-
PDF
- Split View
-
Views
-
Cite
Cite
Yanna Shi, Bai-Jun Li, Donald Grierson, Kun-Song Chen, Insights into cell wall changes during fruit softening from transgenic and naturally occurring mutants, Plant Physiology, Volume 192, Issue 3, July 2023, Pages 1671–1683, https://doi.org/10.1093/plphys/kiad128
- Share Icon Share
Abstract
Excessive softening during fleshy fruit ripening leads to physical damage and infection that reduce quality and cause massive supply chain losses. Changes in cell wall (CW) metabolism, involving loosening and disassembly of the constituent macromolecules, are the main cause of softening. Several genes encoding CW metabolizing enzymes have been targeted for genetic modification to attenuate softening. At least 9 genes encoding CW-modifying proteins have increased expression during ripening. Any alteration of these genes could modify CW structure and properties and contribute to softening, but evidence for their relative importance is sparse. The results of studies with transgenic tomato (Solanum lycopersicum), the model for fleshy fruit ripening, investigations with strawberry (Fragaria spp.) and apple (Malus domestica), and results from naturally occurring textural mutants provide direct evidence of gene function and the contribution of CW biochemical modifications to fruit softening. Here we review the revised CW structure model and biochemical and structural changes in CW components during fruit softening and then focus on and integrate the results of changes in CW characteristics derived from studies on transgenic fruits and mutants. Potential strategies and future research directions to understand and control the rate of fruit softening are also discussed.
Introduction
Fleshy fruits are derived from a variety of flower parts and are important organs for facilitating seed dispersal. During growth and seed development, fruits are unattractive and unpalatable but at maturity they undergo changes in aroma, color, texture, and taste that make them attractive to eat. Softening is an irreversible programmed part of the fleshy fruit ripening process and a soft and juicy texture is the basis of a good eating quality that attracts predators to disperse the seeds (Seymour et al. 2013). However, excessive softening reduces shelf life, increases the likelihood of physical damage and susceptibility to attack by microorganisms, and leads to subsequent food waste and economic losses. Softening, like other aspects of ripening, has been shown to be regulated by endogenous phytohormones, mainly ethylene in climacteric fruits and abscisic acid in nonclimacteric fruits (Li et al. 2021, 2022). A large effort has been expended to manipulate ethylene biosynthesis, perception, or action to prolong fruit storage time (Oeller et al. 1991; Picton et al. 1993; Vrebalov et al. 2002; Giovannoni et al. 2004; Tucker et al. 2017). However, in many fruits ethylene governs most aspects of ripening (color, flavor, aroma, as well as texture), therefore inhibiting its biosynthesis leads to the sacrifice of organoleptic and nutritional characteristics (Oeller et al. 1991; Ayub et al. 1996), as does the use of genes that inhibit ripening, such as rin (ripening inhibitor), in conventional breeding (Vrebalov et al. 2002; Tucker et al. 2017; Li et al. 2020). To resolve this paradox, attention has focused on new approaches to uncoupling softening and ethylene production. Although the degradation of starch in fruits such as banana (Musa acuminata) and kiwifruit (Actinidia deliciosa) may contribute (Shiga et al. 2011), softening is mainly a consequence of changes in primary CW metabolism, and modifying expression of CW metabolism genes seems to be a promising route to manipulate texture. Researchers have attempted to determine the CW biophysical and biochemical changes and the underlying molecular mechanism during softening and modify textural features using biotechnology since the 1990s (reviewed by Fischer and Bennett 1991; Redgwell et al. 1997; Brummell and Harpster 2001; Brummell 2006). More recently, there have been important advances in the discovery and characterization of CW-modifying genes, understanding CW architecture and mechanical properties, and the development of genomic, transcriptomic, and proteomic tools (Tomato Genome Consortium 2012; Posé et al. 2019; Wang et al. 2019). This review focuses on the evidence for the role of specific genes encoding enzymes that participate in CW loosening and disassembly, and their respective contributions to fruit softening.
The emergence of new technologies for CW research has provided substantial insights into how CW enzymes, proteins, and CW dynamics contribute to fruit softening.
Softening is a multigenic trait and can be affected by several genes. Simultaneous suppression of SlPG2a and SlExp1 leads to significantly firmer tomato fruit compared to suppression of either SlPG2a or SlExp1 alone.
Silencing PG has little effect on softening in tomato although pectin metabolism is altered; however, silencing of PG in Rosaceae fruits significantly increases ripe fruit firmness.
Silencing PL in several fruits leads to a pronounced firmer texture. In tomato, suppression of SlPL extends shelf life and reduces susceptibility to gray mold, identifying it as a major contributor to fruit softening and a promising candidate for technological applications.
Structure and organization of cell wall (CW) components
The plant CW is an elaborate extracellular matrix, comprising the primary CW, secondary CW, and middle lamella (ML) between cells. Most fleshy fruits consist mainly of parenchyma cells, which have only a thin primary CW, while few fruits (e.g. pear [Pyrus spp.] and loquat [Eriobotrya Japonica]) contain lignified cells with secondary CWs; thus the secondary CWs are not considered here. The plant’s primary CW is composed of 3 dominant biopolymers, cellulose, hemicellulose (mainly xyloglucan in dicots) and pectin, plus CW proteins (San Clemente et al. 2022; Box 1). Cellulose is a hydrogen-bonded linear chain of β-(1–4)-linked D-glucose assembled into cellulose microfibrils, which are believed to be the main load-bearing polymers. Xyloglucan consists of a cellulose backbone with α-D-xylosyl residues attached to the 6th position and requires strong alkali conditions for solubilization (Schultink et al. 2014). The pectin polysaccharides are hydrated polymers containing galacturonic acid residues, present in the primary CW and ML, comprised mainly of homogalacturonan (HG), the most abundant and best understood pectin type, plus rhamnogalacturonan I (RGI) and rhamnogalacturonan II (RGII). These CW components interact both covalently and noncovalently to form a load-bearing network. CW models have been constructed and revised over recent decades, incorporating advanced spectroscopic approaches and newly gained genetic insights (Cosgrove 2001, 2022; Albersheim et al. 2011; Zhang et al. 2021). Solid-state nuclear magnetic resonance (NMR) results indicate fewer xyloglucan–cellulose interactions than expected and more extensive pectin–cellulose interactions than previously believed (Dick-Pérez et al. 2011; Wang et al. 2015; Wang and Hong 2016). More recently, Park and Cosgrove (2012) proposed the “biomechanical hotspot” model of CW structure, based on studies with xyloglucan-deficient Arabidopsis (Arabidopsis thaliana) and the mechanical effects of endoglucanase digestion. In this revised CW model, cellulose microfibril contacts are mediated by a subfraction of inaccessible xyloglucans, which bind to hydrophobic surfaces at cellulose–cellulose junctions. The space between cellulose layers is occupied by pectins, which make extensive contacts with the hydrophilic surfaces of cellulose (Fig. 1). During growth, changes in CW extensibility involve selective control of slippage and separation of microfibrils at specific and limited sites in the wall (Park and Cosgrove 2012; Cosgrove 2016; Zhang et al. 2021; Cosgrove 2022) and this revised concept of the structure has implications for models of CW softening. The ML is a region of the primary CW, which has been characterized as a zone of intercellular adhesion and separation (Zamil and Geitmann 2017). Tricellular junctions (TCJs) (Fig. 1) are a part of the ML and rich in deesterified HGs. Pectin–pectin interactions generate an egg-box-like pectate gel to strengthen cell-to-cell adhesion, and protein–pectin interactions are detected in the ML, based on immunohistochemical studies using plant CW monoclonal antibodies (Daher and Braybrook 2015; Zamil and Geitmann 2017).
Recently, with the development of CW proteomics, a more comprehensive view of CW-located proteins has been achieved and in Arabidopsis, approximately 1,000 wall proteins have been identified (reviewed by San Clemente et al. 2022). These can be divided into 2 main groups. (i) Proteins acting on CW carbohydrates, including GHs and carbohydrate modifiers. Members of different gene families of wall-associated proteins targeting the 3 main polymers (cellulose, hemicellulose, and pectin) have been widely investigated and discussed in the main text of this review for a potential role in fruit softening. Carbohydrate modifiers consist of PMEs inhibitors, oxidoreductases (e.g. class III peroxidases and laccases, characterized by their function in secondary wall deposition in fruits [Shi et al. 2022], but their role in fruit primary CW stiffening by cross-linking glycoproteins has not been thoroughly investigated). (ii) Glycoproteins include arabinogalactan proteins and extensins. Reports in Arabidopsis have revealed that in addition to wall polysaccharides, glycoproteins can modify the mechanical properties of the CW and play versatile roles in developmental growth, stress-related signal sensing and wall remodeling (Cosgrove 2014; Su and Higashiyama 2018; Herger et al. 2019). There is also evidence suggesting a role for glycoproteins in fruit softening. For example, Meli et al. (2010) silenced 2 CW genes encoding N-glycan processing enzymes (α-Man and β-Hex), which led to a reduced rate of softening and enhanced shelf life, potentially by hydrolyzing the N-glycans of glycoproteins. More attention needs to be paid to characterization of CW proteins, in addition to the more obvious candidates, and investigating their roles in fruit softening.
CW metabolism during fruit softening
Changes in fruit CW metabolism during ripening and storage involve CW loosening, pectin solubilization, pectin and hemicellulose depolymerization, RGI Gal and Ara side chain loss, ML dissolution, cell adhesion reduction, and CW swelling (Crookes and Grierson 1983; Redgwell et al. 1997; Brummell and Harpster 2001). However, the content of cellulose does not decline in most fruits, e.g. in tomato the amount of cellulose remains constant during softening, and only small depolymerization has been observed in the locular gel, but none in pericarp tissue (Maclachlan and Brady 1994). The lack of quantitative changes in cellulose should not be taken to indicate that it has no function in softening, however, since changes in microarchitecture may contribute to CW loosening. Sequential extractions of CW glycans with different solvents have been used to yield different fractions. Water dissolves water-soluble pectins, chelating solution (CDTA or EDTA) extracts pectins held in the wall by ionic bonds, sodium carbonate (Na2CO3) extracts covalently linked pectins, dilute alkali solution (1 M KOH) extracts mainly nonxyloglucan hemicellulose, and concentrated alkali solution (4 M KOH) extracts mainly xyloglucan. These different components have been studied by size-exclusion chromatography and neutral sugar quantification in a range of fruits. Taking tomato fruit as an example, the amount of water-soluble pectins increases while the amount of Na2CO3-soluble pectins declines during ripening (Carrington et al. 1993). This is accompanied by a reduction in the molecular weights of components extracted by chelating solutions and concentrated alkali during ripening, while there is no change to Na2CO3-soluble pectins and dilute alkali extracts (Brummell et al. 1999b). There is a loss of Gal in extracts of Na2CO3-soluble pectins, dilute alkali-soluble, and concentrated alkali-soluble extracts (Seymour et al. 1990; Carrington et al. 1993). However, different fruits or even cultivars display intricate and varied differences in CW biochemical fractions during softening (Redgwell et al. 1997; Brummell 2006; Yoshioka et al. 2011).
How these wall changes during softening relate to one another and contribute to CW structure and fruit softening is still an open question. There are at least 9 types of CW structural or metabolizing proteins that change in amount during fruit ripening and any or all of these could contribute to the overall softening process (Fig. 1; Table 1). Several of the genes encoding one or more of these enzymes have been considered candidates for contributing to softening. The results from genetically modified (GMO) fruits in which genes encoding CW-associated proteins have been down-regulated, or comparisons between WT and naturally occurring mutants, such as melting-flesh and nonmelting-flesh peach (Prunus persica) (lacking endo-PG activity), provide direct evidence for the function of specific genes and the contribution of corresponding CW biochemical modification to fruit softening. Extensive studies on CW gene modification have been carried out on tomato, strawberry, and apple (Brummell and Harpster 2001; Li et al. 2010; Meli et al. 2010; Wang et al. 2018; Shi et al. 2022), using knockdown (antisense/RNAi) and knockout (CRISPR, clustered regularly interspaced short palindromic repeats) approaches. The differences in CW biochemical characteristics of control and GMO fruits and their effects on softening are shown in Table 1 and discussed in the following sections.
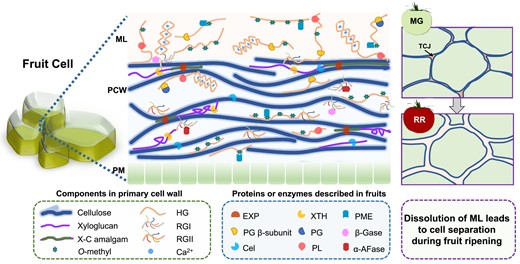
Cartoon of primary cell wall structure and a working model of CW enzymes and proteins implicated in fruit softening. The ML is the layer between primary cell walls (PCW) of neighboring cells, which are external to the plasma membrane (PM). TCJs are a part of the ML, the places where 3 cells meet. One cellulose layer is depicted in this wall model. Xyloglucan binds to hydrophobic surfaces at cellulose–cellulose junctions to form a limited xyloglucan-cellulose (X-C) amalgam called biomechanical hotspots involved in CW loosening (extension/creep) (Park and Cosgrove 2012). EXP is a key protein agent that disrupts the xyloglucan-cellulose amalgam, and causes wall creep, while endo-1,4-β-glucanase (cellulase, Cel) and XTH may also act as wall loosening agents (Cosgrove 2016). Pectins, including HG, RGI, and RGII make extensive contacts with cellulose, and occupy the space between cellulose layers. HG is the most abundant pectin, particularly in the ML, and is deesterified by PME in blockwise or random demethylation patterns. Blockwise deesterified HG forms cross-links with Ca2+ to form an egg-box like pectate gel (shown in the ML and PCW), while random deesterified HG is degraded via endo-PG and PL, both actions have been detected during fruit ripening and postharvest storage. PL has a particular action on pectin in the TCJs, shown in pink in the MG fruit cartoon (top right). The PG β-subunit interacts with and inhibits the activity of PG. RGI and RGII are absent from the ML region, and exo-galactanase/β-galactosidase (β-Gase) and α-L-arabinofuranosidase (α-AFase) cleave the galactosyl and arabinayl residues, respectively, on the side chain of RGI. The ML dissolves due to degradation of HG pectin during fruit softening and the transition from mature green (MG) to red ripe (RR) tomato which causes the loosening of cell adhesion and leads to cell separation.
CW biochemical analysis of stably GMO fruit (genes encoding softening-associated CW-modifying enzymes were used for transformation
Fruits . | Gene . | Strategy . | Cell separation . | Pectin solubilization . | Pectin depolymerization . | Hemicellulose depolymerization . | Firmness . | Shelf life . | Reference . |
---|---|---|---|---|---|---|---|---|---|
Tomato | SlEXP1 | Antisense | / | / | Reduced (CDTA-fraction) | Unaffected (24% KOH-fraction) | Enhanced (13%, BR) | Extended | Brummell et al. (1999b), Powell et al. (2003) |
SlEXP1 | OE | / | / | Unaffected (CDTA-fraction) | Increased (24% KOH-fraction) | Reduced (∼30%, MG) | / | Brummell et al. (1999b), Powell et al. (2003) | |
SlPG2a | Antisense & CRISPR | Reduced | Reduced (water-soluble, in paste) | Reduced (CDTA-fraction; LM19 to deesterified HG) | / | Unaffected | Extended | Schuch et al. (1991), Brummell and Labavitch (1997), Wang et al. (2019) | |
SlPG2a | OE in rin mutant | / | Increased (EDTA-soluble) | Unaffected (chelating-fraction) | / | Unaffected | / | Giovannoni et al. (1989), Della Penna et al. (1990) | |
Slβ-subunit | Antisense | / | Increased (EDTA-soluble) | Increased (EDTA-fraction) | / | Reduced (∼50%, ripe) | / | Watson et al. (1994), Chun and Huber (2000) | |
SLPG&SlEXP1 | Antisense | / | Reduced (water-soluble) | Reduced (all pectin fractions) | Unaffected | Enhanced (>20%, B7) | Powell et al. (2003), Cantu et al. (2008) | ||
SlPL | RNAi & CRISPR | Reduced | Reduced (water-soluble) | Reduced (LM 19 to deesterified HG; INRA-RU1 to RGI) | / | Enhanced (>100%, B7) | Extended | Uluisik et al. (2016), Yang et al. (2017) | |
SlTBG4 | CRISPR | Increased | Increased (water-soluble) | / | / | Unaffected | / | Wang et al. (2019) | |
SlPME2 | Antisense | / | Reduced (EDTA-soluble) | Reduced (EDTA-fraction) | / | Enhanced (∼15%, red ripe) | Shorten | Tieman et al. (1992), Tieman and Handa (1994) | |
SlXTH5 | CRISPR | / | / | / | Reduced (LM25 to xyloglucan) | Enhanced (∼5% to 30%, B7) | / | Wang et al. (2022) | |
SlXTH1 | OE | / | / | / | Reduced (24% KOH-fraction) | Enhanced (∼25%, pink) | / | Miedes et al. (2010) | |
Pepper | CaCel1 | Antisense | / | / | Unaffected (both CDTA and Na2CO3-fraction) | Unaffected (8 M KOH-fraction) | / | / | Harpster et al. (2002a) |
Apple | MdPG1 | Antisense | Reduced | Reduced (water-soluble) | Reduced (CDTA-fraction, Jim5 to deesterified HG) | / | Enhanced (∼50% to 75%, ripe) | Extended | Atkinson et al. (2012) |
Strawberry | FaPG1 | Antisense | Reduced | Reduced | Reduced (both CDTA and Na2CO3-fraction; Jim5 to deesterified HG) | Unaffected | Enhanced (∼60%, ripe) | / | Posé et al. (2013) |
FaPL | Antisense | Reduced | Reduced (water-soluble) | Reduced (both CDTA and Na2CO3-fraction) | Reduced (4 M KOH-fraction) | Enhanced (∼40%, ripe) | / | Santiago-Doménech et al. (2008) | |
FvPLA | RNAi | Reduced | / | Reduced (Jim5 to deesterified HG) | / | Enhanced (∼50%, 20 dpa) | / | Zhang et al. (2022) |
Fruits . | Gene . | Strategy . | Cell separation . | Pectin solubilization . | Pectin depolymerization . | Hemicellulose depolymerization . | Firmness . | Shelf life . | Reference . |
---|---|---|---|---|---|---|---|---|---|
Tomato | SlEXP1 | Antisense | / | / | Reduced (CDTA-fraction) | Unaffected (24% KOH-fraction) | Enhanced (13%, BR) | Extended | Brummell et al. (1999b), Powell et al. (2003) |
SlEXP1 | OE | / | / | Unaffected (CDTA-fraction) | Increased (24% KOH-fraction) | Reduced (∼30%, MG) | / | Brummell et al. (1999b), Powell et al. (2003) | |
SlPG2a | Antisense & CRISPR | Reduced | Reduced (water-soluble, in paste) | Reduced (CDTA-fraction; LM19 to deesterified HG) | / | Unaffected | Extended | Schuch et al. (1991), Brummell and Labavitch (1997), Wang et al. (2019) | |
SlPG2a | OE in rin mutant | / | Increased (EDTA-soluble) | Unaffected (chelating-fraction) | / | Unaffected | / | Giovannoni et al. (1989), Della Penna et al. (1990) | |
Slβ-subunit | Antisense | / | Increased (EDTA-soluble) | Increased (EDTA-fraction) | / | Reduced (∼50%, ripe) | / | Watson et al. (1994), Chun and Huber (2000) | |
SLPG&SlEXP1 | Antisense | / | Reduced (water-soluble) | Reduced (all pectin fractions) | Unaffected | Enhanced (>20%, B7) | Powell et al. (2003), Cantu et al. (2008) | ||
SlPL | RNAi & CRISPR | Reduced | Reduced (water-soluble) | Reduced (LM 19 to deesterified HG; INRA-RU1 to RGI) | / | Enhanced (>100%, B7) | Extended | Uluisik et al. (2016), Yang et al. (2017) | |
SlTBG4 | CRISPR | Increased | Increased (water-soluble) | / | / | Unaffected | / | Wang et al. (2019) | |
SlPME2 | Antisense | / | Reduced (EDTA-soluble) | Reduced (EDTA-fraction) | / | Enhanced (∼15%, red ripe) | Shorten | Tieman et al. (1992), Tieman and Handa (1994) | |
SlXTH5 | CRISPR | / | / | / | Reduced (LM25 to xyloglucan) | Enhanced (∼5% to 30%, B7) | / | Wang et al. (2022) | |
SlXTH1 | OE | / | / | / | Reduced (24% KOH-fraction) | Enhanced (∼25%, pink) | / | Miedes et al. (2010) | |
Pepper | CaCel1 | Antisense | / | / | Unaffected (both CDTA and Na2CO3-fraction) | Unaffected (8 M KOH-fraction) | / | / | Harpster et al. (2002a) |
Apple | MdPG1 | Antisense | Reduced | Reduced (water-soluble) | Reduced (CDTA-fraction, Jim5 to deesterified HG) | / | Enhanced (∼50% to 75%, ripe) | Extended | Atkinson et al. (2012) |
Strawberry | FaPG1 | Antisense | Reduced | Reduced | Reduced (both CDTA and Na2CO3-fraction; Jim5 to deesterified HG) | Unaffected | Enhanced (∼60%, ripe) | / | Posé et al. (2013) |
FaPL | Antisense | Reduced | Reduced (water-soluble) | Reduced (both CDTA and Na2CO3-fraction) | Reduced (4 M KOH-fraction) | Enhanced (∼40%, ripe) | / | Santiago-Doménech et al. (2008) | |
FvPLA | RNAi | Reduced | / | Reduced (Jim5 to deesterified HG) | / | Enhanced (∼50%, 20 dpa) | / | Zhang et al. (2022) |
/ Indicates that the information is missing.
CW biochemical analysis of stably GMO fruit (genes encoding softening-associated CW-modifying enzymes were used for transformation
Fruits . | Gene . | Strategy . | Cell separation . | Pectin solubilization . | Pectin depolymerization . | Hemicellulose depolymerization . | Firmness . | Shelf life . | Reference . |
---|---|---|---|---|---|---|---|---|---|
Tomato | SlEXP1 | Antisense | / | / | Reduced (CDTA-fraction) | Unaffected (24% KOH-fraction) | Enhanced (13%, BR) | Extended | Brummell et al. (1999b), Powell et al. (2003) |
SlEXP1 | OE | / | / | Unaffected (CDTA-fraction) | Increased (24% KOH-fraction) | Reduced (∼30%, MG) | / | Brummell et al. (1999b), Powell et al. (2003) | |
SlPG2a | Antisense & CRISPR | Reduced | Reduced (water-soluble, in paste) | Reduced (CDTA-fraction; LM19 to deesterified HG) | / | Unaffected | Extended | Schuch et al. (1991), Brummell and Labavitch (1997), Wang et al. (2019) | |
SlPG2a | OE in rin mutant | / | Increased (EDTA-soluble) | Unaffected (chelating-fraction) | / | Unaffected | / | Giovannoni et al. (1989), Della Penna et al. (1990) | |
Slβ-subunit | Antisense | / | Increased (EDTA-soluble) | Increased (EDTA-fraction) | / | Reduced (∼50%, ripe) | / | Watson et al. (1994), Chun and Huber (2000) | |
SLPG&SlEXP1 | Antisense | / | Reduced (water-soluble) | Reduced (all pectin fractions) | Unaffected | Enhanced (>20%, B7) | Powell et al. (2003), Cantu et al. (2008) | ||
SlPL | RNAi & CRISPR | Reduced | Reduced (water-soluble) | Reduced (LM 19 to deesterified HG; INRA-RU1 to RGI) | / | Enhanced (>100%, B7) | Extended | Uluisik et al. (2016), Yang et al. (2017) | |
SlTBG4 | CRISPR | Increased | Increased (water-soluble) | / | / | Unaffected | / | Wang et al. (2019) | |
SlPME2 | Antisense | / | Reduced (EDTA-soluble) | Reduced (EDTA-fraction) | / | Enhanced (∼15%, red ripe) | Shorten | Tieman et al. (1992), Tieman and Handa (1994) | |
SlXTH5 | CRISPR | / | / | / | Reduced (LM25 to xyloglucan) | Enhanced (∼5% to 30%, B7) | / | Wang et al. (2022) | |
SlXTH1 | OE | / | / | / | Reduced (24% KOH-fraction) | Enhanced (∼25%, pink) | / | Miedes et al. (2010) | |
Pepper | CaCel1 | Antisense | / | / | Unaffected (both CDTA and Na2CO3-fraction) | Unaffected (8 M KOH-fraction) | / | / | Harpster et al. (2002a) |
Apple | MdPG1 | Antisense | Reduced | Reduced (water-soluble) | Reduced (CDTA-fraction, Jim5 to deesterified HG) | / | Enhanced (∼50% to 75%, ripe) | Extended | Atkinson et al. (2012) |
Strawberry | FaPG1 | Antisense | Reduced | Reduced | Reduced (both CDTA and Na2CO3-fraction; Jim5 to deesterified HG) | Unaffected | Enhanced (∼60%, ripe) | / | Posé et al. (2013) |
FaPL | Antisense | Reduced | Reduced (water-soluble) | Reduced (both CDTA and Na2CO3-fraction) | Reduced (4 M KOH-fraction) | Enhanced (∼40%, ripe) | / | Santiago-Doménech et al. (2008) | |
FvPLA | RNAi | Reduced | / | Reduced (Jim5 to deesterified HG) | / | Enhanced (∼50%, 20 dpa) | / | Zhang et al. (2022) |
Fruits . | Gene . | Strategy . | Cell separation . | Pectin solubilization . | Pectin depolymerization . | Hemicellulose depolymerization . | Firmness . | Shelf life . | Reference . |
---|---|---|---|---|---|---|---|---|---|
Tomato | SlEXP1 | Antisense | / | / | Reduced (CDTA-fraction) | Unaffected (24% KOH-fraction) | Enhanced (13%, BR) | Extended | Brummell et al. (1999b), Powell et al. (2003) |
SlEXP1 | OE | / | / | Unaffected (CDTA-fraction) | Increased (24% KOH-fraction) | Reduced (∼30%, MG) | / | Brummell et al. (1999b), Powell et al. (2003) | |
SlPG2a | Antisense & CRISPR | Reduced | Reduced (water-soluble, in paste) | Reduced (CDTA-fraction; LM19 to deesterified HG) | / | Unaffected | Extended | Schuch et al. (1991), Brummell and Labavitch (1997), Wang et al. (2019) | |
SlPG2a | OE in rin mutant | / | Increased (EDTA-soluble) | Unaffected (chelating-fraction) | / | Unaffected | / | Giovannoni et al. (1989), Della Penna et al. (1990) | |
Slβ-subunit | Antisense | / | Increased (EDTA-soluble) | Increased (EDTA-fraction) | / | Reduced (∼50%, ripe) | / | Watson et al. (1994), Chun and Huber (2000) | |
SLPG&SlEXP1 | Antisense | / | Reduced (water-soluble) | Reduced (all pectin fractions) | Unaffected | Enhanced (>20%, B7) | Powell et al. (2003), Cantu et al. (2008) | ||
SlPL | RNAi & CRISPR | Reduced | Reduced (water-soluble) | Reduced (LM 19 to deesterified HG; INRA-RU1 to RGI) | / | Enhanced (>100%, B7) | Extended | Uluisik et al. (2016), Yang et al. (2017) | |
SlTBG4 | CRISPR | Increased | Increased (water-soluble) | / | / | Unaffected | / | Wang et al. (2019) | |
SlPME2 | Antisense | / | Reduced (EDTA-soluble) | Reduced (EDTA-fraction) | / | Enhanced (∼15%, red ripe) | Shorten | Tieman et al. (1992), Tieman and Handa (1994) | |
SlXTH5 | CRISPR | / | / | / | Reduced (LM25 to xyloglucan) | Enhanced (∼5% to 30%, B7) | / | Wang et al. (2022) | |
SlXTH1 | OE | / | / | / | Reduced (24% KOH-fraction) | Enhanced (∼25%, pink) | / | Miedes et al. (2010) | |
Pepper | CaCel1 | Antisense | / | / | Unaffected (both CDTA and Na2CO3-fraction) | Unaffected (8 M KOH-fraction) | / | / | Harpster et al. (2002a) |
Apple | MdPG1 | Antisense | Reduced | Reduced (water-soluble) | Reduced (CDTA-fraction, Jim5 to deesterified HG) | / | Enhanced (∼50% to 75%, ripe) | Extended | Atkinson et al. (2012) |
Strawberry | FaPG1 | Antisense | Reduced | Reduced | Reduced (both CDTA and Na2CO3-fraction; Jim5 to deesterified HG) | Unaffected | Enhanced (∼60%, ripe) | / | Posé et al. (2013) |
FaPL | Antisense | Reduced | Reduced (water-soluble) | Reduced (both CDTA and Na2CO3-fraction) | Reduced (4 M KOH-fraction) | Enhanced (∼40%, ripe) | / | Santiago-Doménech et al. (2008) | |
FvPLA | RNAi | Reduced | / | Reduced (Jim5 to deesterified HG) | / | Enhanced (∼50%, 20 dpa) | / | Zhang et al. (2022) |
/ Indicates that the information is missing.
Insights into fruit softening-related CW changes from gene-modified fruits
CW loosening
Normal growth involves enzyme access and assembly by glycosyltransferases, whereas softening during ripening involves the disassembly of the CW components by glycoside hydrolases (GHs). The same set of CW-associated gene families are induced in both processes, including the expansins (EXPs), xyloglucan endotransglucosylase (XET)/hydrolases (XTH), and endo-1,4-β-glucanases (EGase or Cel), which are important CW loosening agents. Different members of the same gene family may play different roles in fruit expansion and softening. Softening is most frequently measured by probe penetration or flat plate compression using a texture analyzer, and the maximum loads are used as the indicator of fruit firmness (Shi et al. 2021), whereas cell extension (creep) has been measured in young hypocotyl or onion (Allium cepa) epidermal CWs using an extensometer (Park and Cosgrove 2012).
EXPs, key nonenzymatic agents in CW loosening
EXPs were identified by Cosgrove's lab in 1992 and recognized as a key factor mediating acid-induced CW creep and wall relaxation during plant growth (McQueen-Mason et al. 1992). Three subfamilies have been identified: α-EXP, β-EXP, and microbial EXPs. Members of the α-EXPs have been shown to loosen the CW without hydrolyzing any of the CW polymers. Fruit softening-associated α-EXP members have been identified in a range of fruits, and their transcripts increase concurrently with fruit softening, suggesting that EXPs play a role in this process (Rose et al. 1997; Civello et al. 1999; Trivedi and Nath 2004; Palapol et al. 2015). Crude protein extracted from ripening avocado (Persea americana), pepper (Capsicum annuum), pear fruit, and different tomato fruit developmental stages were used to assay EXP activity in the CW creep test by an extensometer, and extracts from ripening fruits displayed creep activity (Rose et al. 2000).
In tomato, SlExp1 is specifically expressed during fruit ripening and is the most abundant EXP transcript (Rose et al. 1997). Transgenic lines with altered SlExp1 expression were generated and used for textural and CW polymer size distribution analysis (Brummell et al. 1999b). Suppression of SlExp1 by an antisense approach enhanced firmness at the Br (Breaker) stage by 13% compared to the control. Unexpectedly, size distribution analysis showed no substantial change in the molecular mass profile of either strong alkali-extractable hemicelluloses or xyloglucan, while the molecular mass profile of CDTA-extractable pectins changed from the MG (mature green) to the B12 (12 d after color break) with less pectin depolymerization in SlExp1-suppressed fruit compared to the control. Meanwhile, overexpression of SlExp1 accelerated softening and mature green fruits were 30% softer than controls. CW polymer molecular mass profile analysis showed that in SlExp1-overexpressing fruit, hemicelluloses, and xyloglucan were precociously depolymerized, while pectin depolymerization was unchanged. The difference in hemicelluloses and pectin depolymerization between SlExp1-suppressed and overexpressed fruit suggests that the regulation of fruit firmness by SlExp1 is an indirect effect. The suppression of SlExp1 at the ripening stage, which had a relatively small inhibitory effect on softening, may restrain the activity of pectolytic enzymes, including PG and PL discussed later, which are responsible for pectin solubilization and depolymerization (Smith et al. 1988; Schuch et al. 1989, 1991; Uluisik et al. 2016). Ectopic expression of SlExp1 in growing fruit may alter the access of CW lytic genes responding to fruit expansion-related CW loosening (e.g. SlXTH1 and SlCel7, Catalá et al. 1997, 2000), which may account for hemicellulose depolymerization.
Other CW loosening agents
XTH (belonging to the GH19 family with XET and xyloglucan endohydrolase activity) and Cel (Plant Cel belonging to the GH9 family) have also been considered as CW-loosening enzymes, and some gene family members that are expressed during softening in tomato, persimmon (Diospyros kaki), apple, and kiwifruit have been identified (reviewed by Goulao and Oliveira 2008). However, their role in CW loosening is less well understood.
In a previous CW model, xyloglucan was proposed to bind to and tether cellulose microfibrils to form a load-bearing network (Albersheim et al. 2011), whereas in the current model, cellulose–cellulose interactions create a load-bearing network, and xyloglucan interacts with the cellulose at specific biomechanical hotspots (Fig. 1). Evidence from in vitro wall creep tests indicated, however, that XTH does not cause appreciable CW loosening (McQueen-Mason et al. 1993; Cosgrove 2016). In tomato, SlXTH5 (identified as SlXTH14 in the tomato genome annotation) is highly expressed during ripening and acts as a transglucosylase in vitro enzyme activity assays. SlXTH5 recombinant protein can depolymerize xyloglucan rapidly in the presence of an acceptor (xyloglucan oligosaccharides), but cannot induce cucumber (Cucumis sativus) hypocotyl wall creep nor affect elastic or plastic extensibilities, suggesting that SlXTH5 is not a CW loosening agent (Saladié et al. 2006). More recently, Wang et al. (2022) showed that knockout of SlXTH5 by CRISPR resulted in slightly firmer fruit with increased paste viscosity and higher level of LM25 interaction (antibody to xyloglucan), and extracts from SlXTH5 knockout fruit showed lower xyloglucan-degrading activity compared to WT fruit, indicating SlXTH5 is involved in xyloglucan depolymerization. When SlXTH1, which is expressed during fruit growth, was overexpressed in tomato fruit, however, the firmness was higher and polymerization of hemicelluloses and xyloglucan extractable by a strong alkali solution was reduced compared to WT fruit (Miedes et al. 2010). These antagonistic effects on fruit softening suggest that XTHs may contribute to CW integrity by generating higher or lower molecular weight xyloglucan, but how XTHs precisely regulate fruit softening requires further exploration.
Fungal GH12 Cel (e.g. Cel12A), with both xyloglucanase and cellulase activity, induced cucumber hypocotyl CW creep, whereas GH12 Cels with either xyloglucanase or cellulase activity, were unable to cause wall creep (Park and Cosgrove 2012). Plant GH9-Cels have been reported that can hydrolyze cellulose and xyloglucan in vitro in rice (Oryza sativa) and poplar (Populus alba) (Ohmiya et al. 1995; Yoshida and Komae 2006), but the abilities of these enzymes, and indeed their fruit counterparts, to cause CW creep have not been tested. In tomato, antisense knockdown of SlCel1 and SlCel2, and antisense CaCel1 in pepper, had no effect on fruit softening (Lashbrook et al. 1998; Brummell et al. 1999a; Harpster et al. 2002a). The size distribution of CW fractions from CaCel1 antisense fruit showed no detectable changes in the CDTA- and Na2CO3-soluble pectins, matrix glycan (8 M KOH), and xyloglucan depolymerization during ripening, compared with controls (Harpster et al. 2002a). Similarly, overexpressing CaCel1 in tomato did not increase depolymerization of the xyloglucan strong alkali soluble fraction (24% KOH) compared to the control (Harpster et al. 2002b). These transgenic fruit studies suggest that Cel is not a determinant of fruit softening. Moreover, CW creep caused by the bifunctional Cel12A was much slower and smaller than that obtained with EXP (Park and Cosgrove 2012; Zhang et al. 2019), suggesting the role of Cel in wall loosening is probably negligible.
Changes to pectins during CW disassembly
CW weakening and disassembly are caused by solubilization and depolymerization of 2 main components, hemicellulose and pectin. The pectin-rich ML is connected to the primary CW, which also contains pectin (Fig. 1) and both undergo substantial biochemical changes during softening, whereas there are only limited changes in cellulose.
HG pectinase
HG pectinases are a group of enzymes that change in abundance during fruit ripening and that have been widely studied by genetic modification, including pectin methylesterases (PMEs), polygalacturonases (PGs, one of the first enzymes targeted at the end of the 1980s, which led to the first commercial GMO food), the PG β-subunit, and pectate lyases (PLs).
Pectin methylesterases
Fully methylesterified HG is biosynthesized in the Golgi and then secreted into the apoplast, where it is typically demethylesterified by PMEs during ripening, which is a necessary step for HG subsequent degradation by enzymes such as PG and PL. Although PMEs have been characterized in fruits (Goulao and Oliveira 2008), only 2 tomato SlPMEs have been targeted with a transgenic approach, SlPME2 (ripening-related) and SlPmeu1 (early development-related). Silencing SlPME2 or SlPmeu1 results in reduced PME activity but slightly firmer and softer fruit, respectively (Tieman et al. 1992; Hall et al. 1993; Tieman and Handa 1994; Phan et al. 2007). Pectin chemical analysis indicated that silencing SlPME2 increased the methylesterification of pectin and the molecular weight of EDTA-soluble pectins and reduced solubilization of EDTA-soluble pectin (Tieman et al. 1992), suggesting that altering the degree of pectin methylesterification affects pectin polymer metabolism. Intriguingly, SlPME2-suppressed fruit showed complete loss of tissue integrity after 7 wk of storage. Additionally, ion analysis and binding test indicated less calcium binding to pectin in SlPME2-suppressed fruit, suggesting that altering the degree of pectin methylesterification may affect the formation of egg-box structures (Fig. 1; Tieman and Handa 1994). Phan et al. (2007) also speculated that the softer texture of SlPMEU1-suppressed fruit might be due to the disruption of egg-box formation. Two possible actions by PME have been proposed: (i) blockwise demethylation promotes aggregation of HG pectin by binding calcium; (ii) random demethylation promotes the degradation of HG pectin by PG and PL (Hocq et al. 2017). Transgenic studies in tomato suggest that blockwise demethylation may play a dominant role, but the interconnected roles of PME and the pectolytic enzymes PG and PL, discussed below, need further investigation.
Polygalacturonase
PG is an endo-acting enzyme in the GH28 family that cleaves unesterified galacturonosyl linkages along the HG pectin backbone (Hobson 1964; Tucker et al. 1981, 1982). In tomato, SlPG is synthesized de novo during ripening (Tucker and Grierson 1982) and its transcript represents 2% of the total mRNA in ripening fruit (Della Penna et al. 1987). The cDNA and gene were sequenced long before the genome sequence became available (Grierson 1986; Bird et al. 1988) and it was concluded that the different forms of SlPG were derived from the same gene (Tucker et al. 1981).
Antisense suppression of SlPG2a in transgenic tomatoes (Sheehy et al. 1988; Smith et al. 1988) led to the retention of higher molecular weight pectin polymers and a reduced amount of water-soluble pectin at the ripening stage, enhanced juice viscosity, reduced fruit cracking, and extended shelf life (Sheehy et al. 1988; Smith et al. 1988; Schuch et al. 1989, 1991; Brummell and Labavitch 1997). Notably, however, there was little difference in the firmness of PG antisense tomatoes, and antisense fruit with <1% of normal PG activity had similar compression scores to fruit of untransformed lines (Schuch et al. 1991). More recently, the fruit of the SlPG2a knockout mutant generated by CRISPR was shown to soften normally as SlPG2a antisense fruit (Wang et al. 2019). Transmission electron micrographs and immunolocalization analysis indicate that knocking out SlPG2a increased cell-to-cell adhesion and retention of deesterified HG at TCJs (Wang et al. 2019) and this may help explain the earlier reported increase in resistance to damage during transport (Schuch et al. 1989, 1991). Thus, in tomato fruit, SlPG2a does affect pectin metabolism but inhibiting its activity has little effect on overall softening, measured by both compression and penetration (Schuch et al. 1991; Wang et al. 2019).
Experiments with members of the Rosaceae, however, indicate that inhibiting FaPG1 in strawberry and MdPG1 in apple by antisense strategies produced both firmer textured fruit, reduced solubilization and depolymerization of pectin, and reduced cell separation (Atkinson et al. 2012; Posé et al. 2013). Nonmelting-flesh peach, which shows limited postharvest softening are naturally deficient in endo-PG activity encoded by PpPG1 and PpPG2, and nonmelting-flesh peach display lower pectic solubilization and contain higher-molecular-weight pectic polymers than melting-flesh peach (with normal endo-PG activity), suggesting that endo-PG activity is associated with the melting flesh phenotype (Yoshioka et al. 2011).
Thus, antisense suppression of PG in different fruits results in similar inhibition of pectin polymer depolymerization, solubilization, and cell-to-cell separation during ripening. Its effect on softening, however, measured either by compression or probe penetration may be different in different fruits. This may also depend on the anatomy, wall composition, and the relative activities of other CW enzymes in different fruits.
The simultaneous transgenic suppression of SlPG2a and SlExp1 leads to significantly firmer fruit throughout ripening compared to either suppression of SlPG2a or SlExp1 alone (Kalamaki et al. 2003; Powell et al. 2003) and simultaneous suppression of SlPG2a and SlExp1 led to a dramatic reduction in the solubilization and depolymerization of water-soluble pectin, but not hemicellulosic polymers (Cantu et al. 2008). One possible explanation for the difference in the firmness of SlPG2a antisense fruit and SlPG2a and SlExp1 double suppressed fruit is that other HG-pectolyitic enzymes may partially complement when SlPG2a is suppressed along with the normal function of SlExp1, while the accessibility to these enzymes could be reduced in the fruit with simultaneous antisense of SlPG2a and SlExp1.
The PG β-subunit
The PG enzyme, extractable from ripe tomatoes, can degrade pectin substrates in vitro within a matter of minutes, yet this does not happen in vivo, suggesting that PG activity may be constrained in the cell. This could be explained by an accessibility difference or limitations imposed by PME activity. The polygalacturonase β-subunit, which was first characterized in tomato (Zheng et al. 1992), may play an important role in constraining PG activity. The polygalacturonase β-subunit is a CW glycoprotein, possibly related to a previously described factor that forms a stable heterodimer with SlPG2a, to form a complex named SlPG1 (Tucker et al. 1981). The amount of β-subunit protein remains stable throughout ripening although the transcript levels of PG β-subunit decrease (Zheng et al. 1994). Silencing the PG β-subunit resulted in softer fruit, particularly at the ripening stage. CW biochemical and micrographic analyses indicated that suppression of the PG β-subunit led to elevated levels of EDTA-soluble pectins with higher depolymerization at all stages, and ML dissolution (Watson et al. 1994; Chun and Huber 2000). Chun and Huber (2000) suggested that the PG β-subunit might be involved in softening by impeding SlPG2a activity. The PG β-subunit belongs to the BURP-domain (BNM2, USP, RD22, PG1β) class of proteins (Hattori et al. 1998). Interestingly, no other BUPRs have been characterized in fleshy fruits other than tomato. Recently, however, BUPRs have been found to interact with other CW proteins, such as GhRDL1 (AtRD22-Like 1) from cotton (Gossypium hirsutum) and GmRD22 (responsive to dehydration 22) from soybean (Glycine max), which interact with EXP and apoplastic peroxidase to regulate CW growth and integrity, respectively (Wang et al. 2012; Xu et al. 2013). This suggests that BURP-domain proteins function in fruit softening, possibly by tethering enzymes and modulating their activity and this needs further exploration.
Pectate lyase
Unlike PG, PL catalyzes the cleavage of unesterified galacturonosyl residues by the β-elimination reaction and targets specific locations between cells (Uluisik et al. 2016). Ripening-related PLs have been characterized in tomato, strawberry, banana, grape (Vitis vinifera), and mango (Mangifera indica) (Uluisik et al. 2016; Uluisik and Seymour 2020). PL was first silenced in ripening cultivated octaploid strawberry, where suppression of FaPL resulted in firmer fruit with a reduction in pectin solubilization and depolymerization (Jiménez-Bermúdez et al. 2002; Santiago-Doménech et al. 2008). More recently, another PL was silenced and overexpressed in wild diploid strawberry, and overexpressing FvPLA promoted precocious softening, while silencing FvPLA reduced the softening rate (Zhang et al. 2022). Additionally, changes in the ML and TCJs were consistently observed in FvPLA OE and RNAi fruits. In tomato, when ripening-specific SlPL was repressed or mutated, the fruit exhibited substantially enhanced firmness. SlPL is implicated in HG-rich pectin degradation in the TCJs between cells and the ML regions that limit cell separation (Uluisik et al. 2016; Wang et al. 2019). Immunolocalization studies with the SlPL knockout mutant showed not only higher levels of LM19 (antibody to deesterified-HG) labelling but also much higher levels of LM5 (antibody to the β-1,4-galactan side chains of RGI), suggesting that loss of the Gal side chain of RGI may be suppressed in the SlPL knockout mutant via an unknown mechanism (Wang et al. 2019). Moreover, SlPL RNAi fruit displayed an extended shelf life with limited softening 40 d after harvest (Yang et al. 2017). Both PL and PG attack deesterified-HG pectin and act on CW separation. So far, however, there have been no investigations of the effects of combining PG and PL knockouts on softening, and whether suppressing EXP can enhance the firm phenotype of PL knockout, and PG and PL double knockouts need further investigation (see Outstanding Questions). Interestingly, the Cnr tomato mutant, in which SlExp1, SlPG2a, and SlPL are suppressed, showed limited softening with stronger CWs (Box 2; Orfila et al. 2001, 2002; Manning et al. 2006; Zhong et al. 2013).
Map-based cloning of the naturally occurring pleiotropic tomato mutants rin, nor, and Cnr, which affect many aspects of ripening, including limited softening, identified the RIN, NOR, and CNR TFs (Vrebalov et al. 2002; Giovannoni et al. 2004; Manning et al. 2006). Subsequently, CRISPR experiments showed that these were all gain-of-function mutants (discussed by Li et al. 2021). Genetic mapping and expression analysis identified SPL-CNR at the Cnr locus, which encoded an SBP-box protein. The gene had a hyper-methylated promoter and WT coding sequence (Manning et al. 2006), suggesting the mutation involves changes in methylation affecting gene expression. A less severe phenotype was shown by wild-type tomato fruit in which Cnr was knocked out by CRISPR, indicating this is a complex locus requiring further study (discussed by Li et al. 2021). The pericarp of Cnr fruit contains approximately 50% more intercellular space, leading to reduced cell-to-cell adhesion and a floating fruit phenotype, which might be due to the lack of PME activity in Cnr (Orfila et al. 2001, 2002; Zhong et al. 2013). Additionally, immunocytochemistry in Cnr revealed a partially absent PAM1 (specific to large stretches or blocks of deesterified HG) epitope in the intercellular space and ML, and disrupted deposition of (1–5)-α-arabinan (Orfila et al. 2001, 2002). CW material extracted from Cnr could not swell in vitro, suggesting Cnr fruit contain stronger CWs, which eliminates the negative effect caused by abnormal cell-to cell separation, similar to what occurs in the SlTBG4 knockout mutant (Wang et al. 2019), indicating that wall strength as well as cell adhesion play a pivotal role in attenuating softening. All these spontaneous mutants show changes in expression of key CW genes, which is consistent with their role in softening, and rin has been used in conventional breeding to obtain longer shelf-life tomato fruit.
RGI pectinases
Exo-galactanase/β-galactosidase (β-Gase, which belongs to GH35) and α-L-arabinofuranosidase (α-AFase, which belongs to GH3 or GH51) cleave the galactosyl and arabinosyl residues, respectively, on the side chain of RGI. Several β-Gase- and α-AFase-encoding genes have been characterized in fruits (Smith and Gross 2000; Itai et al. 2003; Sekine et al. 2006; Di Santo et al. 2009; Wei et al. 2010; Tateishi et al. 2014), due to the observed loss of Gal and Ara side chains during ripening but few have been targeted for transgenic studies. In tomato, SlTBG1 (tomato β-galactosidase 1) and SlTBG4 were silenced using antisense technology (Carey et al. 2001; Smith et al. 2002), but only suppression of SlTBG4 showed a textual phenotype, with fruit 40% firmer than the control and lower exo-galactanase levels during the early stages of ripening (Smith et al. 2002). More recently, an SlTBG4 knockout mutant showed consistently lower exo-galactanase levels, but similar firmness in comparison to the control, with slightly enhanced juice viscosity (Wang et al. 2019). The reported texture of the SlTBG4 mutant, however, contrasted with the previous report (Smith et al. 2002), and the authors hypothesized that different effects might be observed with different tomato genetic backgrounds (Wang et al. 2019). Further characterization of CW pectin fractions of the SlTBG4 mutant using pectin antibody probes revealed a continuously higher level of galactan-rich pectin in all CW fractions but no significant change in RGI, suggesting that side chain cleavage by SlTBG4 did not affect the solubility of RGI pectin. Nevertheless, elevated levels of water- and CDTA-soluble deesterified HG and a larger intracellular space were observed in the SlTBG4 mutant versus the control (Wang et al. 2019). In the SlTBG4 mutant, the abnormal degradation of deesterified HG in TCJs did not lead to a softer phenotype, however, which might be due to a stronger CW related to the reduced side chain cleavage. Interestingly, an early developmentally-expressed TBG, SlTBG6, was silenced by Moctezuma et al. (2003), resulting in a cracking phenotype and a reduction in firmness at 20 dpa (days post anthesis), but no difference was observed at the ripening stage, although underlying CW modifications were not examined (Moctezuma et al. 2003). In strawberry, antisense suppression of ripening-related FaβGal4 unexpectedly also caused down-regulation of FaβGal1, resulting in a firmer texture. CW chemical analysis showed that silencing FaβGal4&1 resulted in an increased Na2CO3-extractable fraction and a larger amount of galactose in the 1 M KOH faction (Paniagua et al. 2016).
GH3-α-AFase enzymes are usually bifunctional, with α-AFase/β-xylosidase (Arf/Xyl) activity, while GH51 enzymes (α-AFases) lack β-xylosidase activity (Lagaert et al. 2014). In tomato, 2 ripening-related (but not ripening-specific) GH3 gene members, SlXYL1 and SlArf/Xyl1, have been identified (Itai et al. 2003; Tateishi et al. 2014). When SlArf/Xyl2, a GH3-α-AFase gene expressed during early fruit development, was suppressed by antisense in tomato, there were no apparent changes in fruit expansion and CW polysaccharides neutral sugar composition. Additionally, both α-AFase and β-xylosidase activities were unchanged in SlArf/Xyl2 antisense fruits due to the induction of a GH51-α-AFases coding gene SlARF2 (Tateishi et al. 2014). This suggests that before conclusions can be drawn about the results of gene knockouts, it is important to examine whether their function can be complemented by other family members or related genes.
Conclusion and future perspectives
Research on modifying the expression of CW genes has provided insights into softening, prolonging shelf life, and improving disease resistance. CW loosening, pectin degradation, and dissolution of the ML and TCJs are implicated in CW disassembly and are key contributors to fruit softening (Table 1). The roles of RGI side chain loss, xyloglucan depolymerization, and cellulose metabolism are still obscure. RGI side chain loss may increase pectin porosity and allow other lytic enzymes access to their substrates, which is potentially similar to what occurs during CW loosening. Fruit ripening is mainly caused by the nonenzymatic action of EXP, which probably disrupts the xyloglucan-cellulose amalgam to increase the accessibility of substrates for CW enzymes. However, CW loosening itself cannot hydrolyze the CW components, and the effects on CW polymers depend on spatially and temporally expressed local lytic enzymes (Brummell et al. 1999b; Cantu et al. 2008). PG seems to be relatively unimportant in regulating softening in tomato but is more important in fruits of the Rosaceae. PL modulates softening in strawberry, and it has been demonstrated to be an important softening modifier in tomato. SlPL-suppressed tomato fruits possess a substantially firmer texture, prolonged shelf life, and reduced infection during postharvest, without sacrificing quality (Santiago-Doménech et al. 2008; Uluisik et al. 2016; Yang et al. 2017).
Genetic strategies have mostly targeted individual genes, but fruit softening is a multigenic trait and several different enzymes contribute to CW changes in different fruits. Inhibiting SlPG2a and SlExp1 produced firmer tomato fruit (Powell et al. 2003; Cantu et al. 2008) and multigene modification is a viable approach to making further progress, as is suppression of regulatory factors (e.g. TF SlLOB1 [lateral organ boundaries 1], Shi et al. 2021) which regulate multiple softening enzymes. With technological development, multigene genetic modification can be achieved by one-time tissue culture without traditional cross-breeding using CRISPR/Cas9 (Yang et al. 2022). Insights from polysaccharide interactions by NMR studies (Wang et al. 2015; Wang and Hong 2016) and CW protein interactions revealed by pathogens and stress responses (Su and Higashiyama 2018; Herger et al. 2019) could also be incorporated into ripening studies. In addition to pectin metabolism, it is also important to know how xyloglucan depolymerization or microarchitectural changes in cellulose configuration affect softening.
What is the role of EXPs during softening? How can we build a system to test fruit softening-wall loosening as has been done to investigate acid growth-wall creep measured by an extensometer?
How do enzymes with antagonistic effects, including PME and XTH, precisely regulate softening?
How do CW glycoproteins interact with CW polymers or lytic enzymes to promote fruit softening?
What plays a dominant role in fruit softening, CW degradation, or ML dissolution, and what will happen if both paths are diminished?
Which gene combinations can improve textural characteristics using multigene genetic modification? What texture would be generated by knockout of PG + PL + EXP and overexpression of PG β-subunit?
Author contributions
K.C. and D.G. conceived the contents of the manuscript. Y.S. and D.G. wrote the manuscript. Y.S. and B.L. generated the figure. K.C. and D.G. revised the manuscript.
Funding
This work was supported by the National Key Research and Development Program of China (2022YFD2100102), National Natural Science Foundation of China (32030082), the 111 Project (B17039), Ningbo Key Research and Development Program (2022Z179) and Zhejiang Provincial Cooperative Extension Project of Agricultural Key Technology (2022XTTGGP01).
References
Author notes
The author responsible for distribution of materials integral to the findings presented in this article in accordance with the policy described in the Instructions for Authors (https://dbpia.nl.go.kr/plphys/pages/General-Instructions) are Kunsong Chen ([email protected]), Donald Grier ([email protected]).
Conflict of interest statement. None declared.