-
PDF
- Split View
-
Views
-
Cite
Cite
Qiqi Zhao, Rui Liu, Qinzheng Zhou, Jie Ye, Fanwei Meng, Jun Liu, Chao Yang, Calcium-binding protein OsANN1 regulates rice blast disease resistance by inactivating jasmonic acid signaling, Plant Physiology, Volume 192, Issue 2, June 2023, Pages 1621–1637, https://doi.org/10.1093/plphys/kiad174
- Share Icon Share
Abstract
Rice blast, caused by the fungal pathogen Magnaporthe oryzae, is one of the most devastating diseases in rice (Oryza sativa L.). Plant annexins are calcium- and lipid-binding proteins that have multiple functions; however, the biological roles of annexins in plant disease resistance remain unknown. Here, we report a rice annexin gene, OsANN1 (Rice annexin 1), that was induced by M. oryzae infection and negatively regulated blast disease resistance in rice. By yeast 2-hybrid screening, we found that OsANN1 interacted with a cytochrome P450 monooxygenase, HAN1 (“HAN” termed “chilling” in Chinese), which has been reported to catalyze the conversion of biologically active jasmonoyl-L-isoleucine (JA-Ile) to the inactive form 12-hydroxy-JA-Ile. Pathogen inoculation assays revealed that HAN1 was also a negative regulator in rice blast resistance. Genetic evidence showed that OsANN1 acts upstream of HAN1. OsANN1 stabilizes HAN1 in planta, resulting in the inactivation of the endogenous biologically active JA-Ile. Taken together, our study unravels a mechanism where an OsANN1–HAN1 module impairs blast disease resistance via inactivating biologically active JA-Ile and JA signaling in rice.
Introduction
Plants face various attacks from a great number of pathogenic organisms throughout their lifetime. In such unfavorable circumstances, plants have evolved a powerful immune system comprising of several defense layers (Jones and Dangl 2006; Zhou and Zhang 2020). The first layer is the pathogen-associated molecular pattern (PAMP)-triggered immunity (PTI), which is activated by plant cell-surface localized pattern recognition receptors upon perception of microbial PAMPs (Macho and Zipfel 2014). PTI responses include increased generation of reactive oxygen species (ROS), activated Mitogen-activated protein kinase (MAPK) cascade, upregulated expression of pathogenesis-related genes, altered phytohormone signaling, and increased callose deposition (Dodds and Rathjen 2010). The second layer is the effector-triggered immunity (ETI), which is initiated by specific recognition of pathogen-secreted effectors with plant resistance (R) proteins (Dodds and Rathjen 2010). ETI defense responses are much stronger than those of PTI, which is often coupled with a hypersensitive response that leads to plant cell death at the infection site (Alhoraibi et al. 2019). Although the 2 layers of immune system exhibit different activation mechanisms and require different early signal components, PTI and ETI are evolutionarily interconnected as they eventually converge into many similar downstream responses (Yuan et al. 2021).
Annexins are a subfamily of phospholipid- and Ca2+-binding proteins that are widely distributed in Eukaryotes and Prokaryotes (Laohavisit and Davies 2011; Jami et al. 2012). The annexin proteins have diverse biological functions, including Ca2+ channel-regulating activity, peroxidase and ATPase/GTPase activities, actin binding, and callose synthase regulation (Laohavisit and Davies 2011). Plant annexins regulate diverse aspects of plant growth, development, and stress responses (Clark et al. 2012; Laohavisit et al. 2013; Yadav et al. 2018; He et al. 2020). AtANN1 (Annexin 1 in Arabidopsis thaliana) is the most widely studied plant annexin to date. It has been known that AtANN1 is a Ca2+-permeable transporter that mediates the accumulation of cytosolic free Ca2+ in response to ROS, high salinity, ABA, cold, and heat stress (Lee et al. 2004; Konopka-Postupolska et al. 2009; Laohavisit et al. 2013; Wang et al. 2015; Liu et al. 2021). In Arabidopsis, AtANN4 forms a homodimer in a Ca2+-dependent manner to cooperatively regulate the drought and salt stress responses (Huh et al. 2010). Similarly, in rice (Oryza sativa L.), OsANN1, OsANN3, OsANN4, and OsANN10 are reported to respond to heat, drought, ABA, and osmotic stress, respectively (Qiao et al. 2015; Li et al. 2019; Gao et al. 2020; Zhang et al. 2021). Of which, OsANN1 has ATPase activity, and is capable of binding Ca2+, and increases heat tolerance by modulating antioxidant accumulation in rice (Qiao et al. 2015). Nevertheless, the roles of annexins in plant immunity have not been characterized yet. In Arabidopsis, the bacterial pathogen Pseudomonas syringae infection induces AtANN4 expression, whereas Pseudomonas fluorescens and cucumber mosaic virus infection inhibit AtANN4 expression (Marathe et al. 2004; Wang et al. 2005; Truman et al. 2007). Recently, AtANN8 was reported to negatively regulate plant powdery mildew resistance by enhancing H2O2 accumulation triggered by the broad-spectrum resistance gene RPW8.1 (Resistance to Powdery Mildew 8.1) (Zhao et al. 2021).
Jasmonic acid (JA), salicylic acid (SA), and their derivatives are well-known “defense hormones” in regulating plant disease resistances (Fonseca et al. 2009; Robert-Seilaniantz et al. 2011; Wang et al. 2019; Zhou et al. 2019). In general, SA is involved in defending against the biotrophic or hemibiotrophic pathogens and sucking insects, whereas JA triggers plant immunity against the necrotrophic pathogens and chewing insects (Spoel and Dong 2008; Campos et al. 2014). Increasing body of evidence suggests that JA has a favorable effect on plant immunity in recent years (Spoel and Dong 2008; Campos et al. 2014; Wasternack and Song 2017).
Jasmonate is synthesized from the oxygenation of the α-linolenic acid in chloroplasts, and then proceeds through a series of reactions catalyzed by 13-lipoxygenase (LOX), allene oxide synthase (AOS), and allene oxide cyclase (AOC) to produce oxophytodienoic acid (OPDA). OPDA is transported into the peroxisomes and catalyzed by OPDA reductase 3 (OPR3) and acyl-CoA oxidase 1 (ACX1) to produce JA. In cytoplasm, JA can be converted into a variety of derivatives (Wasternack and Song 2017). Importantly, JA is conjugated with isoleucine by Jasmonate Resistant 1 (JAR1) to produce jasmonoyl-L-isoleucine (JA-Ile), which is the bioactive JA (Fonseca et al. 2009; Wang et al. 2019). Upon JA-Ile perception, the F-box receptor protein COI1 (Coronatine Insensitive 1) interacts with JA ZIM domain (JAZ) protein. JAZ proteins function as repressors of JA-responsive transcription factors to prevent the activation of JA-responsive genes. COI1 targets JAZs for ubiquitination by the E3 ligase of SCFCOI1 and the degradation of JAZ proteins by the 26S proteasome activates the expression of JA-responsive genes (Wasternack and Song 2017; Wang et al. 2019). In addition, bioactive JA-Ile can be oxidized to the inactive 12-hydroxy-JA-Ile (12OH-JA-Ile) by a cytochrome P450 protein CYP94B3 in Arabidopsis and its putative ortholog HAN1 in rice to serve as a negative feedback control on JA-Ile levels (Koo et al. 2011; Mao et al. 2019).
Rice is a staple crop that feeds more than half of the world's population. Rice blast disease caused by the fungal pathogen Magnaporthe oryzae leads to great losses in rice production yearly (Liu et al. 2014). Characterizing the genes that regulating the basal defense is a promising approach to control blast disease in rice (Takatsuji 2014). Here, we report that a rice annexin gene OsANN1 negatively regulates the resistance against rice blast disease. Interestingly, OsANN1 interacts with and stabilizes HAN1 to inactivate the biologically active JA-Ile. We unravel a mechanism where the OsANN1-HAN1 module impairs the blast disease resistance by tuning down the JA-mediated plant immune response in rice.
Results
OsANN1 transcription is induced by M. oryzae infection and PAMPs
Rice genome harbors 10 annexin genes and some of them have been shown to be transcriptionally induced by abiotic stresses and to regulate stress responses (Jami et al. 2012; Yadav et al. 2018). To explore the roles of annexin genes in rice, we first examined their expression in M. oryzae-inoculated rice plants. The transcriptome data showed that OsANN1, OsANN5, and OsANN8 were significantly induced during early M. oryzae infection (24 h postinoculation, hpi) (Fig. 1A). OsANN1 encoding protein contains 4 tandem annexin domain architectures and is closely related to the homologs of maize (Zea mays), as revealed by the phylogenetic analysis (Supplemental Fig. S1, A and B). Previously, OsANN1 was reported to be involved in the response to heat stress (Qiao et al. 2015). To validate the transcriptome data, we examined OsANN1 expression levels after M. oryzae infection. The OsANN1 transcription was induced after inoculating with M. oryzae and the expression levels peaked at 24 hpi; after which the expression levels decreased (Fig. 1B). We also examined the OsANN1 expression during PTI by treating the rice suspension cells with chitin and bacterial flagella-derived 22 (flg22) peptide. The transcription of OsANN1 was significantly increased in response to both PAMPs, suggesting that OsANN1 is a PAMP-responsive gene (Fig. 1C).
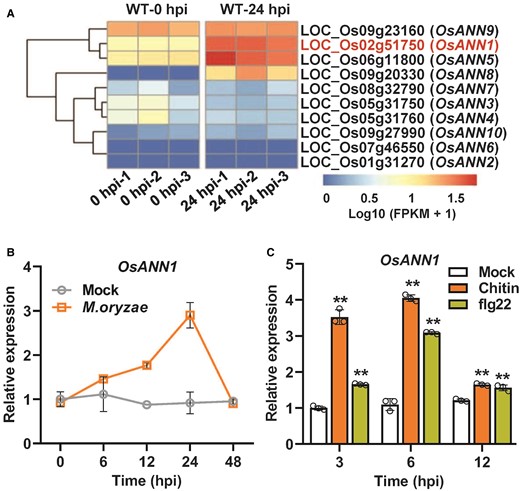
OsANN1 transcription is induced by M. oryzae infection and PAMP treatments. A) Heatmap analysis of rice annexin genes using transcriptome data of M. oryzae-inoculated rice plant leaves. B) Transcription analysis of OsANN1 over time after inoculation with M. oryzae in rice. Conidial suspensions (1 × 105 conidia mL−1 in 0.02% Tween-20) were sprayed onto the leaf surface of 3-wk-old rice seedlings. The inoculated leaves were sampled at indicated time points for RT-qPCR assays. 0.02% Tween-20 serves as mock treatment. hpi, hours postinoculation. Values are means ± Sd (n = 3). C) Transcription analysis of OsANN1 in rice suspension cells treated with 10 nM chitin or 500 nM flg22. RT-qPCR were used to evaluate the gene expression. Values are means ± Sd (n = 3), each circle represents 1 biological replicate. **Significant differences from Mock at P < 0.01 (Student's t-test).
OsANN1 negatively regulates the plant disease resistance
To explore the potential roles of OsANN1 in rice immunity, we challenged 2 independent RNA interference (RNAi) lines of OsANN1 with the M. oryzae isolate ZHONG-84-10 using spraying inoculation assays (Supplemental Fig. S2A) (Qiao et al. 2015). Both lines exhibited significantly less disease lesions and fungal biomass than those on the WT plants (Fig. 2A; Supplemental Fig. S2B), indicating that the RNAi lines are more resistant to blast fungus. We also obtained an OsANN1 knockout mutant (OsANN1-Cas9) using the CRISPR-Cas9 system, which carried a 19-bp nucleotides deletion in the genomic DNA, resulting in the early termination of translation (Supplemental Fig. S2C). Consistently, the OsANN1-Cas9 plants were also more resistant to M. oryzae than the ZH11 (Zhonghua 11) (Fig. 2B). We further expressed OsANN1, driven by a constitutive cauliflower mosaic virus (CaMV) 35S promoter, in rice plants and selected 2 OsANN1-overexpression (OX) lines (Supplemental Fig. S2A). Overexpressing OsANN1 in rice led to decreased disease resistance, revealed by much more lesions and fungal biomass in the 2 OsANN1-OX lines (Fig. 2C; Supplemental Fig. S2D). We next examined the infection process of M. oryzae at the cellular level using the rice leaf sheath to test the appressorial penetration rates and invasive hyphae (IH) extension ability in plants. In the OsANN1-RNAi plants, almost 43% appressoria did not form the primary IH, and extension of IH was markedly slower than that in WT at 24 hpi. However, in the OsANN1-OX plants, only 8% appressoria failed to penetrate the leaf epidermis, and the IH not only filled the first-infected cells but also invaded the neighboring cells (Supplemental Fig. S2, E and F). In addition, we carried out the punch inoculation assays with the leaves of WT, OsANN1-RNAi, and OsANN1-OX. The OsANN1-RNAi lines had significantly smaller lesions than WT, whereas the OsANN1-OX leaves displayed larger lesions (Supplemental Fig. S2G). Collectively, our data indicate that OsANN1 is a negative regulator of rice resistance against blast fungus.
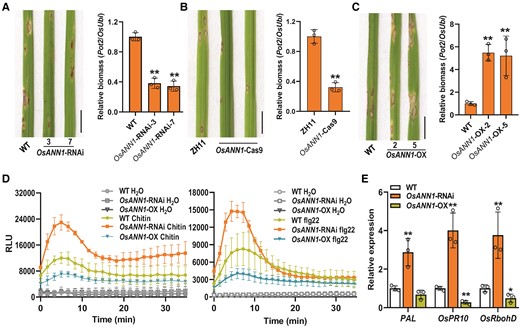
OsANN1 is a negative regulator of blast disease resistance in rice. A) Disease symptoms and relative fungal biomass of the WT and OsANN1-RNAi plants after M. oryzae infection. Conidial suspensions (1 × 105 conidia mL−1 in 0.02% Tween-20) were sprayed onto the leaf surface of 3-wk-old seedlings. Images were taken at the same time (5 dpi). Bar = 1 cm. The fungal biomass was determined by qPCR of M. oryzae Pot2 gene against rice OsUbi1 gene. Values are means ± Sd (n = 3 biological replicates). **Significant differences from WT at P < 0.01 (Student's t-test). B) Disease symptoms and relative fungal biomass of the ZH11 (Zhonghua 11) and OsANN1-Cas9 plants after M. oryzae infection. Others are as in A). C) Disease symptoms and relative fungal biomass of the WT and OsANN1-OX plants after M. oryzae infection. Others are as in A). D) ROS burst induced by PAMPs in suspension cells of the WT and OsANN1 transgenic plants; 10 nM chitin and 500 nM flg22 were used for the ROS burst assays. H2O served as the negative control. RLU, relative light units. Values are means ± Sd (n = 3). E) The expression of defense genes PAL, OsPR10, and OsRbohD in the WT and OsANN1 transgenic plants. The leaves of 4-wk-old plants were sampled, and RT-qPCR was used to evaluate the gene expression. Values are means ± Sd (n = 3 biological replicates). ** and * indicate significant differences from WT at P < 0.01 and 0.05, respectively (Student's t-test).
Furthermore, we challenged the OsANN1 transgenic lines with the bacterial blight pathogen Xanthomonas oryzae pv oryzae (Xoo) PXO99. The OsANN1-RNAi lines exhibited significantly shorter disease lesions on the inoculated leaves than the WT plants, whereas the OsANN1-OX leaves had much longer lesions (Supplemental Fig. S2H). Taken together, our findings indicate that OsANN1 negatively regulates rice immunity against both fungal blast and bacterial blight diseases.
To better understand the role of OsANN1 in rice blast resistance, ROS burst in suspension cells generated from the WT and OsANN1 transgenic plants was examined after PAMP treatments. Consistent with the disease resistance phenotype, the OsANN1-RNAi cells exhibited more robust oxidative burst than that in WT after chitin or flg22 treatments, whereas the OsANN1-OX cells exhibited much weakly response (Fig. 2D). These data suggest that OsANN1 negatively contributes to the ROS burst in PTI signaling. Furthermore, we evaluated various defense gene expression in the OsANN1 transgenic seedlings using RT-qPCR. Indeed, the expression levels of PAL, OsPR10, and OsRbohD were significantly upregulated in the OsANN1-RNAi plants, whereas their expression was repressed in the OsANN1-OX plants (Fig. 2E). Together, these results indicate that OsANN1 is a negative regulator of blast disease resistance in rice.
Notably, the OsANN1-RNAi plants exhibited reduced length of the second leaf sheath at seedlings stage and showed obviously semidwarf phenotype at heading stage; by contrast, the OsANN1-OX plants had much longer second leaf sheath and displayed enhanced plant height than WT (Supplemental Fig. S3, A to D), suggesting that OsANN1 is involved in rice growth and development.
Transcriptome analysis of OsANN1-regulated genes
To investigate the potential OsANN1-regulated genes that are responsible for rice disease resistance, we performed transcriptome analysis (RNA-seq) of WT and OsANN1-OX leaves harvested before (0 h) and 24 h postpathogen inoculation. We first identified 8,838 (4,286 downregulated and 4,552 upregulated) differentially expressed genes (DEGs) and 8,082 (4,111 downregulated and 3,971 upregulated) DEGs based on the criteria fold change > 2 and P < 0.01 in WT and OsANN1-OX leaves after pathogen inoculation when compared with the untreated plants, respectively (Fig. 3A; Supplemental Data Set 1). To explore the functions of OsANN1 in rice blast resistance, we conducted the functional analysis of DEGs in WT and OsANN1-OX. The DEGs of WT and OsANN1-OX were enriched into 355 and 324 Gene Ontology (GO) terms in biology progresses, respectively. Of which, 53 GO terms were specifically associated with OsANN1 (Fig. 3B). Functional annotations further revealed that most specific GO terms in OsANN1-OX were related to cell wall biogenesis, regulation of response to stress, secretion, and response to JA (Fig. 3C). Plant annexins are a type of Ca2+-binding proteins (Laohavisit and Davies 2011). Indeed, some DEGs in OsANN1-OX were specifically enriched into Ca2+ transmembrane transport process (Fig. 3, C and D). Notably, many endoplasmic reticulum (ER)-related pathways were also specifically enriched in OsANN1-OX, suggesting that OsANN1 might participate in ER-mediated functions (Fig. 3C). More importantly, JA and other disease resistance-related GO terms were also specifically enriched in the OsANN1-OX plants, indicating that OsANN1 may participate in blast resistance mediated by JA-related immune signal pathway (Fig. 3, C and D). Further analysis revealed that most genes involved in JA pathway were downregulated, which echoed the susceptibility of OsANN1-OX plants to M. oryzae infection (Fig. 3D; Supplemental Data Set 2).
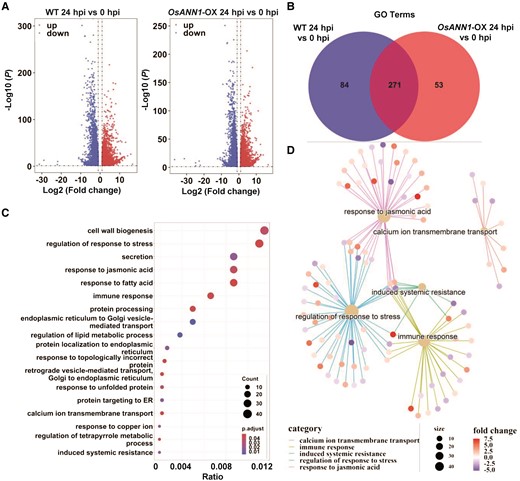
Transcriptome analysis of the OsANN1-regulated DEGs. A) Volcano plots of DEGs affected by M. oryzae inoculation in the WT and OsANN1-OX-2 plants, respectively. Rice plants were infected by M. oryzae for 24 h and the transcriptome was analyzed. DEGs were identified using adjusted P value <0.01 and absolute log2-fold change > 1 as the criteria. B) Venn diagram showing the unique number of GO terms of the WT and OsANN1-OX-2 after M. oryzae inoculation. Enriched GO terms were identified at P value <0.05. C) JA, ER, and immunity-related pathways are specifically enriched in the OsANN1-OX plants after M. oryzae inoculation. D) Most JA- and immunity-related genes were downregulated in the OsANN1-OX plants after M. oryzae inoculation.
OsANN1 interacts with HAN1
To further investigate the regulatory mechanism underlying the OsANN1-mediated disease resistance, we conducted a yeast 2-hybrid (Y2H) screening against a rice cDNA library using OsANN1 as a bait. Interestingly, we identified a cytochrome P450 monooxygenase as a candidate interacting protein of OsANN1, named HAN1, which was reported to catalyze the conversion of biologically active JA-Ile to the inactive form 12OH-JA-Ile (Mao et al. 2019). Our transcriptome data have revealed that JA-related pathways were enriched in inoculated OsANN1-OX plants (Fig. 3, C and D), implying the potential role of HAN1 in this case. We further confirmed that OsANN127–314, a N-terminus truncated version of OsANN1, interacted with HAN1 in yeast, but not the full length of OsANN1 (Fig. 4A). HAN1 contains a signal peptide at its N-terminus and a big domain of P450 (from amino acids 25 to 527) (Supplemental Fig. S4A). We further confirmed that OsANN127–314 interacted with the P450 domain of HAN1 (Fig. 4A). GST pull-down assays revealed that recombinant GST-OsANN1 successfully pulled down MBP-HAN1nsp (nsp, no signaling peptide), but GST alone did not (Fig. 4B). The interaction between OsANN1 and HAN1 was further validated by the co-immunoprecipitation (Co-IP) assays. The recombinant HAN1-FLAG and OsANN1-T7 proteins were coexpressed in Nicotiana benthamiana leaves. The result showed that OsANN1 interacted with HAN1, but it did not interact with GFP (Fig. 4C). In addition, we performed the split-luciferase and bimolecular fluorescence complementation (BiFC) assays and found that OsANN1 interacted with HAN1 at the ER (Fig. 4, D and E). Collectively, the above results demonstrated that OsANN1 interacted with HAN1 in vitro and in vivo.
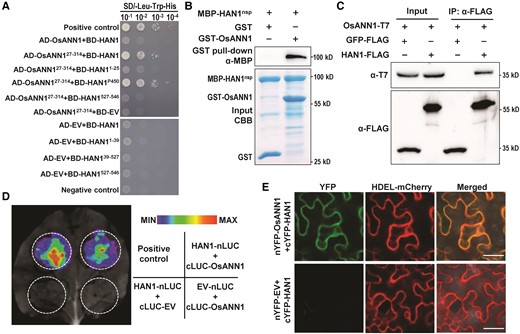
OsANN1 interacts with HAN1 in vitro and in vivo. A) OsANN127–314 interacts with HAN1 in yeast. The plasmids were cotransformed into yeast cells and screened on a synthetic dextrose media lacking leucine and tryptophan (SD-2). Yeast cotransformed with pGADT7-T + pGBKT7-53 served as a positive control. Yeast cotransformed with pGADT7-T + pGBKT7-lam served as a negative control. EV, empty vector. B) OsANN1 interacts with HAN1nsp by GST pull-down assays. The recombinant GST-OsANN1 and the MBP-HAN1nsp were subjected to GST pull-down analysis. GST served as a negative control. The interacting proteins were revealed by anti-MBP immunoblotting. CBB, Coomassie Brilliant Blue. C) HAN1 interacts with OsANN1 in the Co-IP assays. The proteins OsANN1-T7, HAN1-FLAG, and GFP-FLAG were coexpressed in N. benthamiana. Extracted proteins were immunoprecipitated using anti-FLAG beads, and Co-IP proteins were immunoblotted with an anti-T7 antibody. The western blots were captured using a chemiluminescent imaging system coupled to a CCD camera. D) HAN1 interacts with OsANN1 by the split luciferase assays in N. benthamiana. Nicotiana benthamiana leaves were coinfiltrated with 35S:HAN1-nLUC and 35S:cLUC-OsANN1. Luciferase complementation imaging assays were performed 2 d later. E) HAN1 interacts with OsANN1 by the split YFP assays. The experiment procedure was similar to that used in D). HAN1 was fused with cYFP at N-terminal, and OsANN1 was fused with nYFP at N-terminal. HDEL is the ER localization marker. The images were observed under a confocal microscope 2 d later. Bar = 25 μm.
HAN1 negatively regulates the disease resistance in rice
Phylogenetic analysis showed that HAN1 is clustered to the cytochrome P450 CYP94B3 superfamily and is closely related to the homologs of Brachypodium distachyon (Supplemental Fig. S4B). The subcellular localization analysis indicated that HAN1 is an ER-located protein (Supplemental Fig. S4C). HAN1 has previously been reported as a negative regulator of chilling tolerance via oxidizing the biologically active JA-Ile and fine-tuning the JA-mediated chilling response in rice (Mao et al. 2019). However, the function of HAN1 in rice disease resistance is not known. Thus, we examined HAN1 expression in rice leaves during the blast infection. We found that HAN1 expression was significantly elevated following pathogen inoculation (Fig. 5A). We also found that both chitin and flg22 significantly induced HAN1 expression in rice suspension cells (Fig. 5B).
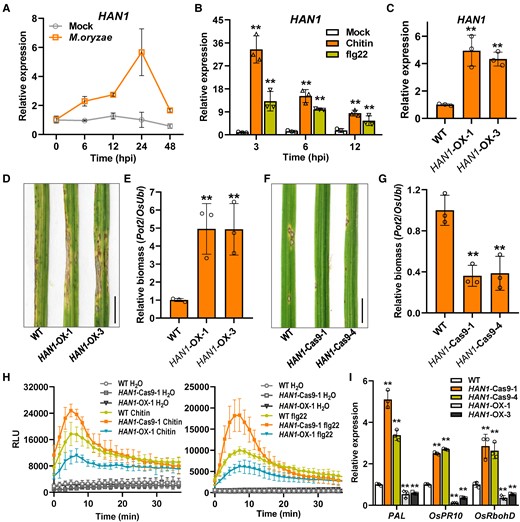
HAN1 negatively regulates the blast disease resistance in rice. A) Transcription analysis of HAN1 over time after inoculation with M. oryzae in rice. Conidial suspensions (1 × 105 conidia mL−1 in 0.02% Tween-20) were sprayed onto the leaf surface of 3-wk-old rice seedlings. The infected leaves were sampled at indicated time points for RT-qPCR assays. 0.02% Tween-20 serves as mock treatment. Values are means ± Sd (n = 3). B) Transcription analysis of HAN1 in rice suspension cells treated with 10 nM chitin or 500 nM flg22. RT-qPCR were used to evaluate the gene expression. Values are means ± Sd (n = 3 biological replicates). **Significant differences from Mock at P < 0.01 (Student's t-test). C)HAN1 expression levels in HAN1 transgenic plants. HAN1-OX-1 and -3 are 2 independent transgenic lines. Values are means ± Sd (n = 3). **Significant differences from WT at P < 0.01 (Student's t-test). D, E) Disease symptoms and relative fungal biomass of WT and HAN1-OX lines after M. oryzae infection. Conidial suspensions (1 × 105 conidia mL−1 in 0.02% Tween-20) were sprayed onto the leaf surface of 3-wk-old seedlings. Images were taken at the same time (5 dpi). Bar = 1 cm. The fugal biomass was determined by qPCR of M. oryzae Pot2 gene against rice OsUbi1 gene. Others are as in C). F, G) Disease symptoms and relative fungal biomass of WT and HAN1-Cas9 lines after M. oryzae infection. Two independent knock-out lines generated by CRISPR/Cas9 technique were used for the assays. Others are as in D). H) ROS burst induced by PAMPs in suspension cells of WT and HAN1 transgenic plants; 10 nM chitin (left) and 500 nM flg22 (right) were used for the ROS burst assays. H2O served as the negative control. RLU, relative light units. Values are means ± Sd (n = 3). I) The expression of defense genes PAL, OsPR10, and OsRbohD in WT and HAN1 transgenic plants. The leaves of 4-wk-old plants were sampled and RT-qPCR was used to evaluate the gene expression. OsActin2 was used as the internal reference gene. Others are as in C).
We then generated the HAN1-OX plants driven by a 35S promoter and obtained 2 HAN1-OX lines that were confirmed with elevated HAN1 expression levels by RT-qPCR (Fig. 5C). We also generated 2 HAN1 knockout lines (HAN1-Cas9) using CRISPR-Cas9 techniques (Supplemental Fig. S5A). HAN1-Cas9-1 harbored a 2-bp nucleotides deletion (140 and 141 bp) in the HAN1 coding region, while HAN1-Cas9-4 carried a 16-bp deletion between nucleotides 296 and 311. Both alleles resulted in a frameshift in the HAN1 coding region and the early translation termination (Supplemental Fig. S5A).
To explore the involvement of HAN1 in rice disease resistance, we first inoculated the leaves of WT, HAN1-OX, and HAN1-Cas9 plants with M. oryzae spores. The HAN1-OX plants were more susceptible to M. oryzae in comparison with WT, whereas the HAN1-Cas9 plants displayed enhanced disease resistance, indicating that HAN1 negatively regulates rice blast resistance (Fig. 5, D to G; Supplemental Fig. S5B). The punch and leaf sheath inoculation assays also demonstrated that HAN1 plays a negative role in resisting M. oryzae invasion (Supplemental Fig. S5, C to E). We also challenged the OsANN1 transgenic lines with the bacterial blight pathogen PXO99. The results revealed that HAN1 negatively regulates rice bacterial blight resistance (Supplemental Fig. S5F). To further confirm the roles of HAN1 in rice immunity, we measured the ROS burst in the HAN1 transgenic plants upon chitin and flg22 treatments. The results showed that the HAN1-Cas9-1 plants had higher ROS burst levels than WT, but HAN1-OX showed very low burst levels (Fig. 5H). In addition, the transcription levels of defense genes were significantly higher in the HAN1-Cas9 plants, but much lower in the HAN1-OX plants (Fig. 5I). All these results confirmed the negative roles of HAN1 in rice immunity.
It is worth noting that HAN1 functions in plant growth and development, with a similar trend to OsANN1. Compared to WT, the HAN1-OX plants exhibited much longer length of second leaf sheath at seedlings stage and enhanced plant height at maturity stage, whereas the HAN1-Cas9 showed significant inhibition in second leaf sheath length and a semidwarf phenotype at maturity (Supplemental Fig. S6, A to F).
HAN1 genetically acts downstream of OsANN1
Since OsANN1 interacts with HAN1 and both proteins are involved in regulation of plant growth and disease resistance, we therefore tested their genetic interaction in rice. We first expressed HAN1 with a constitutive 35S promoter in the OsANN1-RNAi background to generate the OsANN1-RNAi/HAN1-OX plants (Supplemental Fig. S7A). Knocking-down of OsANN1 showed reduced second leaf sheath length and impaired disease response (Fig. 2A; Supplemental Fig. S3B); however, the OsANN1-RNAi/HAN1-OX seedlings exhibited almost the same second leaf length as the HAN1-OX seedlings (Fig. 6, A and B). The pathogen inoculation assays also revealed that the OsANN1-RNAi/HAN1-OX seedlings were more susceptible to M. oryzae, which is similar to HAN1-OX, indicating that overexpressing HAN1 in the OsANN1-RNAi plants markedly subverted their disease resistance (Fig. 6C; Supplemental Fig. S7B). These data demonstrate that OsANN1-mediated plant growth and disease resistance genetically depended on HAN1. To further confirm this notion, we transformed the HAN1-Cas9-1 plants with 35S-driven OsANN1 (HAN1-Cas9/OsANN1-OX) (Supplemental Fig. S7C). The HAN1-Cas9/OsANN1-OX plants exhibited similar second leaf sheath length and disease resistance as the HAN1-Cas9-1 plants, indicating that overexpressing OsANN1 had no significant effect on the HAN1-regulated plant growth and disease resistance (Fig. 6, D to F; Supplemental Fig. S7D). Taken together, our results suggest that HAN1 genetically acts downstream of OsANN1.
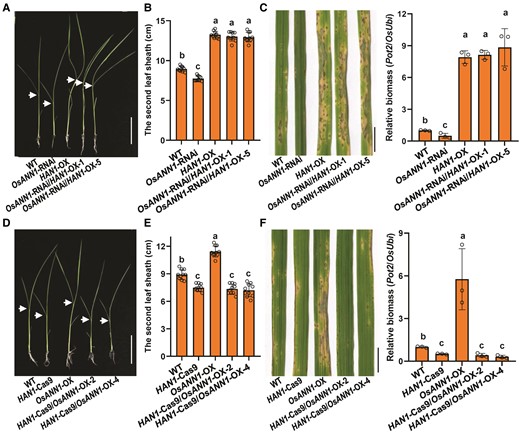
HAN1 genetically acts downstream of OsANN1. A) Phenotype of 10-d-old OsANN1-RNAi/HAN1-OX seedlings. Arrows indicate the lamina joints. Bar = 5 cm. B) The lengths of the second leaf sheaths in A). Values are means ± Sd (n = 20). Different letters indicate the significant differences based on 1-way ANOVA with Tukey's HSD test. C) Disease symptoms and relative fungal biomass of the OsANN1-RNAi/HAN1-OX lines after inoculated with M. oryzae conidia. Conidial suspensions were sprayed onto the leaf surface of 3-wk-old seedlings. Images were taken at the same time (5 dpi). Bar = 1 cm. The fungal biomass was determined by qPCR of M. oryzae Pot2 gene against rice OsUbi1 gene. Different letters indicate the significant differences based on 1-way ANOVA with Tukey's HSD test. Values are means ± Sd (n = 3 biological replicates). D) Phenotype of 10-d-old HAN1-Cas9/OsANN1-OX seedlings. Others are as in A). E) The lengths of second leaf sheaths in D). Others are as in B). F) Disease symptoms and relative fungal biomass of the HAN1-Cas9/OsANN1-OX lines after inoculated with M. oryzae conidia. Others are as in C).
OsANN1 enhances the HAN1 protein stability
To further explore the biological relevance of the interaction between OsANN1 and HAN1, we performed a cell-free degradation assay to investigate whether OsANN1 affects HAN1 stability. We noticed that OsANN1 stabilized HAN1. As shown in Fig. 7A, recombinant MBP-HAN1nsp was degraded rapidly in protein extracts from the OsANN1-RNAi seedlings than from the WT cell extracts, but much slowly in the OsANN1-OX cell extracts (Fig. 7A). Moreover, the 26S proteasome-specific inhibitor MG132 partially prevented the degradation of MBP-HAN1nsp in these cell extracts (Fig. 7A). These data suggest that OsANN1 could suppress the 26S proteasome-dependent degradation of HAN1. To further assess whether OsANN1 regulates HAN1 protein stability in planta, we expressed HAN1-FLAG in the protoplasts of WT, OsANN1-RNAi, and OsANN1-OX, respectively. In rice protoplasts transfected with the HAN1-FLAG plasmids, HAN1 protein abundance was decreased in OsANN1-RNAi, but increased in the OsANN1-OX plants compared with that in WT (Fig. 7B). We then checked the protein stability of HAN1 after the protein synthesis inhibitor cycloheximide (CHX) treatment in protoplasts. OsANN1-GFP and HAN1-FLAG or control vectors were cotransfected in protoplasts of WT, respectively. After CHX treatment, the protoplasts transfected with OsANN1-GFP exhibited substantially enhanced HAN1 protein stability compared with that transfected with GFP (Fig. 7C). These results implied that the interaction of OsANN1 with HAN1 enhanced the stability of HAN1 in planta.
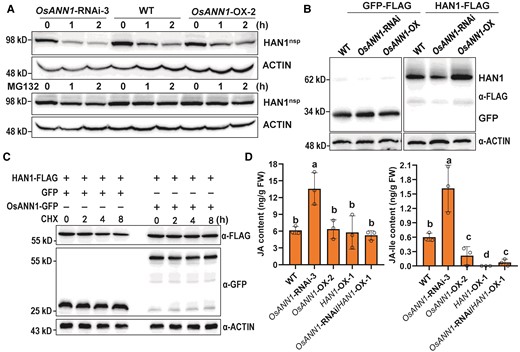
OsANN1 enhances the stability of HAN1. A) In vitro cell-free degradation assays showing that OsANN1 stabilizes HAN1. Total proteins extracted from 10-d-old WT, OsANN1-RNAi, and OsANN1-OX seedlings were incubated with recombinant MBP-HAN1nsp protein for indicated times. MBP-HAN1nsp was detected with the anti-MBP antibody. ACTIN served as a control. The western blots were captured using a chemiluminescent imaging system coupled to a CCD camera. B) HAN1 protein abundance in protoplasts of the WT, OsANN1-RNAi, and OsANN1-OX. The different rice protoplasts were transfected with HAN1-FLAG or GFP-FLAG constructs. Extracted proteins were immunoblotted with an anti-FLAG antibody and captured using a chemiluminescent imaging system. C) CHX treatment of WT protoplasts cotransfected with OsANN1-GFP and HAN1-FLAG. The rice protoplasts transfected with different constructs were treated with 4 μ M CHX for 0 to 8 h. Extracted proteins were immunoblotted with respective antibodies and captured using a chemiluminescent imaging system. D) The JA and JA-Ile content in different transgenic lines. Two-wk-old plant leaves were used to measure the JA and JA-Ile contents. FW, fresh weight. Different letters indicate the significant differences based on 1-way ANOVA with Tukey's HSD test. Values are means ± Sd (n = 3 biological replicates).
OsANN1 tunes down the JA-Ile accumulation
HAN1 has been previously reported to function as an oxidase to catalyze the conversion of biologically active JA-Ile to the inactive form 12OH-JA-Ile (Mao et al. 2019). Because OsANN1 interacts with HAN1 and enhances its stability in rice (Fig. 7, A to C), it is speculated that OsANN1 may contribute to the oxidization of biologically active form JA-Ile in plants. Thus, we measured JA and JA-Ile contents in leaves of OsANN1 transgenic lines, and found that the WT and OsANN1-OX lines had almost the same levels of JA, but JA content in OsANN1-RNAi was about 2-fold more than the above 2 lines (Fig. 7D). However, the JA-Ile content was reduced in OsANN1-OX, but increased in the OsANN1-RNAi plants when compared with WT (Fig. 7D). We also found that the OsANN1-RNAi/HAN1-OX-1 plants had extremely low level of JA-Ile. In addition, in the HAN1-OX plants, the JA-Ile content was not detectable (Fig. 7D), suggesting that HAN1 subverted the JA-Ile accumulation in OsANN1-RNAi plants. All these results suggest that OsANN1 tunes down the JA-Ile accumulation to undermine the disease resistance in plants.
Chitin attenuates the interaction of OsANN1 and HAN1
Because OsANN1 interacts with and stabilizes HAN1 to regulate the disease resistance, to find the link between this case and the pathogen infection, we first attempted to determine whether chitin treatment could influence the subcellular localization of OsANN1. However, the subcellular localization of OsANN1-GFP was almost unchanged after 30 min of chitin treatments in both N. benthamiana and rice protoplasts (Supplemental Fig. S8, A and B). These observations suggest that OsANN1 localization was not affected during PTI. Interestingly, we found that the interaction of OsANN1 and HAN1 was significantly weakened by chitin treatment in the split-luciferase assays, as revealed by the much weaker signal after chitin treatment than that of the control (Fig. 8, A and B). The results were subsequently validated by the Co-IP assays in rice protoplasts. As shown in Fig. 8C, the interaction of OsANN1 and HAN1 was markedly impaired after 30 min of chitin treatment. These data suggest that PTI activation facilitates the dissociation of OsANN1 from HAN1.
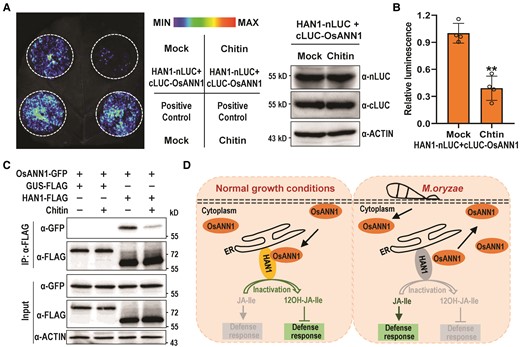
Chitin treatment dissociates OsANN1 from HAN1. A) The split luciferase assays showing that chitin treatment restrains the interaction of OsANN1 and HAN1. Nicotiana benthamiana leaves were coinfiltrated with 35S:HAN1-nLUC and 35S:cLUC-OsANN1; 200 μ M chitin was injected to the leaves for 30 min before the luminescence was recorded. The immunoblotting at the right shows the levels of respective proteins. B) Quantitative analysis of luminescence intensity for the comparison of Mock and chitin treatments in A). Values are means ± Sd (n = 4 biological replicates). **Significant differences from Mock at P < 0.01 (Student's t-test). C) The Co-IP assays showing that chitin treatment diminished the interaction of OsANN1 and HAN1. HAN1-FLAG and OsANN1-GFP were coexpressed in WT protoplasts. GUS-FLAG served as a negative control. After transfection for 14 h, the protoplasts were treated with 5 μ M chitin for 30 min. Co-IP was performed using anti-FLAG beads and the proteins were subjected to immunoblot analysis with anti-FLAG or anti-GFP antibody as indicated. ACTIN served as an internal control. The western blots were captured using a chemiluminescent imaging system coupled to a CCD camera. The experiment was repeated 3 times with similar results. D) A model showing the OsANN1-HAN1 module in regulating the disease response in rice. Without pathogen infection, OsANN1 interacts with HAN1 and enhances its stability, which promotes the transition of JA-Ile to the inactive form, thereby suppressing the immune response. Upon pathogen infection, PTI activation facilitates the dissociation of OsANN1 from HAN1, which subsequently leads to the HAN1 degradation. In this event, JA-Ile accumulated and then promoted the disease resistance in rice.
Discussion
Plant annexins are a type of proteins that are widespread in the Plant Kingdom (Mortimer et al. 2008; Laohavisit and Davies 2011). There are 8 and 10 genes encoding annexins in Arabidopsis and rice genomes, respectively (Jami et al. 2012). Previous studies have demonstrated that annexin proteins are heavily involved in regulating the abiotic stress responses in plants (Laohavisit and Davies 2011). Taking AtANN1 for example, it was reported to mediate several major abiotic stress responses, such as NaCl, ABA, cold, and heat stresses, which helps plant survival through various regulatory mechanisms (Lee et al. 2004; Konopka-Postupolska et al. 2009; Laohavisit et al. 2013; Wang et al. 2015; Liu et al. 2021). In this study, we analyzed the M. oryzae and PAMPs inducible OsANN1 and found that it is involved in the plant immune and disease responses. Biochemical and genetic evidence demonstrate that OsANN1 negatively regulates the ROS burst and defense genes expression in rice, which subverts plant tolerance to rice blast fungus. In addition, we revealed HAN1, a JA-Ile monooxygenase, that genetically acts downstream of OsANN1 and is stabilized by OsANN1. Thus, we propose that the OsANN1-HAN1 module fine-tunes the blast disease resistance in rice (Fig. 8C). Under normal growth conditions, OsANN1 interacts with HAN1 and enhances its protein's stability, which promotes the transition of the biologically active JA-Ile to the inactive form, thereby suppressing the immune response and promoting the plant growth in rice. Upon pathogen infection, PTI activation could facilitate the dissociation of OsANN1 from HAN1, which subsequently leads to the HAN1 degradation. In this event, JA-Ile accumulated in plants and then promoted the disease resistance in rice (Fig. 8D).
Several lines of evidence have shown that plant annexins possess peroxidase activity and regulate the production of ROS (Gorecka et al. 2005; Qiao et al. 2015; Gao et al. 2020; Zhang et al. 2021). AtANN1 regulates peroxide-stimulated Ca2+ influx to respond to the extracellular H2O2 in root epidermal cells (Richards et al. 2014). Besides, overexpression of AtANN1 resulted in hypersensitivity to drought and reduced accumulation of H2O2 in guard cells (Konopka-Postupolska et al. 2009). In rice, overexpression of OsANN1 improved the heat stress tolerance through eliminating excess ROS accumulation by increasing peroxidase activity (Qiao et al. 2015). OsANN4 regulated ROS and redox homeostasis in response to exogenous ABA (Zhang et al. 2021). In the present study, we showed that OsANN1 impairs the blast disease resistance by suppressing ROS burst and OsRbohD expression (Fig. 2). Similar to our observation, AtANN8 negatively regulates resistance to powdery mildew in Arabidopsis, where the resistance protein RPM8.1-triggered ROS accumulation was suppressed (Zhao et al. 2021). Interestingly, plant annexin knockout mutants are more sensitive to abiotic stress, and overexpression plants are more tolerant (Konopka-Postupolska et al. 2009; Qiao et al. 2015; Zhang et al. 2021). However, our results showed that knocking down/out of OsANN1 resulted in increased disease resistance to both fungal blast and bacterial blight, but overexpression of OsANN1 reduced disease resistance in the transgenic plants (Fig. 2, A to C; Supplemental Fig. S2, B to H). This may be partially due to the different roles of ROS in abiotic and biotic stresses. Although overaccumulation of ROS can lead to toxic effects on plants, ROS generation is a crucial defense signaling process to inhibit pathogen infection (Mittler 2002).
Phytohormone-mediated cellular signaling plays key roles in triggering plant immune response (Pieterse et al. 2012). Previously, we reported that M. oryzae infection activated ethylene biosynthesis, and the elevated ethylene levels increased disease resistance by facilitating ROS and phytoalexin production in rice (Yang et al. 2017). JAs are one of the major defense hormones, and their roles in defending herbivores and necrotrophic pathogens have been well demonstrated (Wang et al. 2019). In rice, exogenous application of JA increased rice blast resistance (Mei et al. 2006). Consistently, overexpressing OsAOS2, a key enzyme of JA biosynthesis in rice, increased JA levels, leading to the enhanced blast disease resistance (Mei et al. 2006). Our previous study indicates that ethylene promotes JA biosynthesis by activating OsOPR4 expression as well as producing phytoalexin to resist the blast fungus infection (Yang et al. 2017). In addition, we also found that a transcription activator OsbHLH6 regulates the dynamic changes of SA and JA signaling through its nucleo-cytosolic trafficking and the activation of the plant immune response (Meng et al. 2020). The roles of JA function in plant antiviral defense are controversial. Studies have indicated that JA positively regulates plant antiviral defense in Arabidopsis and rice (Wasternack and Song 2017). For example, a recent study reported that the accumulation of JA induced by rice stripe virus infection initiated the host defense network in rice (Yang et al. 2020). On the contrary, Oka et al. (2013) showed that JA treatment reduced the resistance to Tobacco mosaic virus in N. benthamiana. Here, our whole-genome transcriptome analysis suggests that OsANN1-mediated disease resistance requires JA response, ER and immunity-related processes (Fig. 3, C and D). Importantly, we found that OsANN1 interacted with HAN1 (Fig. 4), which facilitates the conversion of biologically active JA-Ile to the inactive form 12OH-JA-Ile in rice (Mao et al. 2019). Overexpression of HAN1 indeed resulted in the decreased JA-Ile accumulation, which subsequently impaired disease resistance (Figs 5D and 7D). Further, we were able to show that OsANN1-mediated plant susceptibility to M. oryzae genetically depended on HAN1 (Fig. 6). Because OsANN1 stabilizes HAN1, we concluded that OsANN1 reduced JA-Ile accumulation by stabilizing HAN1 in plants (Fig. 7, A to D). Thus, our study unravels an OsANN1-HAN1 module in rice blast disease resistance.
Notably, both OsANN1 and HAN1 affect rice seedlings growth. Overexpression of OsANN1 or HAN1 in rice increased the second leaf sheath length, but knocking them down or out significantly inhibited the second leaf sheath elongation (Supplemental Figs S3 and S6). Gibberellins (GAs) are very important factors in regulating the second leaf sheath and internode elongation in rice (Yamaguchi 2008). Generally, JAs inhibit plant growth and promote defense response, while GA often has the opposite roles (Huang et al. 2017). In fact, JAs-GA antagonism is an important regulatory mechanism for plants to balance growth and defense, although the underlying molecular processes remain to be discovered (Yang et al. 2012; Huang et al. 2017). De Vleesschauwer et al. (2016) reported that JA could restrict GA-induced growth promotion in rice seedlings and reverse GA-induced susceptibility to the bacterial leaf blight. However, another report showed that GA suppressed JA-mediated resistance against Meloidogyne graminicola, leading to the enhanced susceptibility to nematode infection (Yimer et al. 2018).
Although we found that OsANN1 enhances HAN1 protein's stability, the exact mechanism underlying how OsANN1 initiates this event remains to be further explored. Annexins have diverse functions, including the regulation of protein–protein interactions and the functions related to associations with cell membranes (Agrawal and Thelen 2006; Rohila et al. 2006). It is possible that OsANN1 might directly or indirectly collaborate with some other proteins to regulate HAN1's stability and function. We found that HAN1 could be degraded by the 26S proteasome (Fig. 7), although the E3 ligase participated in this ubiquitination has not been identified yet. Since OsANN1 interacted with HAN1 at its P450 domain (enzyme active center), we hypothesized that OsANN1 probably represses HAN1 ubiquitination through preventing the binding by E3 ligases. On the other hand, the regulatory role of OsANN1 in early signaling is not clear. Phosphorylation modification of annexin plays a role in stress signal transduction (Rohila et al. 2006). Several annexins are found to interact with various kinases, including MAPKK, Ste20-related protein kinase, and a calcium-dependent protein kinase OsCDPK24 (Rohila et al. 2006; Qiao et al. 2015; Zhang et al. 2021). In addition, AtANN4 is phosphorylated by SOS2 under salt stress (Ma et al. 2019). OST1 interacts with and phosphorylates AtANN1 to promote freezing tolerance by activating calcium signaling (Liu et al. 2021). Interestingly, chitin receptor CERK1 physically interacted with AtANN1 to regulate the plant salt stress response, although CERK1 kinase domain was not able to phosphorylate AtANN1 (Espinoza et al. 2017). These findings provide us some clues that whether rice immune receptors directly activate OsANN1 to transduce immune signals in cytosol. Future studies should investigate the potential regulators of the OsANN1-HAN1 module and the biochemical functions of OsANN1 in order to fully understand how OsANN1 is engaged in plant immune response.
Materials and methods
Plant and fungal strain growth conditions
Rice (O. sativa subsp. japonica cv Nipponbare) was used as the wild type (WT). The OsANN1-RNAi lines were obtained from Qiao et al. (2015). The OsANN1-Cas9 mutants were obtained from Wimibio (China). The plants were grown at 28 °C with a 16-h/8-h light/dark photoperiod and 75% relative humidity. Magnaporthe oryzae strain Zhong-84-10 was grown on oatmeal agar medium (30 g L−1 oat, 0.6 g L−1 calcium carbonate, and 16 g L−1 agar) for about 10 d. Then the conidia were induced under white light for 3 d after removing the aerial mycelium from the plate.
Plant transformation
For generating the overexpression transgenic plants, the coding sequences of OsANN1 and HAN1 were introduced into the binary vector pCAMBIA2300 with the 35S promoter, respectively. For constructing HAN1-CRISPR/Cas9 plants, the gRNA expression cassette was inserted into the CRISPR/Cas9-MTmono binary vector. Then all these vectors were introduced into Agrobacterium tumefaciens strain EHA105 through electroporation, and the resulting strains were used to transform the corresponding rice calli.
RNA extraction and RT-qPCR assay
Rice leaves or suspension cells were collected at indicated time points. Total RNA was extracted using TRIZOL reagent (Invitrogen, USA). The cDNA synthesis was performed using HiScript QRT SuperMix according to the manufacturer's instructions (Vazyme, China). ChamQ SYBR qPCR Master Mix (Vazyme) was used for RT-qPCR analysis with CFX96TM Real-time System (Bio-RAD, USA). The primers used are listed in Supplemental Table S1.
Plant infection assay
The plant infection assay was performed as described previously (Yang et al. 2017). For spray inoculation, conidial suspensions were adjusted to 2 × 105 conidia mL −1 in 0.02% (v/v) Tween-20. Then the suspension was sprayed onto the rice leaf surface of 3-wk-old rice seedlings in all directions uniformly. Subsequently, the plants were placed in growth chamber at 28 °C without light for 24 h, then switched back to normal growth conditions. For punch inoculation, a 10 μL volume spore suspension was applied to the punched leaves. Photographs were taken at 5 to 7 d after inoculation. The fungal biomass was determined by qPCR using specific primers for Pot2 gene of M. oryzae and normalized to the reference rice gene OsUbi1. The primers used are listed in Supplemental Table S1. For rice sheath penetration assays, the conidial suspensions (1 × 105 conidia mL−1 in 0.02% [v/v] Tween-20) were injected into the seedling inner leaf sheaths by a syringe, and then the inoculated leaf sheaths were incubated under the same condition as the spray assay. At 24 hpi, the leaf sheaths were examined under a microscope to observe the fungal development in rice tissues.
ROS burst assay
The ROS burst assay was performed following the method described by Yang et al. (2021). Briefly, the rice suspensions cells were incubated in preincubation medium [3% (w/v) sucrose, 10 mM MES in 5% (v/v) culture medium, pH 5.8] for 4 h. Then the medium was replaced with reaction buffer [20 μ M L-012 (Wako Chemicals, USA), 10 mg mL−1 horseradish peroxidase (Sigma, USA), 100 nM flg22 or 2 μg mL−1 chitin elicitors]. The luminescence was recorded by a Centro XS3 LB 960 Luminometer (Berthold Technologies, Germany).
Library preparation and bioinformatics analysis
For RNA-seq analysis, 2-wk-old rice seedlings (WT and OsANN1-OX) before and 24 h post M. oryzae inoculation were used to extract total RNA from the third leaves using TRIZOL reagent. Library preparation and sequencing process were described previously (Yang et al. 2017; Meng et al. 2020). Briefly, RNA integrity was evaluated using a Bioanalyzer 2100 (Agilent Technologies, USA). The libraries were constructed and sequenced on an Illumina NovaSeq 6000 at Novegene (Beijing) and 150 bp paired-end reads were generated. Raw reads of fastq format were firstly processed by fastp to generate clean reads (Chen et al. 2018). Then clean reads were mapped to rice reference genome MSU7.0 using HISAT2 (v2.2.1) with default parameters, and FPKM (Fragments Per Kilobase of exon model per Million mapped fragments) of each gene was calculated by StringTie (v2.2.1) with default parameters (Pertea et al. 2015). The differential-expression analysis was performed using DESeq2 (1.37.6) with the criteria of adjust P value <0.01 and absolute log2-fold change >1. Finally, GO analysis of DEGs was performed using clusterProfiler (v4.4.4), and terms with P value <0.05 were supposed to be enriched (Yu et al. 2012).
Y2H assays
The Y2H assay was carried out following the manufacturer's instructions (Matchmaker, Clontech, USA). OsANN1 or OsANN127–314 coding sequence was inserted into pGADT7 vector, and HAN1 or different fragments of HAN1 coding sequence was cloned into pGBKT7 vector. pGADT7-T and pGBKT7-53 served as positive control, and pGADT7-T and pGBKT7-Lam served as negative control. Yeast strain AH109 transformed with the corresponding vectors was cultured on synthetic dropout media lacking leucine and tryptophan (SD/-Leu-Trp). Then the single yeast colonies were screened for interaction on the synthetic dropout media lacking leucine, tryptophan and Histidine (SD/-Leu-Trp-His) with serial dilution.
GST pull-down assay
The coding sequence of OsANN1 was cloned into pGEX-4T-1 to generate GST-OsANN1. The sequence of HAN1 without signaling peptide (removal of signaling peptide from amino acids 1 to 25) was cloned into pMAL-C4X to generate MBP-HAN1nsp. Protein purification and GST pull-down assays were performed as described previously (Luo et al. 2020).
Co-IP assays
The Co-IP assays in the N. benthamiana and rice protoplasts were performed as described previously (Luo et al. 2020). Taking the assay in N. benthamiana as an example, the leaves that expressed the corresponding proteins were harvested at 48 hpi, then the leaves were ground in liquid nitrogen and extracted in extraction buffer containing 50 mM Tris–HCl (pH 7.5), 150 mM NaCl, 0.1% (v/v) Triton X-100, 0.2% (v/v) Nonidet P-40, 1 mM DTT, and 1 × complete protease inhibitor (Roche, Switzerland). The homogenate was centrifuged at 15,000 × g for 20 min. Anti-FLAG antibody-conjugated agarose beads (Sigma) were added to the supernatant. After incubation at 4 °C on an end-over-end shaker for 2 h, the beads were spun down at 1,500 g for 2 min and washed with extraction buffer at least 5 times. The bound proteins were eluted by 1.5× Laemmli buffer and resolved by 12% (w/v) SDS-PAGE and then subjected to immunoblot analysis.
Split-Luciferase complementation assay
The Split-Luciferase complementation assay was performed as described by Meng et al. (2020). Agrobacterium tumefaciens strains carrying the corresponding nLUC and cLUC vectors were mixed and infiltrated into 4-wk-old N. benthamiana leaves using a 1-mL needleless syringe. After 2 d, N. benthamiana leaves were rubbed with 0.5 mM luciferin and kept in the dark for 5 min to quench the fluorescence. Then the luminescence was recorded by an in vivo Plant Imaging System with a CCD camera (NightShade LB985, Berthold technologies).
BiFC assay
The coding sequences of HAN1 and OsANN1 were cloned into pSAT1-nYFP and pSAT1-cYFP vectors, respectively. The A. tumefaciens strain C58C1 carrying the corresponding vectors was mixed and infiltrated into the leaves of 3-wk-old N. benthamiana leaves. The YFP fluorescence was observed 48 h later using a confocal laser scanning microscope (Leica Model TCS SP8, Germany) using 514 nm excitation radiation and observing 525 to 545 nm of emission. The mCherry fluorescence was also observed using 587 nm excitation radiation and observing 590 to 610 nm of emission.
Cell-free protein degradation assay
The cell-free protein degradation assay of HAN1 was performed as described previously (Ding et al. 2015). Total proteins were extracted from the leaves of 10-d-old rice plants in extraction buffer. Equal amounts of total proteins from the WT, OsANN1-RNAi-3, and OsANN1-OX-2 plants were incubated with recombinant MBP-HAN1nsp protein for the time indicated. The reactions were stopped by adding 1.5× Laemmli buffer and separated on a 10% (v/v) SDS-PAGE gel. HAN1nsp protein was detected with anti-MBP antibody.
Rice protoplast transformation
For the preparation of rice protoplasts, the rice seeds were geminated and grown for 10 d and tissues from the stems and sheaths of seedlings were used. The protoplast isolation and polyethylene glycol-mediated transformation procedures were carried out as described previously (Meng et al. 2020). Total proteins were extracted from rice protoplasts expressing HAN1-FLAG or GFP-FLAG and detected with anti-FLAG antibody (Sigma).
Measurement of JA and JA-Ile HPLC-TOF/MS
The leaves (100 mg each) were freeze dried and homogenized in liquid nitrogen and extracted with 1 mL 90% (v/v) aqueous methanol containing 0.1% (v/v) formic acid. Then the samples were subjected to liquid chromatography–tandem mass spectrometry (LC–MS) analysis with Agilent 6520B quadrupole time-of-flight mass spectrometry (Q-TOF MS/MS) equipped with a dual ESI electrospray ion source (Agilent Technologies). Six nanograms of d5-JA were used as the internal standard, and JA-Ile was determined using external standard method. The JA and JA-Ile levels were determined with the mass-to-charge ratio combinations (precursor/product ions) of 214.1497/61.0265 for d5-JA, 209.1183/59.0143 for JA, and 322.2023/130.0866 for JA-Ile. Experiments were performed with 3 independent biological replicates.
Phylogenetic analyses
The amino acid sequences of the OsANN1 and HAN1 homologous proteins were downloaded from the UniProt website (https://www.uniprot.org/). Sequence alignment was performed with ClustalW. A neighbor-joining method implemented in MEGA7.0 was used to generate the phylogenetic tree. The bootstrap values indicated at the nodes in the phylogenetic tree are based on 1,000 replications.
Accession numbers
Sequence data from this article can be found in the GenBank/EMBL data libraries under accession numbers: OsANN1 (LOC_Os02g51750), HAN1 (LOC_Os11g29290), PAL (LOC_Os02g41680), OsRbohD (LOC_Os05g38980), OsPR10 (LOC_Os03g18850), PR10 (LOC_Os12g36880), OsActin2 (LOC_Os10g36650), Pot2 (MK608664), and OsUbi1 (LOC_Os03g13170).
Acknowledgments
We thank Prof. Zhengge Zhu at Hebei Normal University for kindly providing the seeds of OsANN1-RNAi lines. We also thank Dr Xujun Chen and Dr Linlu Qi at China Agricultural University for technical assistance on the use of HPLC-TOF/MS.
Author contributions
C.Y., Q.Q.Z., and R.L. conceived and designed the project; Q.Q.Z., R.L., Q.Z.Z., J.Y., and C.Y. performed the experiments; F.W.M. created the rice materials; C.Y. and R.L. wrote the article, J.L revised, and edited the manuscript.
Supplemental data
The following materials are available in the online version of this article.
Supplemental Figure S1. A phylogenetic analysis of the OsANN1 homologs in various plants.
Supplemental Figure S2. OsANN1 negatively regulates the fungal blast and bacterial blight diseases resistance in rice.
Supplemental Figure S3. OsANN1 influences the rice growth.
Supplemental Figure S4. Protein structure and subcellular localization of HAN1.
Supplemental Figure S5. HAN1 is a negative regulator of disease resistance in rice.
Supplemental Figure S6. HAN1 functions in plant growth and development.
Supplemental Figure S7. OsANN1-mediated disease resistance genetically depends on HAN1.
Supplemental Figure S8. Chitin treatment could not influence the subcellular localization of OsANN1.
Supplemental Table S1. Primers used for this study.
Supplemental Data Set 1. List of the DEGs in WT and OsANN1-OX 24 hpi vs 0 hpi.
Supplemental Data Set 2. List of the DEGs of the specifically enriched GO terms in OsANN1-OX in Fig. 3D.
Funding
This study was supported by the Natural Science Foundation of China (31972257, 32070290) and China Agricultural University.
Data availability
The authors confirm that all experimental data are availableand accessible via the main text and/or the supplemental data.
References
Author notes
These authors contributed equally to the work.
The author responsible for distribution of materials integral to the findings presented in this article in accordance with the policy described in the Instructions for Authors (https://dbpia.nl.go.kr/plphys/pages/General-Instructions) is Chao Yang ([email protected]).
Conflict of interest statement. The authors declare no conflict of interest.