed ripening and maintained membrane integrity (↓ H2O2 and MDA) | Dong et al. (2021) | |
Papaya | Breaker | Dipping, 2 h | 4×10−4 | Improved defenses against biotic stress (Colletotrichum spp.) | Fan et al. (2022a) |
Peach | Commercial maturity | Dipping, 10 min | 10−4 | Senescence inhibition (↓ ROS) | Gao et al. (2016) |
Pear | Commercial maturity | Dipping, 12 h | 10−4 | Senescence inhibition (↓ ethylene) | Liu et al. (2019b) |
Before breaker | Dipping, 1 h | 2·10−4 | ↑anthocyanins and anthocyanin biosynthesis genes ↑ H2O2 | Sun et al. (2021a) | |
Commercial maturity | Dipping, 6 h | 10−4 | Improved defenses against biotic stress (Botryosphaeria dothidea) | Wang et al. (2022) | |
Plum | Commercial maturity | Dipping | 10−4; 10−3 | Improved cold tolerance (h ascorbic acid, phenols, and antioxidant activity) | Bal (2019) |
Commercial maturity | Spray | 10−4 | Improved post-harvest life (↑ anthocyanin and phenol content) | Arabia et al. (2022) | |
Green mature | Dipping, 1 h | 5×10−5; 10−4 | Delayed ripening (↑ firmness, ↓ respiration rate, and ethylene) | Yan et al. (2022) | |
Pomegranate | Commercial maturity | Dipping, 15 min | 10−4 | Improved cold tolerance (↓ MDA and H2O2) | Jannatizadeh (2019) |
Strawberry (Fragaria × ananassa) | Commercial maturity | Dipping, 5 min | 10−4 | Improved cold tolerance (↑ GABA-T enzyme activity) | Aghdam and Fard (2017) |
Commercial maturity | Dipping, 5 min | 10−4; 10−3 | Senescence inhibition and improved cold tolerance (↑ phenols and flavonoids, ↓ MDA and H2O2) | Liu et al. (2018a, b) | |
Commercial maturity | Dipping, 5 min | 10−4 | Senescence inhibition (↓ weight loss and decay incidence) | El-Mogy et al. (2019) | |
Tomato | Green | Dipping, 2 h | 5×10−5 | Ripening acceleration and improved quality (↑ ethylene and lycopene) | Sun et al. (2015) |
Mature green | Dipping, 5 min | 10−4 | Improved cold tolerance (↑ polyamines, proline and NO) | Aghdam et al. (2019) | |
Mature green | Dipping, 5 min | 10−4 | Improved cold tolerance (↑ H2O2 and GABA shunt pathway) | Sharafi et al. (2019) | |
Breaker | Dipping, 2 h | 5×10−5 | Improved quality (↑ αβ-carotene and lycopene) | Sun et al. (2020) | |
Mature green | Dipping, 10 min | 5×10−5 | improved quality (↑ lycopene) and increased demethylase activity | Shan et al. (2022) |
The developmental stage during application, the method used, the concentration applied, and the main effects are shown.
ABA, abscisic acid; GABA, γ-aminobutyric acid; MDA, malondialdehyde; NO, nitric oxide; ROS, reactive oxygen species; TA, titratable acid; TSS, total soluble solids.
Generally, the dose of exogenous melatonin applied to fruits has been 10−3 to 10−5 M in both pre- and postharvest studies. However, varying effects of melatonin have been reported. During preharvest, in climacteric fruits such as apples (Malus domestica Borkh.), the exogenous application of 10−4 M melatonin at the green stage just before the onset of ripening has been reported to cause an acceleration in ripening parameters such as ethylene levels, sugar concentrations, weight, color, and firmness (Verde et al. 2022). Likewise, the application of 10−4 M melatonin in Zaosu pears (Pyrus bretschneideri Rehd.) has been demonstrated to cause a higher accumulation of sugars at the ripening stage (Liu et al. 2019c). On the contrary, melatonin applied at 10−5 M on nonclimacteric fruits such as sweet cherries (Prunus avium L.) at the green stage in a tree orchard inhibited ripening by delaying anthocyanin accumulation and increasing the cytokinin content (Tijero et al. 2019). The same effect was observed when melatonin was exogenously applied at 10−5 M to strawberries (Fragaria × ananassa Duch.) at the green stage, since it delayed their ripening (Mansouri et al. 2021). However, when melatonin was applied at 10−3 M, this accelerated the ripening of the strawberries by increasing the levels of ABA, hydrogen peroxide (H2O2), and anthocyanins. Moreover, in contrast to the effects of melatonin on cherries and strawberries, melatonin applied at 10−5 M and at 10−4 M promoted grape (Vitis vinifera L.) berry ripening by increasing the levels of ABA, ethylene, and H2O2 (Xu et al. 2018).
Changes in ripening induced by melatonin treatment during postharvest life have also been studied, particularly in climacteric fruits. In tomatoes, the exogenous application of melatonin at 5×10−5 M at the green mature stage was shown to induce ethylene and carotenoid biosynthesis as well as cell-wall modifications, demonstrating accelerated ripening (Sun et al. 2015, 2020). By contrast, when plums (Prunus salicina L.) were harvested at the green mature stage and treated with melatonin at 5×10−5 M and 10−4 M, their ripening slowed, as indicated by the firmness, respiration rate, and ethylene production (Yan et al. 2022). Similarly, it has been described that the application of melatonin at a concentration of 2×10−4 M and 5×10−4 M to mature unripe bananas (Musa acuminata L.) represses the expression of 1-AMINOCYCLOPROPANE-1-CARBOXYLIC ACID OXYDASE 1 (MaACO1) and 1-AMINOCYCLOPROPANE-1-CARBOXYLIC ACID SYNTHASE 1 (MaACS1), thereby inhibiting ethylene biosynthesis and delaying fruit ripening (Hu et al. 2017). In addition, 10−4 M and 5×10−4 M of melatonin were observed to delay the process of ripening and softening in mango (Mangifera indica L.) fruits by inhibiting ethylene production as well as suppressing ABA accumulation and pectin depolymerization (Liu et al. 2020; Dong et al. 2021). Hence, there is an antagonistic relationship between melatonin and ethylene in most climacteric fruits during postharvest that involves changes in the expression of ethylene biosynthesis-related genes (Table 1).
Several factors determine the outcome of the exogenous application of melatonin. First, the method of application, for instance, by spraying, dipping or infiltration, influences the absorption of the molecule into the fruit, while the use of surfactants can also play a role, thereby determining treatment effectiveness. Indeed, all examples found in literature for melatonin involvement on fruit ripening and quality (Table 1) have overlooked root application, even if this method would be the easiest to implement in fields for biostimulant purposes. While some examples can be found for melatonin irrigation to evaluate plant performance in horticultural crops (Supplemental Table S1), to our knowledge, only one study evaluated the effects of melatonin by root application versus leaf spraying in tomato seedlings (Yang et al. 2021), but tomato quality or its implications in fruit formation were not investigated. A second factor affecting melatonin impact would be whether applications were performed during fruit pre- or postharvest, which can have a differential influence, since the sink strength exerted by cytokinin in attached fruits can substantially alter the effects of exogenous melatonin. Third, the differences in physiology between climacteric and nonclimacteric fruits, simple and aggregated fruits, or even true and false fruits according to their origin and constitution might explain some observed disparities. The melatonin dose applied is also an essential factor, since different doses can produce opposite phenotypes, as seen in strawberries (Mansouri et al. 2021). It must be considered that exogenous melatonin applications may lead to a huge increase in its endogenous concentration, generating nonphysiological endogenous levels (Sun et al. 2015; Liu et al. 2018a; Wang et al. 2021b). In that manner, high doses may contribute to creating unnatural physiological conditions, resulting in unexpected outcomes. Moreover, another key point in the exogenous application of melatonin is the moment of application, in other words, at which fruit developmental stage the application is performed. The physiological and biochemical events taking place in fruits differ depending on the stage of development (Kapoor et al. 2022) and elicit different responses. Since the transfer of knowledge to the field of biotechnology is one of the main objectives of research on biostimulants, the spray mode of application in preharvest crops and/or dipping in postharvest fruits are the most effective and easiest options to implement in the field. Nevertheless, several factors influence the outcome and much more research is needed to better understand the factors influencing the final effect of melatonin applied exogenously.
Hence, studies performing exogenous applications of biomolecules can improve knowledge on the role of regulatory molecules and their capability to stimulate or inhibit metabolic pathways. Comprehending the biostimulant function of a molecule is of great interest for its application in agrifood biotechnology. Moreover, assessing the effects of exogenous applications is a simple, economical, and fast technique to gain a better understanding of the physiological involvement of the molecule under study. Nevertheless, some limitations must be contemplated and multiple studies are required to define the optimal dose, mode, and moment of application for each fruit variety. Finally, it is also essential to unravel the mechanisms and modes of action of these regulatory molecules because the knowledge of their role will undoubtedly make exogenous applications more efficient and provide a better prediction of the desired effects.
Correlative analyses and causation
Fruits show different physiological characteristics between the beginning of development, when growth occurs through cell expansion and division, and the beginning of ripening, when seed maturation is complete and ripening occurs, arresting growth and triggering different processes to generate the final quality attributes. Fruit ripening is tightly controlled by multiple regulatory biomolecules that are involved in the up- or downregulation of metabolic pathways, inducing or repressing the transcription of different genes (Mariotti et al. 2011; Kim et al. 2019). For that reason, the endogenous contents of regulatory molecules differ throughout the different stages of fruit development. Thus, analyzing their endogenous concentrations at different points of growth, ripening, and postharvest periods is of great relevance in establishing causation about their biological role.
Melatonin concentration has been quantified in several species and fruit varieties by different analytical techniques (Table 2). In fruits, the presence of melatonin has been described in a wide variety of plant families, indicating that it is ubiquitous at a concentration of ng/g (Nawaz et al. 2016). However, its endogenous concentration differs across species and varieties, even between particular tissues of the same fruit. Therefore, specific tissue analysis is necessary to deepen our understanding of its role, which is conditioned by its localization and the specific metabolic processes taking place there. For example, in climacteric fruits such as Golden Delicious apples, the contents of endogenous melatonin differ between the skin and the pulp, being up to 60 times higher in the former than in the latter. In fact, marked spatiotemporal variations in melatonin contents occur in these fruits; melatonin levels in the skin are very low during the first stages of apple growth, but increase abruptly before the onset of ripening before decreasing constantly until the end of the process (Verde et al. 2022). In suppressed climacteric fruits such as Angeleno plums, higher melatonin contents in the skin than in the pulp have also been reported (Arabia et al. 2022). This higher content in the outer layer of the fruit, which is in contact with the environment, could be related to a protective role. Indeed, melatonin has been described to be involved in stomatal closure to cope with abiotic stress (Li et al. 2015, 2022; Wei et al. 2018). Therefore, its higher levels in the exocarp might be a protective mechanism against environmental stress and might be related not only to its role as a regulatory molecule but also to an additional putative antioxidant role.
Fruit . | Developmental stage . | Endogenous concentration (ng/g) . | Analysis method . | References . |
---|---|---|---|---|
Apple | Full maturity | 0.04 FW | HPLC–FD | Hattori et al. (1995) |
Full maturity | 0.16 FW | GC–MS | Badria (2002) | |
30 d postanthesis | 0.25 (skin); 0.02 (pulp) FW | HPLC–FD | Verde et al. (2022) | |
100 d postanthesis | 1 (skin); 0.006 (pulp) FW | |||
Onset of ripening | 2 (skin); 0.012 (pulp) FW | |||
Full maturity | 0.5 (skin) 0.012 (pulp) FW | |||
Banana | Full maturity | 0.47 FW | GC–MS | Dubbels et al. (1995) |
Full maturity | 0.66 FW | GC–MS | Badria (2002) | |
Full maturity | 0.01 FW | HPLC–FD | Johns et al. (2013) | |
Full maturity | 0.02 to 0.07a FW | ELISA | Hu et al. (2017) | |
6 d postharvest | 0.035 to 0.34a FW | |||
Cherry | Full maturity | 0.00 to 0.224a FW | HPLC–ESI–MS | González-Gómez et al. (2009) |
Initial green | 10 FW | HPLC–FD | Zhao et al. (2013) | |
Green | 35 FW | |||
Full maturity | 10 FW | |||
Initial green | 25 DW | UHPLC–ESI–MS/MS | Tijero et al. (2019) | |
Full maturity | 10 DW | |||
Full maturity | 1.43 FW | |||
Grape | Green | 2 DW | UHPLC–MS | Xu et al. (2018) |
Onset of ripening | 8 DW | |||
Full maturity | 1 DW | |||
Full maturity | 1.2 to 1.5a FW | HPLC–FD | Mercolini et al. (2012) | |
Full maturity | 0.005 to 0.965a (skin, FW) | ELISA | Iriti et al. (2006) | |
Kiwi fruit | Full maturity | 0.24 FW | HPLC–FD | Hattori et al. (1995) |
Mango | Full maturity | 0.7 FW | HPLC–FD | Johns et al. (2013) |
Orange | Full maturity | 0.15 FW | HPLC–FD | Johns et al. (2013) |
Papaya | Full maturity | 0.24 FW | HPLC–FD | Johns et al. (2013) |
Pineapple | Full maturity | 0.04 FW | HPLC–FD | Hattori et al. (1995) |
Full maturity | 0.28 FW | GC–MS | Badria (2002) | |
Full maturity | 0.3 FW | HPLC–FD | Johns et al. (2013) | |
Plum | Full maturity | 20 (skin); 14 (pulp) DW | UHPLC–ESI–MS/MS | Arabia et al. (2022) |
Pomegranate | Full maturity | 0.17 FW | GC-MS | Badria (2002) |
Strawberry (Fragaria magna) | Full maturity | 0.01 FW | HPLC–FD | Hattori et al. (1995) |
Full maturity | 0.14 FW | GC–MS | Badria (2002) | |
Strawberry (Fragaria × ananassa) | Full maturity | 1.4 to 11.26a FW | LC–MS | Stürtz et al. (2011) |
Light green | 7 FW | HPLC–FD | Mansouri et al. (2021) | |
Initial red | 27 FW | |||
Full maturity | 20 FW | |||
Mulberry | Fruit set | 5.76 to 10.34a | HPLC–ESI–MS/MS | Wang et al. (2016a) |
Full maturity | 0.58 to 1.41a | |||
Tomato | Full maturity | 0.11 to 0.51a FW | GC–MS | Dubbels et al. (1995) |
Full maturity | 0.3 FW | GC–MS | Badria (2002) | |
Immature green | 1.5 FW | ELISA | Okazaki and Ezura (2009) | |
Full maturity | 2.7 FW | ELISA | Okazaki and Ezura (2009) | |
Full maturity | 4.1 to 114.5a FW | LC–MS | Stürtz et al. (2011) |
Fruit . | Developmental stage . | Endogenous concentration (ng/g) . | Analysis method . | References . |
---|---|---|---|---|
Apple | Full maturity | 0.04 FW | HPLC–FD | Hattori et al. (1995) |
Full maturity | 0.16 FW | GC–MS | Badria (2002) | |
30 d postanthesis | 0.25 (skin); 0.02 (pulp) FW | HPLC–FD | Verde et al. (2022) | |
100 d postanthesis | 1 (skin); 0.006 (pulp) FW | |||
Onset of ripening | 2 (skin); 0.012 (pulp) FW | |||
Full maturity | 0.5 (skin) 0.012 (pulp) FW | |||
Banana | Full maturity | 0.47 FW | GC–MS | Dubbels et al. (1995) |
Full maturity | 0.66 FW | GC–MS | Badria (2002) | |
Full maturity | 0.01 FW | HPLC–FD | Johns et al. (2013) | |
Full maturity | 0.02 to 0.07a FW | ELISA | Hu et al. (2017) | |
6 d postharvest | 0.035 to 0.34a FW | |||
Cherry | Full maturity | 0.00 to 0.224a FW | HPLC–ESI–MS | González-Gómez et al. (2009) |
Initial green | 10 FW | HPLC–FD | Zhao et al. (2013) | |
Green | 35 FW | |||
Full maturity | 10 FW | |||
Initial green | 25 DW | UHPLC–ESI–MS/MS | Tijero et al. (2019) | |
Full maturity | 10 DW | |||
Full maturity | 1.43 FW | |||
Grape | Green | 2 DW | UHPLC–MS | Xu et al. (2018) |
Onset of ripening | 8 DW | |||
Full maturity | 1 DW | |||
Full maturity | 1.2 to 1.5a FW | HPLC–FD | Mercolini et al. (2012) | |
Full maturity | 0.005 to 0.965a (skin, FW) | ELISA | Iriti et al. (2006) | |
Kiwi fruit | Full maturity | 0.24 FW | HPLC–FD | Hattori et al. (1995) |
Mango | Full maturity | 0.7 FW | HPLC–FD | Johns et al. (2013) |
Orange | Full maturity | 0.15 FW | HPLC–FD | Johns et al. (2013) |
Papaya | Full maturity | 0.24 FW | HPLC–FD | Johns et al. (2013) |
Pineapple | Full maturity | 0.04 FW | HPLC–FD | Hattori et al. (1995) |
Full maturity | 0.28 FW | GC–MS | Badria (2002) | |
Full maturity | 0.3 FW | HPLC–FD | Johns et al. (2013) | |
Plum | Full maturity | 20 (skin); 14 (pulp) DW | UHPLC–ESI–MS/MS | Arabia et al. (2022) |
Pomegranate | Full maturity | 0.17 FW | GC-MS | Badria (2002) |
Strawberry (Fragaria magna) | Full maturity | 0.01 FW | HPLC–FD | Hattori et al. (1995) |
Full maturity | 0.14 FW | GC–MS | Badria (2002) | |
Strawberry (Fragaria × ananassa) | Full maturity | 1.4 to 11.26a FW | LC–MS | Stürtz et al. (2011) |
Light green | 7 FW | HPLC–FD | Mansouri et al. (2021) | |
Initial red | 27 FW | |||
Full maturity | 20 FW | |||
Mulberry | Fruit set | 5.76 to 10.34a | HPLC–ESI–MS/MS | Wang et al. (2016a) |
Full maturity | 0.58 to 1.41a | |||
Tomato | Full maturity | 0.11 to 0.51a FW | GC–MS | Dubbels et al. (1995) |
Full maturity | 0.3 FW | GC–MS | Badria (2002) | |
Immature green | 1.5 FW | ELISA | Okazaki and Ezura (2009) | |
Full maturity | 2.7 FW | ELISA | Okazaki and Ezura (2009) | |
Full maturity | 4.1 to 114.5a FW | LC–MS | Stürtz et al. (2011) |
Data are shown as the mean concentration expressed in ng/g. FW, fresh weight; DW, dry weight; HPLC, high-performance liquid chromatography; ESI, electrospray; MS, mass spectrometry; FD, fluorescence detector; UHPLC, ultra-high-resolution liquid chromatography; MS/MS, tandem mass spectrometry; GC, gas chromatography; LC, liquid chromatography; ELISA, enzyme-linked immunosorbent assay.
Measures in different varieties.
Fruit . | Developmental stage . | Endogenous concentration (ng/g) . | Analysis method . | References . |
---|---|---|---|---|
Apple | Full maturity | 0.04 FW | HPLC–FD | Hattori et al. (1995) |
Full maturity | 0.16 FW | GC–MS | Badria (2002) | |
30 d postanthesis | 0.25 (skin); 0.02 (pulp) FW | HPLC–FD | Verde et al. (2022) | |
100 d postanthesis | 1 (skin); 0.006 (pulp) FW | |||
Onset of ripening | 2 (skin); 0.012 (pulp) FW | |||
Full maturity | 0.5 (skin) 0.012 (pulp) FW | |||
Banana | Full maturity | 0.47 FW | GC–MS | Dubbels et al. (1995) |
Full maturity | 0.66 FW | GC–MS | Badria (2002) | |
Full maturity | 0.01 FW | HPLC–FD | Johns et al. (2013) | |
Full maturity | 0.02 to 0.07a FW | ELISA | Hu et al. (2017) | |
6 d postharvest | 0.035 to 0.34a FW | |||
Cherry | Full maturity | 0.00 to 0.224a FW | HPLC–ESI–MS | González-Gómez et al. (2009) |
Initial green | 10 FW | HPLC–FD | Zhao et al. (2013) | |
Green | 35 FW | |||
Full maturity | 10 FW | |||
Initial green | 25 DW | UHPLC–ESI–MS/MS | Tijero et al. (2019) | |
Full maturity | 10 DW | |||
Full maturity | 1.43 FW | |||
Grape | Green | 2 DW | UHPLC–MS | Xu et al. (2018) |
Onset of ripening | 8 DW | |||
Full maturity | 1 DW | |||
Full maturity | 1.2 to 1.5a FW | HPLC–FD | Mercolini et al. (2012) | |
Full maturity | 0.005 to 0.965a (skin, FW) | ELISA | Iriti et al. (2006) | |
Kiwi fruit | Full maturity | 0.24 FW | HPLC–FD | Hattori et al. (1995) |
Mango | Full maturity | 0.7 FW | HPLC–FD | Johns et al. (2013) |
Orange | Full maturity | 0.15 FW | HPLC–FD | Johns et al. (2013) |
Papaya | Full maturity | 0.24 FW | HPLC–FD | Johns et al. (2013) |
Pineapple | Full maturity | 0.04 FW | HPLC–FD | Hattori et al. (1995) |
Full maturity | 0.28 FW | GC–MS | Badria (2002) | |
Full maturity | 0.3 FW | HPLC–FD | Johns et al. (2013) | |
Plum | Full maturity | 20 (skin); 14 (pulp) DW | UHPLC–ESI–MS/MS | Arabia et al. (2022) |
Pomegranate | Full maturity | 0.17 FW | GC-MS | Badria (2002) |
Strawberry (Fragaria magna) | Full maturity | 0.01 FW | HPLC–FD | Hattori et al. (1995) |
Full maturity | 0.14 FW | GC–MS | Badria (2002) | |
Strawberry (Fragaria × ananassa) | Full maturity | 1.4 to 11.26a FW | LC–MS | Stürtz et al. (2011) |
Light green | 7 FW | HPLC–FD | Mansouri et al. (2021) | |
Initial red | 27 FW | |||
Full maturity | 20 FW | |||
Mulberry | Fruit set | 5.76 to 10.34a | HPLC–ESI–MS/MS | Wang et al. (2016a) |
Full maturity | 0.58 to 1.41a | |||
Tomato | Full maturity | 0.11 to 0.51a FW | GC–MS | Dubbels et al. (1995) |
Full maturity | 0.3 FW | GC–MS | Badria (2002) | |
Immature green | 1.5 FW | ELISA | Okazaki and Ezura (2009) | |
Full maturity | 2.7 FW | ELISA | Okazaki and Ezura (2009) | |
Full maturity | 4.1 to 114.5a FW | LC–MS | Stürtz et al. (2011) |
Fruit . | Developmental stage . | Endogenous concentration (ng/g) . | Analysis method . | References . |
---|---|---|---|---|
Apple | Full maturity | 0.04 FW | HPLC–FD | Hattori et al. (1995) |
Full maturity | 0.16 FW | GC–MS | Badria (2002) | |
30 d postanthesis | 0.25 (skin); 0.02 (pulp) FW | HPLC–FD | Verde et al. (2022) | |
100 d postanthesis | 1 (skin); 0.006 (pulp) FW | |||
Onset of ripening | 2 (skin); 0.012 (pulp) FW | |||
Full maturity | 0.5 (skin) 0.012 (pulp) FW | |||
Banana | Full maturity | 0.47 FW | GC–MS | Dubbels et al. (1995) |
Full maturity | 0.66 FW | GC–MS | Badria (2002) | |
Full maturity | 0.01 FW | HPLC–FD | Johns et al. (2013) | |
Full maturity | 0.02 to 0.07a FW | ELISA | Hu et al. (2017) | |
6 d postharvest | 0.035 to 0.34a FW | |||
Cherry | Full maturity | 0.00 to 0.224a FW | HPLC–ESI–MS | González-Gómez et al. (2009) |
Initial green | 10 FW | HPLC–FD | Zhao et al. (2013) | |
Green | 35 FW | |||
Full maturity | 10 FW | |||
Initial green | 25 DW | UHPLC–ESI–MS/MS | Tijero et al. (2019) | |
Full maturity | 10 DW | |||
Full maturity | 1.43 FW | |||
Grape | Green | 2 DW | UHPLC–MS | Xu et al. (2018) |
Onset of ripening | 8 DW | |||
Full maturity | 1 DW | |||
Full maturity | 1.2 to 1.5a FW | HPLC–FD | Mercolini et al. (2012) | |
Full maturity | 0.005 to 0.965a (skin, FW) | ELISA | Iriti et al. (2006) | |
Kiwi fruit | Full maturity | 0.24 FW | HPLC–FD | Hattori et al. (1995) |
Mango | Full maturity | 0.7 FW | HPLC–FD | Johns et al. (2013) |
Orange | Full maturity | 0.15 FW | HPLC–FD | Johns et al. (2013) |
Papaya | Full maturity | 0.24 FW | HPLC–FD | Johns et al. (2013) |
Pineapple | Full maturity | 0.04 FW | HPLC–FD | Hattori et al. (1995) |
Full maturity | 0.28 FW | GC–MS | Badria (2002) | |
Full maturity | 0.3 FW | HPLC–FD | Johns et al. (2013) | |
Plum | Full maturity | 20 (skin); 14 (pulp) DW | UHPLC–ESI–MS/MS | Arabia et al. (2022) |
Pomegranate | Full maturity | 0.17 FW | GC-MS | Badria (2002) |
Strawberry (Fragaria magna) | Full maturity | 0.01 FW | HPLC–FD | Hattori et al. (1995) |
Full maturity | 0.14 FW | GC–MS | Badria (2002) | |
Strawberry (Fragaria × ananassa) | Full maturity | 1.4 to 11.26a FW | LC–MS | Stürtz et al. (2011) |
Light green | 7 FW | HPLC–FD | Mansouri et al. (2021) | |
Initial red | 27 FW | |||
Full maturity | 20 FW | |||
Mulberry | Fruit set | 5.76 to 10.34a | HPLC–ESI–MS/MS | Wang et al. (2016a) |
Full maturity | 0.58 to 1.41a | |||
Tomato | Full maturity | 0.11 to 0.51a FW | GC–MS | Dubbels et al. (1995) |
Full maturity | 0.3 FW | GC–MS | Badria (2002) | |
Immature green | 1.5 FW | ELISA | Okazaki and Ezura (2009) | |
Full maturity | 2.7 FW | ELISA | Okazaki and Ezura (2009) | |
Full maturity | 4.1 to 114.5a FW | LC–MS | Stürtz et al. (2011) |
Data are shown as the mean concentration expressed in ng/g. FW, fresh weight; DW, dry weight; HPLC, high-performance liquid chromatography; ESI, electrospray; MS, mass spectrometry; FD, fluorescence detector; UHPLC, ultra-high-resolution liquid chromatography; MS/MS, tandem mass spectrometry; GC, gas chromatography; LC, liquid chromatography; ELISA, enzyme-linked immunosorbent assay.
Measures in different varieties.
Variations in endogenous melatonin contents during fruit development have been also reported in various nonclimacteric fruits. In Moldova grapes, melatonin levels increase rapidly during veraison, reaching a concentration of 8 ng/g DW, before decreasing abruptly to the lowest level of around 1 ng/g DW at the ripe stage (Xu et al. 2018). Indeed, a drastic decrease occurs in the endogenous melatonin content from 25 ng/g DW at the first green stage of sweet cherry development to 10 ng/g DW at the fully ripe stage (Tijero et al. 2019). Likewise, endogenous melatonin levels peak at the green developmental stages of Hongdeng and Rainier cherries, decreasing once ripening starts (Zhao et al. 2013). Moreover, in Sabrina strawberries, melatonin contents sharply increase at the onset of ripening, reaching 27 ng/g FW, before gradually decreasing as the ripening progresses (Mansouri et al. 2021). This pattern of melatonin levels decreasing with fruit ripening has been shown also in black and white mulberries (Morus nigra L. and Morus alba L., respectively, Wang et al. 2016a).
There are several methods to quantify the endogenous levels of molecules in fruits that differ in their sensitivity and specificity. Some of the most widely used methods, from the least to the most sensitive, are enzyme-linked immunosorbent assays (ELISAs), radioimmunoassays (RIAs), high-performance liquid chromatography (HPLC) coupled to an electrochemical detector (ECD) or a fluorescence detector (FD), gas chromatography (GC), and HPLC coupled to mass spectrometry (MS) (Feng et al. 2014). On the one hand, the detection of endogenous levels of molecules depends on intrinsic factors of the sample such as the mass/water/oil ratio of the tissue under study, as well as the factors depending on the chemical properties of the matrix, such as its extraction capacity by organic solvents. Thus, an appropriate choice of the extraction solvent is essential. On the other hand, extrinsic factors such as the sensitivity of the instrument determine the capacity of detection. Immunological techniques such as RIAs and ELISAs are based on the principle of competition. However, several molecules present in fruits may cross-react with the antibodies and enzymes used, causing an overestimation of melatonin levels (Johns et al. 2013). In fact, van Tassel et al. (2001) showed that the melatonin concentration obtained with RIAs was overestimated when compared to that obtained with GC–MS due to the relatively high levels of compounds that cross-reacted with the antibody used in the RIAs. Although useful for its relatively low cost, ELISA techniques with low specificity or low sensitivity can lead to incorrect results that over- or underestimate the levels of the molecules of interest. Consequently, chromatographic methods have been the most widely used for melatonin detection in recent years. Although GC–MS stands out for its high sensitivity, specificity, and reproducibility, HPLC does not require previous derivatization or high temperatures and is also a powerful and precise technique (Chafi and Ballesteros 2022). However, it is important to consider its limitations such as interferences due to matrix complexity as well as the huge amount of organic solvent needed. Huang and Mazza (2011) reported that HPLC–MS presented the lowest limits of melatonin detection, being more sensitive than HPLC–FD, HPLC–ECD, and HPLC–UV. Moreover, the multiple reaction monitoring mode allowed the production of multiple specific ion fragments from their specific precursors, providing more sensitivity and specificity (Huang and Mazza 2011). Thus, HPLC–MS is one of the most reliable techniques to quantify melatonin, showing high sensitivity and even more specificity than other techniques.
The study of endogenous levels of melatonin in a spatiotemporal manner, both at different stages and in different tissues, will determine its accumulation pattern, providing essential information for unraveling its regulatory role. However, studies on endogenous melatonin present differences in the analyzed melatonin levels as well as in the units used to express the results. Several studies report the endogenous levels as ng/g of fresh weight, whereas others use g of dry weight or g of tissue. During the analysis of the levels of regulatory molecules at different developmental stages or in tissues, differences between them must be taken into account. For instance, the water content is higher in the flesh tissues or at the fully ripe stage than in the skin of the fruit or at the initial greener stages. Consequently, in physiological terms, by not considering this water status depending on the stage or tissue analyzed, expressing the results as fresh weight may cause an over- or underestimation of the endogenous levels of the regulatory molecules. Therefore, it may be possible that one of the principal factors influencing the high variability in the melatonin levels reported in the literature and even the contradictory results between fruits in various studies is the way the results have been expressed, making comparisons extremely difficult (if not impossible).
A growing number of studies are describing and quantifying the endogenous levels of not only melatonin, but also of other molecules such as the phytohormones and antioxidants involved in the ripening process, as well as the expression of the genes related to their biosynthesis, by performing statistical correlation analyses (Zhao et al. 2013; Tijero et al. 2019; Verde et al. 2019). Establishing a significant positive or negative correlation between melatonin and other compounds or genes using the Pearson or Spearman coefficient could help better comprehend the main metabolic pathways involved. However, it must be considered that a correlation does not imply causation. There may be other factors involved. To confirm this possible interaction, it is necessary to perform in-depth genetic studies that will provide advanced and more specific knowledge from a mechanistic point of view. Indeed, a complex aspect to be additionally considered in the interpretation of variations in the endogenous contents of melatonin is that this compound may both act as a signal molecule and as an antioxidant at the same time. Therefore, assigning specific physiological roles and discussion of possible functions for melatonin and their mode of action based on changes of melatonin contents should be made very carefully, especially if one aims to establish causation.
Overall, despite differences in the analytical methodology used and the units applied to express these results, data from most of the studies suggest that melatonin could have a different regulatory role depending on the stage of development, and there might be differences between climacteric and nonclimacteric fruits (Fig. 2). Melatonin is a natural indoleamine and its biosynthesis involves the chorismate pathway. Chorismate gives rise to tryptophan and after several reactions, it can generate melatonin, but also phytohormones such as the auxin indole-3-acetic acid (IAA). Melatonin and IAA share a common precursor and a very similar chemical structure (Pérez-Llorca et al. 2019). In addition, it has been found that melatonin has auxin-like functions, such as in root growth (Chen et al. 2009; Wen et al. 2016). During fruit development, IAA is principally involved in fruit set and growth, reaching its highest levels during the initial stages of fruit development before decreasing prior to the onset of ripening (Hu et al. 2018). Therefore, we suggest that melatonin may have an auxin-like role during fruit development, promoting growth at the green stages by itself and/or through interactions with auxins, possibly regulating their accumulation in fruits (Fig. 2). Moreover, although auxins have been described to repress ripening, crosstalk between auxins and ethylene has been reported in the ripening of climacteric fruits, with some genes in the ethylene domain regulated by auxins (Trainotti et al. 2007; Zhang et al. 2020; Wang et al. 2021c; Gambhir et al. 2022). Likewise, melatonin can upregulate the expression of genes encoding ethylene biosynthesis enzymes, such as 1-aminocyclopropane-1-carboxylate synthase (ACS) and 1-aminocyclopropane-1-carboxylate oxidase (ACO), as well as influence ethylene signaling (Sun et al. 2015; Verde et al. 2023). In this sense, Shan et al. (2022) showed that melatonin postharvest treatments in green tomato fruits could induce ethylene production by demethylation of CpG islands (DNA regions that contain a high frequency of CpG dinucleotides located around the gene transcription initiation sites) of ethylene biosynthesis (SlACS1) and signal transduction, such as ETHYLENE RESPONSE FACTOR A1 (SlERF-A1) genes, thereby promoting fruit ripening. Likewise, it has been reported that increased production of lycopene and other carotenoids after melatonin treatments in green tomato fruits could be partially explained by increases in the ethylene production mediated by melatonin (Sun et al. 2020). This hypothesis is based on the melatonin effects in both green tomato fruits and never ripe (nr) mutants, insensitive to ethylene signaling (ETHYLENE RECEPTOR 3), where melatonin treatments on nr fail to promote carotenoid biogenesis that can be seen in nonmutagenic tomatoes. Although this work highlights the relationship between melatonin and ethylene, it fails to describe a direct interaction between melatonin and ethylene-related genes. Similarly, Ma et al. (2021) found that exogenous melatonin promoted ethylene biosynthesis in grape berries during on-tree development when treated at green stages and demonstrated that this interaction could be related to VvMYB14 transcription, encoding a transcription factor that binds to the promoter region of VvACS1. Although this study showed that VvACS1 expression was downregulated in VvMYB14 suppressed lines when melatonin was applied, the experiment did not demonstrate a direct interaction between VvMYB14 and melatonin, therefore leaving a gap in the melatonin signal transduction. However, these experiments evidence that in climacteric fruits, melatonin could also act by promoting ethylene biosynthesis through synergistic crosstalk during ripening (Fig. 2). On the contrary, an antagonistic relationship between IAA and ABA has been described during nonclimacteric fruit ripening, with IAA inhibiting the expression of NCED (which encodes 9-cis-epoxycarotenoid dioxygenase that is required for ABA biosynthesis) and PYRABACTIN RESISTANCE 1 (which encodes the receptor for ABA) (Jia et al. 2016). Likewise, melatonin and ABA generally have an antagonistic relationship, with melatonin decreasing ABA levels via the downregulation of ABA biosynthetic genes, the upregulation of its catabolic genes, and the inhibition of its signaling components during stress conditions (Li et al. 2015, 2021; Zhang et al. 2017b; Tan et al. 2019; Wang et al. 2021a). Therefore, in nonclimacteric fruits, it is possible that melatonin might interact with ABA, suppressing its biosynthesis by downregulating the genes associated with ABA synthesis and inhibiting ABA signaling at the onset of ripening.
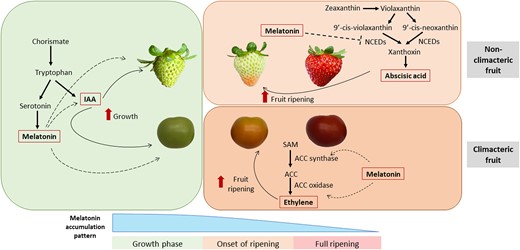
Proposed model of melatonin accumulation and its regulatory role in fruit ripening during different developmental stages in climacteric and nonclimacteric fruits. It is suggested that melatonin promotes fruit growth by itself or by a possible crosstalk with IAA in both climacteric and nonclimacteric fruits. In nonclimacteric fruits, melatonin may act in the advanced developmental stages, interacting antagonistically with ABA through the downregulation of its biosynthesis-related genes such as NCED. By contrast, melatonin might have a positive crosstalk with ethylene, positively influencing its biosynthesis and signaling in climacteric fruits. ACC, 1-aminocyclopropane-1-carboxylic acid; IAA, indole-3-acetic acid; NCED, 9-cis-epoxycarotenoid dioxygenase; SAM, S-adenosyl-methionine.
In summary, measuring endogenous melatonin concentrations can potentially establish, in a spatiotemporal manner, its stimulatory or inhibitory role (reflected by an increase or reduction in its levels, respectively) in the regulation of fruit ripening. To date, some scientific evidence has been obtained not only on the role of melatonin in the modulation of ripening, but also on the metabolic pathways and other molecules involved in the regulation of this process by melatonin. However, as discussed before, some critical factors must be considered to correctly establish causation and avoid quantification mistakes that may sometimes lead to misleading conclusions. Furthermore, it is still not fully clear whether melatonin acts as an antioxidant or signal molecule, more specifically in those cases where endogenous melatonin tissue concentrations are high. It is essential that changing patterns of melatonin are consistent with that of ripening-related indexes to establish causation effects, and this is not clearly observed in all cases, thus suggesting that melatonin might not be a key regulator in fruit ripening, at least not in all fruits.
Evidence in mutant and transgenic lines
Genetics and genomics have revolutionized our knowledge about fruit ripening, being used in studies that have provided important information about the function of genes and metabolites involved in fruit ripening (Giovanoni et al. 2017). There is a plethora of techniques used to obtain transgenic organisms for research purposes in order to identify key regulatory signals or enzymes involved in a given process. One example is RNA interference (RNAi), which consists of downregulating the expression of the gene of interest to study its function and regulation (Baulcombe 2004). There are many methods for transferring dsRNA into plant cells to trigger RNAi pathways, for instance, by developing specific vectors for Agrobacterium tumefaciens transformation and infiltration into plant cells or by infecting plants with autonomous viral vectors (Lin et al. 2013). To better understand the function of a target gene and possible competition with other pathways, it is important not only to study the effects of its silencing, but also those of its overexpression. Overexpression can be achieved through the amplification and cloning of the coding region of the gene of interest into a binary vector with restriction enzymes and using this vector to transform A. tumefaciens (Zhou et al. 2022). Virus-induced gene silencing is also a fast and efficient method for fruit transformation, most specifically for fruit crops that are recalcitrant to Agrobacterium-mediated transformation or for fruit functional studies when gene silencing could induce abnormal plant development (Senthil-Kumar et al. 2011a). Indeed, transgenic lines overexpressing or silencing the gene of interest should be compared to unravel and verify its molecular functions. However, there are some limitations to these methods such as incomplete and temporary knockdowns or nonspecific insertions, leading to ambiguous results and unpredictable effects (Senthil-Kumar et al. 2011b; Casacuberta et al. 2015).
By contrast, specific target mutagenesis is a more efficient method for gene disruption, using engineered nucleases to create a double-strand break that is repaired by homologous or nonhomologous recombination (Wang et al. 2018). Precise genome editing systems using zinc-finger nucleases, transcription activator-like effector nucleases or clustered regularly interspaced short palindromic repeats (CRISPR)-associated protein 9 (Cas9) are promising tools that reduce long cycles to obtain transgenic offspring with homozygosity and avoid nonspecific mutagenesis. However, there are some limitations when applied to fruit studies. Many fruit crops have heterozygous or even polyploid genomes, hybrid incompatibility, and long growth cycles, particularly fruit trees, where initial fruit production can take many years, thus complicating directed mutagenesis (Wang et al. 2018). The CRISPR/Cas9 system uses a guiding RNA molecule to target and cleave specific DNA regions in complex genomes. This system stands out as the most efficient for fruit mutagenesis studies as it is simple and efficient and is not time-consuming (Zhang et al. 2017a), even though its use in fruits is still far from being optimized. For instance, stable transformations in strawberries (Fragaria × ananassa) are complex due to their octoploid genome and their high level of heterozygosity. Indeed, transforming methods must have a higher percentage of effectiveness to obtain satisfactory results in polyploid crops. In these cases, transient expression studies using the agroinfiltration of a CRISPR/cas9 cassette are satisfactory alternatives (Martín-Pizarro et al. 2019). Therefore, the different advantages and limitations of different powerful techniques as well as the added complexity of fruit genomes must be considered in each case when elucidating the function of a particular gene (Fig. 3).
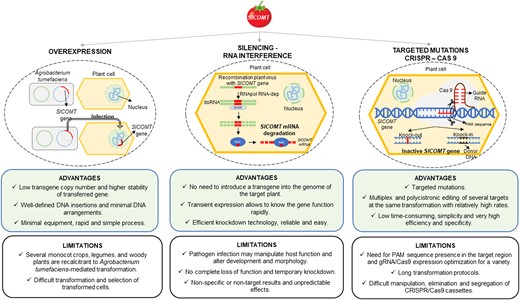
Schematic diagram showing the three techniques used to obtain a mutant tomato line, using the SlCOMT gene (caffeic acid O-methyltransferase) as an example. Gene overexpression using A. tumefaciens transformation (left), gene silencing with RNA interference (center), and targeted mutations with the CRISPR/Cas9 cassette (right) are shown. The advantages and limitations are discussed for each technique.
In plants, melatonin is synthesized by six enzymes: tryptophan decarboxylase (TDC), tryptophan hydroxylase, tryptamine 5-hydroxylase, serotonin N-acetyltransferase (SNAT), N-acetylserotonin O-methyltransferase (ASMT), and caffeic acid O-methyltransferase (COMT). This synthesis includes four reactions that start with tryptophan, with serotonin an essential intermediary (Back et al. 2016). Thus, downregulation or overexpression of some of these genes in fruits, especially the most specific ones involved in the last steps of melatonin biosynthesis (SNAT, ASMT, and COMT), can be used to clarify melatonin function. Studies of the genes associated with melatonin biosynthesis using genetic transformations have mainly focused on their role in abiotic stress resistance, such as mitigating toxicity (Li et al. 2016; Yan et al. 2019), cold-induced oxidative stress (Wang et al. 2020a), and heat stress in tomato plants (Ahammed et al. 2019), by silencing COMT or TDC with Agrobacterium transformation and infiltration. However, none of these studies have evaluated fruit ripening or the regulation of quality attributes to identify the role of melatonin in fruit development. In fact, most gene editing studies in fruit crops have analyzed the expression and regulation of the genes associated with melatonin biosynthesis in vegetative organs such as leaves. Thus, there is a great lack of studies using gene-editing techniques to study melatonin in fruits (Table 3). It was previously shown that the ectopic expression of the ovine homologs of SNAT (oAANAT, from arylalkylamine N-acetyltransferase that is equivalent to the SNAT gene) and ASMT (oHIOMT, from hydroxyindole-O-methyltransferase) in Micro-Tom tomato plants, involving Agrobacterium-mediated transformation, caused a higher accumulation of melatonin in fruits and reduced total plant IAA levels, possibly due to competition for the precursor substrates (Wang et al. 2014). That study did not report reductions in fruit set, deformities in the fruit morphology or parthenocarpy, which would have been promoted by reductions in IAA levels as IAA plays a role in fruit architecture and seed formation (Wang et al. 2005; Batista-Silva et al. 2022). However, it did report that the ectopic expression of oAANAT and oHIOMT induced a severe loss of apical dominance in plant growth by reducing IAA levels in the stems and leaves. Thus, the increased melatonin levels in these plants could not compensate for the IAA deficiency and throws into question the auxin-like function attributed to melatonin, at least for growth. Liu et al. (2019a) also examined the overexpression of SlCOMT1, which is associated with melatonin biosynthesis, in tomato plants to evaluate its role in salt stress resistance. They showed that this gene was highly expressed in fruits at the ripe stages, suggesting its involvement in fruit development. However, the study did not measure fruit production, fruit size or biomass, the ripening dynamics related to color acquisition, starch content, or any basic fruit quality parameter such as sugar content and fruit firmness. In another study, rootstock transformation of tomato plants overexpressing SlCOMT1 increased the total melatonin content in tomato fruits without increasing the expression of SlCOMT1 in fruits (Yan et al. 2019). Yet again, no analyses were performed to evaluate fruit growth and ripening, and the only conclusions made were related to pesticide metabolism through GSH conjugation.
Tomato mutant lines, technique used to generate the line, and the main effects of melatonin are shown
Fruit . | Technique . | Main effects . | References . |
---|---|---|---|
Tomato | Transgenic tomato plants overexpressing oAANAT and oHIOMT using A. tumefaciens | ↑Endogenous melatonin ↓Indoleacetic acid Highest expression in leaves than in ripened fruits | Wang et al. (2014) |
Transgenic tomato plants overexpressing SlCOMT1 using A. tumefaciens | Improved defenses against salinity stress Highest expression in fruits | Liu et al. (2019a) | |
Transgenic rootstock: overexpression of COMT1 using A. tumefaciens | ↑Melatonin in fruit ↓Carbendazim residues (↓ROS and MDA) | Yan et al. (2019) |
Fruit . | Technique . | Main effects . | References . |
---|---|---|---|
Tomato | Transgenic tomato plants overexpressing oAANAT and oHIOMT using A. tumefaciens | ↑Endogenous melatonin ↓Indoleacetic acid Highest expression in leaves than in ripened fruits | Wang et al. (2014) |
Transgenic tomato plants overexpressing SlCOMT1 using A. tumefaciens | Improved defenses against salinity stress Highest expression in fruits | Liu et al. (2019a) | |
Transgenic rootstock: overexpression of COMT1 using A. tumefaciens | ↑Melatonin in fruit ↓Carbendazim residues (↓ROS and MDA) | Yan et al. (2019) |
COMT1, caffeic acid O-methyltransferase 1; MDA, malondialdehyde; ROS, reactive oxygen species; oAANAT, arylalkylamine N-acetyltransferase from the pineal gland of Ovis aries (sheep); oHIOMT, hydroxyindole-O-methyltransferase from the pineal gland of Ovis aries (sheep).
Tomato mutant lines, technique used to generate the line, and the main effects of melatonin are shown
Fruit . | Technique . | Main effects . | References . |
---|---|---|---|
Tomato | Transgenic tomato plants overexpressing oAANAT and oHIOMT using A. tumefaciens | ↑Endogenous melatonin ↓Indoleacetic acid Highest expression in leaves than in ripened fruits | Wang et al. (2014) |
Transgenic tomato plants overexpressing SlCOMT1 using A. tumefaciens | Improved defenses against salinity stress Highest expression in fruits | Liu et al. (2019a) | |
Transgenic rootstock: overexpression of COMT1 using A. tumefaciens | ↑Melatonin in fruit ↓Carbendazim residues (↓ROS and MDA) | Yan et al. (2019) |
Fruit . | Technique . | Main effects . | References . |
---|---|---|---|
Tomato | Transgenic tomato plants overexpressing oAANAT and oHIOMT using A. tumefaciens | ↑Endogenous melatonin ↓Indoleacetic acid Highest expression in leaves than in ripened fruits | Wang et al. (2014) |
Transgenic tomato plants overexpressing SlCOMT1 using A. tumefaciens | Improved defenses against salinity stress Highest expression in fruits | Liu et al. (2019a) | |
Transgenic rootstock: overexpression of COMT1 using A. tumefaciens | ↑Melatonin in fruit ↓Carbendazim residues (↓ROS and MDA) | Yan et al. (2019) |
COMT1, caffeic acid O-methyltransferase 1; MDA, malondialdehyde; ROS, reactive oxygen species; oAANAT, arylalkylamine N-acetyltransferase from the pineal gland of Ovis aries (sheep); oHIOMT, hydroxyindole-O-methyltransferase from the pineal gland of Ovis aries (sheep).
Overall, current experimental approaches using gene editing to determine melatonin function have provided very little information about the actual role of melatonin in fruits and whether this molecule is essential enough for fruit growth and ripening to be considered a hormonal regulator. Directed mutagenesis to suppress melatonin expression involving knockout mutants or gene silencing has been mostly performed in Arabidopsis (Arabidopsis thaliana [L.] Heynh.) or rice (Oryza sativa L.) models to determine the effects on stress tolerance [see recent reviews by Back (2021) and Liu et al. (2022a, b)]. These studies indicate that melatonin deficiency strongly influences pathogen sensitivity (Lee et al. 2015) and the hormonal crosstalk with auxins and gibberellins (GAs) in root architecture and flowering, respectively (Lee et al. 2015; Yang et al. 2021). These effects of melatonin could be of particular interest for understanding the regulation of both fruit development and fruit preservation during postharvest. To our knowledge, the only study referring to melatonin deficiency in fruit crops was based on the silencing of COMT1 in tomato, demonstrating that the silencing aggravated heat stress-related symptoms, but not analyzing the implications of this deficiency for fruit development (Ahammed et al. 2019).
Another important aspect is identifying the signaling pathways elicited by both endogenous melatonin and melatonin applied exogenously as a biostimulant in fruits. In this sense, it is known that melatonin can activate the transcription of ripening-related genes in different fruits, as has been observed after the application of exogenous melatonin. Transcriptomic analysis after melatonin application in fruits has demonstrated that it specifically activates the genes related to the antioxidant system (Zhang et al. 2018; Ze et al. 2021). Modulation of gene transcription by melatonin could be exerted through the direct activation of specific promoter regions, such as the ones described for other phytohormones (Leng et al. 2014; Liu et al. 2015), or by specific interactions with other molecules, such as the ones described for ROS or NO signaling (Corpas et al. 2018; Muñoz and Munné-Bosch 2018). For the former, melatonin would need a specific receptor for its signal transduction, while for the latter, melatonin would need to modify the chemical or structural properties of different molecules to elicit a signaling cascade. Although a lot of effort has been made to identify melatonin-specific transmembrane receptors and signal transduction, there is still a lot of missing gaps, particularly in fruit-related studies. Several recent reviews have proposed potential melatonin-signaling pathways (Back 2021; Liu et al. 2022a, 2022b), such as the one where melatonin interacts with the only melatonin transmembrane receptor identified in plants, the putative phytomelatonin receptor (PMTR1/CAND2) leading to downstream signaling via the activation of the Gα subunit of the receptor or via the mitogen-activated protein kinase (MAPK) signaling cascades. The lack of fruit mutants with impaired melatonin biosynthesis as well as the dearth of melatonin-specific receptors in fruits make it very difficult to classify melatonin as an essential hormonal regulator of fruit development (Box 3). It is important to complement fruit studies, both using exogenous applications and studies on variations in endogenous contents, with transgenic approaches to consider melatonin as an essential molecule in the regulation of fruit development. In this sense, new genome editing tools such as CRISPR/Cas9 and the willingness to develop strategic integrative studies on fruit development are essential in answering outstanding questions related to the role of melatonin in fruit development and ripening.
Not all regulatory biomolecules meet the requirements to be considered a phytohormone involved in the regulation of fruit ripening (see Box 3 Figure). Aside from the classic five (auxins, GAs, cytokinins, abscisic acid, and ethylene), salicylic acid and jasmonates fulfil all requirements (despite some additional evidence using mutant and overexpressed lines as well as in different models is required). Furthermore, brassinosteroids and strigolactones also meet all requirements, except transport and universality, respectively. Other regulatory biomolecules, such as melatonin, meet a variable number of requirements, but not all. These gaps underline future lines of research.
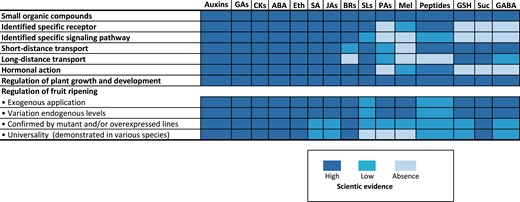
Requirements met by various regulatory biomolecules. ABA, abscisic acid; BRs, brassinosteroids; CKs, cytokinins; Eth, ethylene; GABA, γ-aminobutyric acid; GAs, gibberellins; GSH, glutathione; JAs, jasmonates; Mel, melatonin; PAs, polyamines; Peptides, peptide hormones; SA, salicylic acid; SLs, strigolactones; Suc, sucrose.
Complementary studies integrating knowledge
Experimental design is the basis of producing rigorous data that can elucidate and validate our hypotheses. Studies integrating different experimental approaches can provide relevant information to better understand relationships governing fruit growth, ripening, and postharvest physiological events, which can then improve knowledge for biotechnological and environmentally friendly applications in fruit production. Here, three experimental approaches have been described to illustrate the complexity of characterizing regulatory molecules in fruit ripening, but all are essential and complementary to establish such a role.
Melatonin has been described to be a putative hormone that can regulate fruit quality attributes and fine-tune the ripening process by crosstalk with other hormones. After a thorough analysis of all the studies related to melatonin and fruit ripening using different experimental approaches, it appears that melatonin promotes the initial phases of fruit development (Table 2). This is mainly because endogenous levels in most fruits show a pattern of higher contents at the beginning of fruit development and reductions prior to the onset of ripening, either with a progressive decline during fruit growth or through an accumulative peak before maturation (Fig. 2). This function of melatonin is further validated by the exogenous applications of melatonin. Although there is an important effect of melatonin dosage and the fruit developmental stage, doses of around 10−4 M applied at the beginning of fruit development have been shown to delay the onset of ripening and influence the expression of growth-promoting phytohormones (such as IAA and GAs), while partially repressing the elicitors of fruit ripening (such as ABA and ethylene) (Table 1; Fig. 2). However, it should be noted that molecular analyses in fruits harvested at the early stages of development and detached from plants do not accurately capture the actual functions of the molecule, particularly if the subject of discussion is whether this molecule has a hormonal-like function and, therefore, is transported over short or long distances.
Integrating the findings from the experimental approaches of assessing the effects of exogenous applications and measuring endogenous levels as well as considering the structural nature of melatonin, it appears that melatonin might act as a classical hormone regulating fruit ripening. However, its functions are not different from those of other low-molecular-weight organic molecules that are not considered phytohormones such as GSH and GABA. To truly ascertain whether melatonin acts as a hormonal signal in fruit growth and ripening, it is important to include genetic studies. However, to date, genetic studies on fruit crops have evaluated the effects of melatonin from a stress-related perspective, without considering fruit development and quality parameters. We can speculate from other mutagenesis studies, which have evaluated melatonin function from a mechanistic point of view with growth-promoting phytohormones such as IAA and GAs, that melatonin has a positive relationship with these phytohormones. However, it cannot compensate for their physiological functions when they are deficient, as shown in examples of IAA precursor competition (Wang et al. 2014), IAA mutants (Wang et al. 2016b; Yang et al. 2021), and gibberellin inhibition (Liu et al. 2018b). Melatonin may trigger its function in fruits through IAA-dependent signaling, interacting with other phytohormones, including GAs, ethylene, and ABA. In this model (a), melatonin fine-tunes IAA levels at the initial stages of fruit growth development, integrating circadian or environmental signals that can alter melatonin contents, induce metabolic changes in IAA accumulation, and activate IAA-dependent signaling, while IAA activates nuclear transcriptomic changes to regulate fruit growth architecture and the ripening onset through its interactions with ethylene and ABA (Fig. 4). In a second model (b), melatonin activities are independent of IAA and even if melatonin affects the endogenous contents of this growth-promoting phytohormone, melatonin can directly activate specific promoter regions of genes associated with promoting growth and the ripening-related phytohormones in fruits (Fig. 4), as evidenced by the direct increases in GAs (Zhang et al. 2014) and in particular with ethylene (Sun et al. 2020), either promoting demethylation activity of CpG regions in ethylene-related genes (Shan et al. 2022) or promoting the transcription of specific transcription factors such as those related to the MYB family (Ma et al. 2021). Likewise, because melatonin regulates the redox balance by activating antioxidant systems, a third model (c) considers melatonin an endogenous regulator of ROS levels and, therefore, an activator of signaling cascades, primarily to activate the antioxidant defense system or to elicit controlled ROS-dependent signaling (Fig. 4). This model is supported by work showing that exogenous melatonin triggers transient H2O2 accumulation at the early developmental stages by activating NADPH oxidase (RBOH), which further induces anthocyanin accumulation in pears (Sun et al. 2021a), and also in postharvest models, where melatonin enhances total antioxidant activity to regulate total ROS production and ameliorate fruit decay (Bal 2019; Wang et al. 2019b; Arabia et al. 2022). Furthermore, this model would explain one of the putative melatonin-signaling pathways involving the increased activation of MAPK, which has been long related to the redox status and dependent on H2O2 production control in plants (Kovtun et al. 2000).
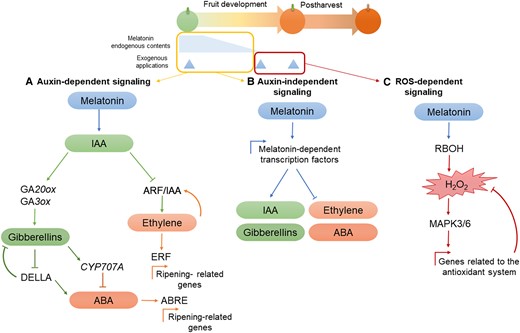
Proposed models for the role of melatonin in fruits. In general, higher melatonin contents are found at the early stages of fruit development. Endogenously, at the early stages, melatonin may interact with other phytohormones to regulate fruit growth and ripening. In the first model A) melatonin, at low concentrations, interacts with auxins (IAA) and elicits IAA-dependent signaling, where high IAA contents promote the activity of gibberellins (GAs), but inhibit both ethylene and ABA. In the second model B), melatonin acts independently of IAA and activates the expression of specific transcription factors that then interact with other promoter regions of important phytohormones in fruit development, promoting IAA and GA accumulation, while inhibiting ethylene and ABA. Lastly, in the model C), exogenous melatonin, especially when applied at the advanced stages of fruit development or during postharvest, elicits ROS-dependent signaling and induces a moderate production of hydrogen peroxide (H2O2) by the activation of NADPH oxidase (RBOH), which in turn triggers the MAPK signaling cascade to enhance the fruit antioxidant machinery. ABRE, ABA-responsive element-binding factors; ARF, auxin response factors; DELLA, negative protein regulators of GAs; ERF, ethylene response factors.
At the moment, models (a) and (c), which are not mutually exclusive, could be considered the most plausible for the regulation of fruit development, but further research is needed because knockout mutants have not been developed, the short- or long-distance transport of melatonin has not been validated, and no promoter regions recognizing melatonin have been identified. Major breakthroughs in understanding the function of melatonin in fruit development would be the identification of a specific receptor of melatonin in fruits and the effects of its downregulation, determining the mechanism of melatonin transport and signal transduction, and identifying specific promoter motifs that recognize melatonin. Undoubtedly, integrative studies that use all experimental approaches would give more information about the role of melatonin, but the limitations of the techniques and budget must be considered. In this sense, cumulative efforts from the scientific community working towards standardized and focused fruit melatonin research would give a complete picture on this topic.
Conclusions and future perspectives
We show here that a combination of the three complementary experimental approaches (exogenous applications, analysis of endogenous contents, and mutagenesis studies) must be considered in elucidating the actual function of active biomolecules, including melatonin in fruit development and ripening. Exogenous applications of melatonin indicate that melatonin could act as a growth promoter and ripening retardant. Due to its high similarity with IAA and given that mutagenesis studies show an interaction between melatonin and IAA, it can be concluded that melatonin acts through auxin-dependent signaling at the early stages of growth. However, the involvement of melatonin in ROS regulation cannot be excluded, especially at the later stages of fruit development or during post-harvest, where it has a biostimulant-like function. At the same time, melatonin cannot be classified as a hormonal regulator of fruit development and ripening until its transport and signaling have been completely elucidated. Altogether, the analysis of this molecule shows the need for integrative studies in fruit development and ripening to discern between phytohormones and other regulatory molecules. Melatonin studies aimed at elucidating the hormonal role of melatonin in fruit development and ripening should include a complete spatiotemporal evaluation of melatonin biosynthesis and signaling, whether the molecule is required at specific phases of fruit development or whether tissular gradients determine its mode of action. Functional studies with mutagenic lines will also validate whether this molecule is essential for fruit development and ripening, including examples in nonmodel species with different patterns of hormonal regulation, where sensitiveness to a lack of melatonin could have particular outcomes. At the same time, in silico studies for the discovery of melatonin ligands at upstream regions of melatonin-differentially expressed genes should be a priority taking advantage of the appropriate bioinformatic analyses and the plethora of RNAseq already available from studies already made that are based on melatonin applications. Ultimately, integrative studies considering melatonin involvement in fruit ripening from a whole plant perspective will undoubtedly help elucidating key open questions (see “Outstanding Questions”) related to the possible hormonal role of this molecule, including root-to-shoot and shoot-to-root translocation and evaluation of plant performance, so that new innovative solutions can be implemented for improving ongoing technological agricultural practices.
Integrative studies including various experimental approaches (based on exogenous applications, analysis of endogenous contents, and mutagenesis) can give us a complete understanding of the function of phytohormones and other regulatory biomolecules in plants.
Melatonin is a hormone-like compound with growth-promoting effects in fruit development.
Melatonin promotes auxin signaling and this model may explain several of the functions of melatonin observed in fruits.
ROS-melatonin interactions may explain its biostimulant function, particularly at the later stages of fruit development and during postharvest.
Will additional studies using fruits with altered melatonin contents, either by TILLING or CRISPR/Cas9 methods, help us clarify the putative hormonal role of melatonin in fruit development and ripening?
Will a melatonin receptor and the underlying signaling components be identified in fruits?
Will long-distance transport of melatonin related to the regulation of fruit development and ripening be identified in the near future?
Does melatonin applied as a biostimulant have contrasting dose–response effects in climacteric and nonclimacteric fruits?
Acknowledgments
We thank Michael Maudsley for English correction of the manuscript.
Supplemental data
The following materials are available in the online version of this article.
Supplemental Table S1. Exogenous treatments of melatonin applied by irrigation in fruity crops.
Funding
The work in SMB laboratory is supported by the Catalan Government through an ICREA Academia award and the 2021 SGR 00675 grant, and by the Spanish Government through the PID2019-110335GB-I00 and CEX2021-001234-M grants.
Data availability
No new datasets were generated or analysed in this study.
References
Author notes
Senior author.
The author responsible for distribution of materials integral to the findings presented in this article in accordance with the policy described in the Instructions for Authors (https://dbpia.nl.go.kr/plphys/pages/general-instructions) is Sergi Munné-Bosch ([email protected]).
Conflict of interest statement. None declared.