-
PDF
- Split View
-
Views
-
Cite
Cite
Brendan M O’Leary, Andrew P Scafaro, Larry M York, High-throughput, dynamic, multi-dimensional: an expanding repertoire of plant respiration measurements, Plant Physiology, Volume 191, Issue 4, April 2023, Pages 2070–2083, https://doi.org/10.1093/plphys/kiac580
- Share Icon Share
Abstract
A recent burst of technological innovation and adaptation has greatly improved our ability to capture respiration rate data from plant sources. At the tissue level, several independent respiration measurement options are now available, each with distinct advantages and suitability, including high-throughput sampling capacity. These advancements facilitate the inclusion of respiration rate data into large-scale biological studies such as genetic screens, ecological surveys, crop breeding trials, and multi-omics molecular studies. As a result, our understanding of the correlations of respiration with other biological and biochemical measurements is rapidly increasing. Difficult questions persist concerning the interpretation and utilization of respiration data; concepts such as allocation of respiration to growth versus maintenance, the unnecessary or inefficient use of carbon and energy by respiration, and predictions of future respiration rates in response to environmental change are all insufficiently grounded in empirical data. However, we emphasize that new experimental designs involving novel combinations of respiration rate data with other measurements will flesh-out our current theories of respiration. Furthermore, dynamic recordings of respiration rate, which have long been used at the scale of mitochondria, are increasingly being used at larger scales of size and time to reflect processes of cellular signal transduction and physiological response to the environment. We also highlight how respiratory methods are being better adapted to different plant tissues including roots and seeds, which have been somewhat neglected historically.
Introduction
The study of plant respiration has for centuries investigated non-photosynthetic gas exchange in plants: the consumption of oxygen and release of carbon dioxide. One may ask what the purpose of plant respiration research is today and why there is a continued need for methodological improvements. After all, the principal function of cellular respiration (to generate usable chemical energy) and the foundational biochemical mechanisms by which this occurs have been settled. But plant respiration is by no means a static rate, nor does it perform a singular energy-generating function, as cellular redox balancing and generation of carbon skeletons for biosynthesis also involve respiratory fluxes (O’Leary et al., 2019). Indeed, the major goals of respiration research have changed over time and new instrumental requirements and challenges have arisen. Research focus has long since shifted from understanding the phenomenon of respiration per se to understanding differences in respiration among species, genotypes, tissues, and environments. One major overarching theme is the attempt to understand and ultimately model the variable respiratory fluxes in various plant tissues and whole plants. There is a need to predict plant net CO2 uptake and release as it pertains to plant growth and changes to the environment, such as the rise in atmospheric CO2 concentrations (Huntingford et al., 2017; Collalti et al., 2020). There is also the potential to further improve agricultural yield by optimizing respiratory metabolism in crops, avoiding unnecessary loss of assimilated carbon (Amthor et al., 2019). Both these goals benefit from high-throughput respiration measurements that can be combined and correlated with large multi-dimensional datasets. Another research theme is that many regulatory mechanisms and genetic elements controlling respiratory flux remain to be discovered or clarified, particularly at the whole cell or tissue level (van Dongen et al., 2011). A change in respiratory rate by itself is often difficult to interpret—why did it occur? Rather, clear interpretation requires parallel assays of respiratory gas-exchange measurements with other molecular measurements such as additional fluxes or metabolite levels. Ideally, these parallel measurements aim to observe system dynamics (i.e. time course observations of change) by being at least semi-continuous in nature. A third and innovative research theme considers whether respiration measurements can be diagnostic tools to estimate other physiological processes such as seed germination vigor or infection resistance, processes that require respiration measurements to be dynamic in nature and adapted to multiple tissues.
High-throughput capabilities allow the incorporation of plant respiration rate measurements into large-scale multi-dimensional studies within plant biology and agriculture.
Genetic and environmental influences on respiration rate are complex but becoming better defined by higher-throughput correlative studies.
The molecular and genetic mechanisms underpinning respiratory variation can be investigated through new multiplexed measures of cellular energy status based on fluorescent probes.
Respiration measurements in sink tissues like seeds and roots have become more feasible but remain less studied than respiration in leaves.
Shared protocols and greater community engagement allow new methods to be deployed quickly around the world.
There are currently a number of methods to measure respiratory gas fluxes in plants. In Table 1, we briefly summarize the advantages and disadvantages of the most common of these analytical methods. Largely due to differences in temporal resolution and physical sample size, each technique tends to be more suitable for particular fields of work (Figure 1). Ideally, both oxygen and carbon dioxide exchange would be measured simultaneously, as they relate to different stages of respiratory metabolism, but in practice, this is rarely the case. In fact, respiratory quotients (the CO2:O2 flux ratio; RQ) are often difficult to obtain with a high degree of confidence because of the separate analytical techniques typically required (Scafaro et al., 2017). The choice of CO2 versus O2-based measurements is usually determined by instrument availability and capability. All respiratory gas-exchange measurements are subject to potential interference from non-respiratory reactions that complicate data interpretation if not explicitly considered (e.g. CO2 reassimilation, or non-mitochondrial oxidase reactions; O’Leary et al., 2019).
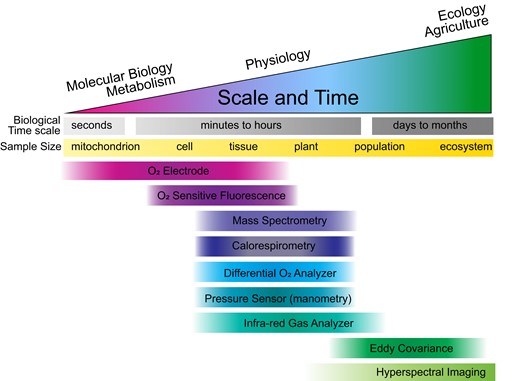
Available methods for quantifying respiration rates are generally suitable for biological studies at a particular scale: both time and size. The time scales shown are very approximate but indicate that techniques are typically used to observe respiratory variation at different intervals. Infrared gas analyzers (IRGA) are utilized across the greatest scope of time and size, and in fact, eddy covariance relies on IRGA measurements of CO2 but calculates respiratory flux by a different method.
Method . | Gas Analyzed . | High-Throughput . | Dynamic . | Other Experimental Advantages/Disadvantages . | Protocol or Theory References . |
---|---|---|---|---|---|
Clark Type O2 Electrode | O2 | No | Yes | Experimental Advantage − Liquid phase measurements are suitable for mitochondrial activity assays because of rapid dynamic responses combined with easy, mid-assay addition of chemical effectors (e.g. inhibitors, ADP, substrates) − Can be combined with a light source to also measure photosynthetic O2 release from tissues Experimental Disadvantage −Gas-phase assays are possible but are less sensitive compared to IRGA and thus infrequently used for respiration − Short duration: aqueous O2 depletion is on the order of minutes, limiting observations of dynamics in tissues − During tissue measurements, the requirement for submersion and rapid stirring may have unintended effects | (Clark et al., 1953; Jacoby et al., 2015) |
O2 Luminescence Quenching | O2 | Yes | Yes | Experimental Advantage − Long duration: gas-phase measurements lasting several days are possible, suitable for observing dynamic responses in tissues that involve changes in gene expression, acclimation or development. − Adaptable: the fluorescent dyes can be placed in any type of sealed container and measurements conducted in gas or liquid phase − Combinable with measurements of other fluorescent metabolite probes in multi-parameter plate reader assays Experimental Disadvantage − Mostly employed in a closed system where buildup of gaseous H2O, CO2 and ethylene can occur; but Schilling et al. 2015 introduce an open/closed hybrid system. −Gas-phase measurements are sensitive to temperature and physical disturbances, increasing background noise. | (Tyystjärvi et al., 1998; Schilling et al., 2015; Sew et al., 2015; Scafaro et al., 2017; Wagner et al., 2019; O’Leary et al., 2022) |
Mass spectrometry | O2 and CO2 | No | Yes | Experimental Advantage: − Multiple gases can be analyzed simultaneously − Allows isotope discrimination measurements needed for certain determinations of day respiration and measurement of alternative oxidase versus cytochrome pathway flux − Highly sensitive instruments can enable detection of minimal flux rates − Online versus offline versions offer adaptable sample collection regimes Experimental Disadvantage − Expensive to maintain and run − Can be technically challenging and specialized skills required | (Ribas-Carbo et al., 2005; Beckmann et al., 2009; Cheah et al., 2014) |
Differential O2 Analyzer | O2 | No | Yes | Experimental Advantage − Precise quantification of O2 uptake by respiration in an open system − Can be paired with IRGA Experimental Disadvantage − Prone to leaks from cuvette or chamber creating error − Difficult to calibrate − Short lifespan of oxygen fuel cell sensors | (Hunt, 2003) |
Infrared Gas Analyzer | CO2 | Partly | Yes | Experimental Advantage − Relatively fast, straightforward measurement process allows higher sample throughputs and scalable data collection − Often designed as portable, field use instruments − Adaptable technology: open and closed systems; large range of sample (chamber) sizes; − Can be combined with H2O measurements for stomatal analysis or light and dark measurement combinations Experimental Disadvantage − Prone to leaks from cuvette or chamber creating error | (Flexas et al., 2006; Fonseca et al., 2021) |
Eddy Covariance | CO2 | No | Yes | Experimental Advantage − Large spatial and temporal scale of measurement − Non-destructive, non-intrusive Experimental Disadvantage − Specific environmental and geographical conditions must be met to satisfy assumptions within the calculations | (Goulden et al., 1996; Baldocchi, 2003) |
Hyperspectral Imaging | n/a | Yes | No | Experimental Advantage − Largest spatial and temporal scale (can be implemented from satellites and aircraft) − Non-destructive, non-intrusive Experimental Disadvantage − An indirect measurement: dependent upon a large training data set that requires direct respiratory measurements to create a predictive model; therefore, only as reliable as the training data − Large data storage required − Images must be captured in the light, but training respiration rate data typically measured in the dark | (Coast et al., 2019) |
Method . | Gas Analyzed . | High-Throughput . | Dynamic . | Other Experimental Advantages/Disadvantages . | Protocol or Theory References . |
---|---|---|---|---|---|
Clark Type O2 Electrode | O2 | No | Yes | Experimental Advantage − Liquid phase measurements are suitable for mitochondrial activity assays because of rapid dynamic responses combined with easy, mid-assay addition of chemical effectors (e.g. inhibitors, ADP, substrates) − Can be combined with a light source to also measure photosynthetic O2 release from tissues Experimental Disadvantage −Gas-phase assays are possible but are less sensitive compared to IRGA and thus infrequently used for respiration − Short duration: aqueous O2 depletion is on the order of minutes, limiting observations of dynamics in tissues − During tissue measurements, the requirement for submersion and rapid stirring may have unintended effects | (Clark et al., 1953; Jacoby et al., 2015) |
O2 Luminescence Quenching | O2 | Yes | Yes | Experimental Advantage − Long duration: gas-phase measurements lasting several days are possible, suitable for observing dynamic responses in tissues that involve changes in gene expression, acclimation or development. − Adaptable: the fluorescent dyes can be placed in any type of sealed container and measurements conducted in gas or liquid phase − Combinable with measurements of other fluorescent metabolite probes in multi-parameter plate reader assays Experimental Disadvantage − Mostly employed in a closed system where buildup of gaseous H2O, CO2 and ethylene can occur; but Schilling et al. 2015 introduce an open/closed hybrid system. −Gas-phase measurements are sensitive to temperature and physical disturbances, increasing background noise. | (Tyystjärvi et al., 1998; Schilling et al., 2015; Sew et al., 2015; Scafaro et al., 2017; Wagner et al., 2019; O’Leary et al., 2022) |
Mass spectrometry | O2 and CO2 | No | Yes | Experimental Advantage: − Multiple gases can be analyzed simultaneously − Allows isotope discrimination measurements needed for certain determinations of day respiration and measurement of alternative oxidase versus cytochrome pathway flux − Highly sensitive instruments can enable detection of minimal flux rates − Online versus offline versions offer adaptable sample collection regimes Experimental Disadvantage − Expensive to maintain and run − Can be technically challenging and specialized skills required | (Ribas-Carbo et al., 2005; Beckmann et al., 2009; Cheah et al., 2014) |
Differential O2 Analyzer | O2 | No | Yes | Experimental Advantage − Precise quantification of O2 uptake by respiration in an open system − Can be paired with IRGA Experimental Disadvantage − Prone to leaks from cuvette or chamber creating error − Difficult to calibrate − Short lifespan of oxygen fuel cell sensors | (Hunt, 2003) |
Infrared Gas Analyzer | CO2 | Partly | Yes | Experimental Advantage − Relatively fast, straightforward measurement process allows higher sample throughputs and scalable data collection − Often designed as portable, field use instruments − Adaptable technology: open and closed systems; large range of sample (chamber) sizes; − Can be combined with H2O measurements for stomatal analysis or light and dark measurement combinations Experimental Disadvantage − Prone to leaks from cuvette or chamber creating error | (Flexas et al., 2006; Fonseca et al., 2021) |
Eddy Covariance | CO2 | No | Yes | Experimental Advantage − Large spatial and temporal scale of measurement − Non-destructive, non-intrusive Experimental Disadvantage − Specific environmental and geographical conditions must be met to satisfy assumptions within the calculations | (Goulden et al., 1996; Baldocchi, 2003) |
Hyperspectral Imaging | n/a | Yes | No | Experimental Advantage − Largest spatial and temporal scale (can be implemented from satellites and aircraft) − Non-destructive, non-intrusive Experimental Disadvantage − An indirect measurement: dependent upon a large training data set that requires direct respiratory measurements to create a predictive model; therefore, only as reliable as the training data − Large data storage required − Images must be captured in the light, but training respiration rate data typically measured in the dark | (Coast et al., 2019) |
Dynamic refers to whether a technique is amenable to continuous measurement, over an appropriate timescale, to observe how rates change.
Method . | Gas Analyzed . | High-Throughput . | Dynamic . | Other Experimental Advantages/Disadvantages . | Protocol or Theory References . |
---|---|---|---|---|---|
Clark Type O2 Electrode | O2 | No | Yes | Experimental Advantage − Liquid phase measurements are suitable for mitochondrial activity assays because of rapid dynamic responses combined with easy, mid-assay addition of chemical effectors (e.g. inhibitors, ADP, substrates) − Can be combined with a light source to also measure photosynthetic O2 release from tissues Experimental Disadvantage −Gas-phase assays are possible but are less sensitive compared to IRGA and thus infrequently used for respiration − Short duration: aqueous O2 depletion is on the order of minutes, limiting observations of dynamics in tissues − During tissue measurements, the requirement for submersion and rapid stirring may have unintended effects | (Clark et al., 1953; Jacoby et al., 2015) |
O2 Luminescence Quenching | O2 | Yes | Yes | Experimental Advantage − Long duration: gas-phase measurements lasting several days are possible, suitable for observing dynamic responses in tissues that involve changes in gene expression, acclimation or development. − Adaptable: the fluorescent dyes can be placed in any type of sealed container and measurements conducted in gas or liquid phase − Combinable with measurements of other fluorescent metabolite probes in multi-parameter plate reader assays Experimental Disadvantage − Mostly employed in a closed system where buildup of gaseous H2O, CO2 and ethylene can occur; but Schilling et al. 2015 introduce an open/closed hybrid system. −Gas-phase measurements are sensitive to temperature and physical disturbances, increasing background noise. | (Tyystjärvi et al., 1998; Schilling et al., 2015; Sew et al., 2015; Scafaro et al., 2017; Wagner et al., 2019; O’Leary et al., 2022) |
Mass spectrometry | O2 and CO2 | No | Yes | Experimental Advantage: − Multiple gases can be analyzed simultaneously − Allows isotope discrimination measurements needed for certain determinations of day respiration and measurement of alternative oxidase versus cytochrome pathway flux − Highly sensitive instruments can enable detection of minimal flux rates − Online versus offline versions offer adaptable sample collection regimes Experimental Disadvantage − Expensive to maintain and run − Can be technically challenging and specialized skills required | (Ribas-Carbo et al., 2005; Beckmann et al., 2009; Cheah et al., 2014) |
Differential O2 Analyzer | O2 | No | Yes | Experimental Advantage − Precise quantification of O2 uptake by respiration in an open system − Can be paired with IRGA Experimental Disadvantage − Prone to leaks from cuvette or chamber creating error − Difficult to calibrate − Short lifespan of oxygen fuel cell sensors | (Hunt, 2003) |
Infrared Gas Analyzer | CO2 | Partly | Yes | Experimental Advantage − Relatively fast, straightforward measurement process allows higher sample throughputs and scalable data collection − Often designed as portable, field use instruments − Adaptable technology: open and closed systems; large range of sample (chamber) sizes; − Can be combined with H2O measurements for stomatal analysis or light and dark measurement combinations Experimental Disadvantage − Prone to leaks from cuvette or chamber creating error | (Flexas et al., 2006; Fonseca et al., 2021) |
Eddy Covariance | CO2 | No | Yes | Experimental Advantage − Large spatial and temporal scale of measurement − Non-destructive, non-intrusive Experimental Disadvantage − Specific environmental and geographical conditions must be met to satisfy assumptions within the calculations | (Goulden et al., 1996; Baldocchi, 2003) |
Hyperspectral Imaging | n/a | Yes | No | Experimental Advantage − Largest spatial and temporal scale (can be implemented from satellites and aircraft) − Non-destructive, non-intrusive Experimental Disadvantage − An indirect measurement: dependent upon a large training data set that requires direct respiratory measurements to create a predictive model; therefore, only as reliable as the training data − Large data storage required − Images must be captured in the light, but training respiration rate data typically measured in the dark | (Coast et al., 2019) |
Method . | Gas Analyzed . | High-Throughput . | Dynamic . | Other Experimental Advantages/Disadvantages . | Protocol or Theory References . |
---|---|---|---|---|---|
Clark Type O2 Electrode | O2 | No | Yes | Experimental Advantage − Liquid phase measurements are suitable for mitochondrial activity assays because of rapid dynamic responses combined with easy, mid-assay addition of chemical effectors (e.g. inhibitors, ADP, substrates) − Can be combined with a light source to also measure photosynthetic O2 release from tissues Experimental Disadvantage −Gas-phase assays are possible but are less sensitive compared to IRGA and thus infrequently used for respiration − Short duration: aqueous O2 depletion is on the order of minutes, limiting observations of dynamics in tissues − During tissue measurements, the requirement for submersion and rapid stirring may have unintended effects | (Clark et al., 1953; Jacoby et al., 2015) |
O2 Luminescence Quenching | O2 | Yes | Yes | Experimental Advantage − Long duration: gas-phase measurements lasting several days are possible, suitable for observing dynamic responses in tissues that involve changes in gene expression, acclimation or development. − Adaptable: the fluorescent dyes can be placed in any type of sealed container and measurements conducted in gas or liquid phase − Combinable with measurements of other fluorescent metabolite probes in multi-parameter plate reader assays Experimental Disadvantage − Mostly employed in a closed system where buildup of gaseous H2O, CO2 and ethylene can occur; but Schilling et al. 2015 introduce an open/closed hybrid system. −Gas-phase measurements are sensitive to temperature and physical disturbances, increasing background noise. | (Tyystjärvi et al., 1998; Schilling et al., 2015; Sew et al., 2015; Scafaro et al., 2017; Wagner et al., 2019; O’Leary et al., 2022) |
Mass spectrometry | O2 and CO2 | No | Yes | Experimental Advantage: − Multiple gases can be analyzed simultaneously − Allows isotope discrimination measurements needed for certain determinations of day respiration and measurement of alternative oxidase versus cytochrome pathway flux − Highly sensitive instruments can enable detection of minimal flux rates − Online versus offline versions offer adaptable sample collection regimes Experimental Disadvantage − Expensive to maintain and run − Can be technically challenging and specialized skills required | (Ribas-Carbo et al., 2005; Beckmann et al., 2009; Cheah et al., 2014) |
Differential O2 Analyzer | O2 | No | Yes | Experimental Advantage − Precise quantification of O2 uptake by respiration in an open system − Can be paired with IRGA Experimental Disadvantage − Prone to leaks from cuvette or chamber creating error − Difficult to calibrate − Short lifespan of oxygen fuel cell sensors | (Hunt, 2003) |
Infrared Gas Analyzer | CO2 | Partly | Yes | Experimental Advantage − Relatively fast, straightforward measurement process allows higher sample throughputs and scalable data collection − Often designed as portable, field use instruments − Adaptable technology: open and closed systems; large range of sample (chamber) sizes; − Can be combined with H2O measurements for stomatal analysis or light and dark measurement combinations Experimental Disadvantage − Prone to leaks from cuvette or chamber creating error | (Flexas et al., 2006; Fonseca et al., 2021) |
Eddy Covariance | CO2 | No | Yes | Experimental Advantage − Large spatial and temporal scale of measurement − Non-destructive, non-intrusive Experimental Disadvantage − Specific environmental and geographical conditions must be met to satisfy assumptions within the calculations | (Goulden et al., 1996; Baldocchi, 2003) |
Hyperspectral Imaging | n/a | Yes | No | Experimental Advantage − Largest spatial and temporal scale (can be implemented from satellites and aircraft) − Non-destructive, non-intrusive Experimental Disadvantage − An indirect measurement: dependent upon a large training data set that requires direct respiratory measurements to create a predictive model; therefore, only as reliable as the training data − Large data storage required − Images must be captured in the light, but training respiration rate data typically measured in the dark | (Coast et al., 2019) |
Dynamic refers to whether a technique is amenable to continuous measurement, over an appropriate timescale, to observe how rates change.
Many respiratory measurement techniques are derived from three fundamental analytical mechanisms: infrared gas analysis (IRGA) of CO2 (e.g. Figure 2, B and C), electrochemical detection of O2 with electrodes (e.g. Figure 2A), and O2-sensitive luminescence quenching (e.g. Figure 2D). The theory of these techniques and important technical aspects of their implementation into open and closed respiratory measurement devices have been previously reviewed (Hunt, 2003; Quaranta et al., 2012). These techniques supplanted the much earlier dependence upon manometry for gas-exchange measurements, but it too still has unique analytical applicability (González-Buesa and Salvador, 2019). Protocols and methodological guidance for most techniques have been published (Table 1) and will not be covered here. Rather this review focuses on how ongoing innovation in the use of respiratory techniques is allowing new experimental designs. In this regard, adapting technology originally proposed for animal research has and will continue to be an important contributor to expanding the repertoire of plant respiration methods (Box 1). Advancements in metabolic modeling techniques such as metabolic flux analysis, flux balance analysis, and carbon isotope labeling studies will be highly complementary as they allow enzyme-level resolution of carbon fluxes and carbon dioxide production. These techniques are beyond the scope of this review but the advancements they provide for respiration research have been recently reviewed elsewhere (Sweetlove et al., 2013; Tcherkez et al., 2017; Xu et al., 2021).

Examples of experimental equipment used for measuring respiration rates. A, A set of in-parallel Clark-type oxygen electrode measurement chambers. B, A portable infrared gas analyzer (IRGA) device. C, A set of three in-parallel IRGAs combined with a bead bath for higher-throughput CO2 release measurements of plant roots. D, An automated high-throughput O2 sensor that measures O2 consumption rate (OCR) in closed tubes with caps containing O2-sensitive fluorescent dyes.
There is important methodological and analytical crossover between the plant and animal respiration fields (Hammond and Diamond, 1997). Platinum cathode detection of O2 consumption, the bedrock of respiration studies for most of the twentieth century, was originally applied in 1938 to the measurement of photosynthesis and respiratory O2 evolution and consumption by an alga Ulva thallus and aquatic plant from the Potamogeton genus (Blinks and Skow, 1938). It was then refined in 1956 by Leland Clark with the addition of a polyethylene membrane semipermeable to O2 that enabled O2 partial pressure to be measured in solution (Severinghaus and Astrup, 1986). This enabled the technique to measure oxygen dissolved in blood (Kreuzer, 1957), an important breakthrough in medical research. The Clark-type electrode subsequently returned as a staple in measuring plant leaf, root, and mitochondrial respiration (Azcón-Bieto et al., 1983; Noguchi et al., 2005; González et al., 2006). The platinum oxygen electrode demonstrates that adapting techniques and their application from animal to plant biology (or vice versa) may be a relatively simple way of broadening the knowledge and methodology of research. A more recent example of methodological crossover is the XF96 Extracellular Flux Analyzer (Seahorse Bioscience, Billerica, MA, USA), a 96-well microplate with an adenylate selective fluorophore and optical sensor. This instrument was originally designed and used for measuring the oxygen consumption rates of mammalian cells and isolated mitochondria subject to drug or exogenous compound applications (Ferrick et al., 2008; Gerencser et al., 2009). Subsequently, the method was repurposed for plant science to characterize the growth and developmental respiratory rates of Arabidopsis leaves and roots (Sew et al., 2013). The need to hold tissue in position at the base of wells added extra technical difficulty when using the XF96 Analyzer for the purposes of leaf and root respiration, demonstrating that even when crossover occurs, unforeseen challenges will arise considering the morphological and physiological differences between animal and plant cells and tissue.
Leaf day respiration requires special mention because, despite decades of clear need, respiration in photosynthesizing tissue remains difficult to measure. Respiratory CO2 efflux and O2 uptake are confounded by the opposing CO2 uptake and O2 efflux by photosynthesis—not to mention the refixation of respiratory CO2 by photosynthesis. Several measurement techniques are currently used but none is without issue. The gas-exchange-based methods by Kok (1949) and Laisk (1977) are the simplest and most popular techniques for obtaining day respiration. They rely on measurements of CO2 efflux at low light (Kok) or low CO2 concentrations (Laisk). Whether measuring day respiration at low light or CO2 is equivalent to day respiration that would occur under higher irradiance and atmospheric CO2 concentrations may be a fundamental flaw in Kok and Laisk methods due to unintended changes of chloroplast CO2 concentrations and photorespiratory CO2 loss (Farquhar and Busch, 2017). Metabolic modeling also predicts a tight interdependency of plastidial and mitochondrial energy-generating pathways indicating that respiration and photosynthesis will be codependent (Shameer et al., 2019). A more precise but cumbersome method of determining day respiration is to trace the uptake and release of isotopes. This can be done using isotopic 16O2 and 18O2 in conjunction with membrane inlet mass spectrometry (Beckmann et al., 2009). The respiratory uptake of unnatural 18O2 by a leaf can be distinguished from 16O2 release by photosystem II. Alternatively, 18O2 can be introduced using isotopic labeled water to distinguish photosynthesis released 18O2 from respiratory 16O2 uptake (Grande et al., 1989; Gauthier et al., 2018). Similarly, isotopic labeling of carbon substrates and CO2 are not practical with high numbers of samples or in the field but provide more accurate accounts of respiratory metabolism and what substrates are being consumed by daytime respiration (Tcherkez et al., 2017). Despite widespread use, evidence to support either gas exchange or isotopic labeling methods as equating to actual daytime mitochondrial respiration rates is lacking (Tcherkez and Atkin, 2021). Furthermore, respiratory O2 versus CO2 fluxes may become largely disconnected in photosynthesizing tissues (O’Leary et al., 2019). Indeed, a recent 13CO2 flux analysis concluded that <10% of CO2 released during illumination can be attributed to mitochondrial respiration through the TCA cycle (Xu et al., 2021). Instead, the study attributed >90% of CO2 release to the conversion of glucose-6-phosphate to 6-phosphogluconate, a shunt of the Calvin–Benson Cycle of photosynthesis. Altogether, the relatively nuanced methodologies currently required to establish day respiration rates are not applicable in a high-throughput setting, greatly limiting our understanding of daytime respiratory variation.
Progress in high-throughput leaf respiration measurements
The last decade has seen the development of several methods for high-throughput O2 consumption rate (OCR) measurements in excised leaf tissue (Scafaro et al., 2017; O’Leary et al., 2022). We have previously demonstrated the power of automated O2 luminescence quenching technology (e.g. Figure 2D) in obtaining high-throughput measures of leaf respiration through OCR (Scafaro et al., 2017). The combination of robotics or multichannel sensors with O2-sensitive fluorescent dyes has led to a 10- to 26-fold faster acquisition of leaf respiration rates over previous gas-exchange methods as hundreds of samples can be measured simultaneously. These fluorophore-based systems have subsequently characterized leaf respiration in Arabidopsis, rice, wheat, Eucalyptus trees and a range of C4 type species (O’Leary et al., 2017; Rashid et al., 2020a; Fan et al., 2022; Posch et al., 2022). It has allowed for greater knowledge of how leaf respiration relates to endogenous metabolite levels (O’Leary et al., 2017; Rashid et al., 2020b). The metabolic products of daytime metabolism, such as starch and amino acids, correlated most strongly with respiration rate (see also Kruse and Adams, 2008a). The energetic demand of exporting these nutrients from leaf cells may be a major determinant of night-time respiration rate (Kruse and Adams, 2008a; O’Leary et al., 2017). Large-scale screens in wheat lines and Arabidopsis natural accessions identified an approximate two-fold variation in leaf OCR between genotypes grown in common conditions; however similar OCR variation persisted in a genetically homogenous population (O’Leary et al., 2017; Scafaro et al., 2017). Thus, it appears that substantial respiratory variation due to subtle environmental differences are difficult to eliminate even under controlled environments (see also similar studies in roots below). Automated fluorophore technology has measured the acclimation response of wheat to warmer days and nights and identified that downregulation of respiration at a given measurement temperature occurred with warmer growth conditions but more so on a O2 basis than a CO2 basis (Coast et al., 2021; Posch et al., 2022). The technology has enabled genome-wide association studies of Brachypodium species that linked leaf respiration rates to quantitative trait loci (Wilson et al., 2019), and found the rate of leaf respiration to be positively correlated with nitrogen and phosphorus concentrations in river red gum trees (Eucalyptus camaldulensis) (Asao et al., 2020). Evidently, these wide ranging and high-throughput experiments have enabled a broader understanding of genetic and environmental influences on leaf respiration.
While the examples given above are impressive, and more use of the technology will follow, there are limitations to measuring tissue respiration through OCR using a fluorophore system. The foremost limitation is that it requires a destructive harvest of leaf tissue that is separated from the plant. The extent to which an isolated leaf piece encapsulates the respiration of an intact canopy is unknown. Another limitation of fluorophore technology is that there are no commercially available CO2 reactive dyes that can operate in the gas phase alone. CO2 fluorophore dyes must rely on pH changes, which have required the sensor to be in solution or 100% humidity (Pfeifer et al., 2020). Recently, gas-phase CO2 fluorophore dyes that are stable and do not require special storage conditions to maintain viability have been developed (Fernández-Ramos et al., 2019). However, until this technology is commercially available, it is likely that there will be an increase in leaf respiration rates measured through O2 uptake rather than CO2 release. This is beneficial in that O2 uptake is more indicative of oxidative phosphorylation and thus ATP production, O2 being the terminal electron acceptor. However, in the context of screening leaf respiration to determine the impact of respiration on plant biomass accumulation and global carbon biosphere cycles, knowledge of CO2 release is more informative than O2 uptake. Measuring on an O2 or CO2 basis is not important if the RQ is at unity as is often assumed (Lambers and Oliveira, 2008); however, departures from unity are also commonly observed. For example, in wheat, warmer days and nights appear to shift the RQ to >1, with the likelihood that organic acids are being preferentially used in respiration (Coast et al., 2021; Posch et al., 2022). With an RQ not at unity, whether leaf respiration rate is measured on an O2 or CO2 basis will have implications on the accuracy of assessing energy efficiency and carbon loss. More studies focused on determining the RQ of leaves under contrasting conditions are needed, especially considering the wider use of O2 uptake as a measure of leaf respiration.
The use of a high-throughput fluorophore system may be a steppingstone to building less destructive and even wider ranging leaf respiration screening technologies. Hyperspectral reflectance is emerging as a means of screening for leaf traits on a scale that spans from individual leaves and canopies to continents through remote satellite sensing (Asner and Martin, 2008; Wang and Gamon, 2019). It is non-destructive and can measure leaf traits in situ, providing a wealth of knowledge about which environmental perturbations influence a trait of interest under ecologically, physiologically, and agriculturally relevant scenarios (Asner et al., 2014). Obtaining predictions of leaf respiration rates through hyperspectral reflectance is an indirect measurement requiring pre-determination of spectral signatures that correlate with leaf respiration. This training data must be collected by independent respiration measurements, and the more training data available the more accurate the hyperspectral models will become (Burnett et al., 2021). Automated fluorophore technology can be a means of obtaining sufficient observational data to train a hyperspectral signature to accurately predict leaf respiration rate. Indeed, fluorophore automation has been used to measure wheat dark respiration and correlate it successfully with hyperspectral reflectance measurements in the light (Coast et al., 2019). Based on training data correlations of hyperspectral and OCR measurements from 1,380 glasshouse and field-grown wheat leaf samples, a reliable predictor of leaf dark respiration rate, with an r2 of 0.54, was obtained from leaf reflectance spectra. The effect of temperature and light on wheat dark respiration were detected through this hyperspectral study, suggesting that the method has practical application in determining environmental perturbations on respiration rates. Broadening training data and hyperspectral imaging to more species and environmental conditions may result in a more generalized signature of respiration through spectra that can be used by farmers, crop breeders, and climate scientists to better record the impact of respiration on crop performance and carbon cycling. An understanding of how daytime hyperspectral images can be applied to predict respiration rates throughout the diurnal cycle are also needed.
Pairing leaf photosynthesis and respiration measurements
Tandem and high-throughput measurements of leaf photosynthetic and respiratory rates may be a powerful means of obtaining more reliable measures of tissue energy efficiency. A reduction in leaf respiration by itself may indicate improved energy efficiency, but may also be detrimental to other leaf metabolic activities; this potential tradeoff is difficult to assess by respiration alone (Reynolds et al., 2021). A better indicator of energy efficiency would be if leaf respiration rate (R) is reduced relative to CO2 assimilation (A) or photosynthetic capacity in the form of the maximum Ribulose-1,5-bisphosphate Carboxylase Oxygenase (Rubisco) CO2 fixation rates (Vcmax). The R/A or R/Vcmax ratio provides an indication of how much respiration is needed for a given photosynthetic rate or capacity, and thus, a lower R/A or R/Vcmax would imply less CO2 loss per CO2 gained or protein invested in photosynthesis, respectively. Obtaining Vcmax through hyperspectral reflectance is currently achievable (Serbin et al., 2012; Ainsworth et al., 2014; Silva-Perez et al., 2018). With the expected advances in obtaining leaf R through hyperspectral reflectance, it is likely that R/Vcmax can be screened in situ on field sites. This represents a substantial advance in the screening capabilities for plant energy use efficiency. Obtaining an R/A prediction directly through hyperspectral imaging, following training directly on R/A observations, is also a possibility. With the advance in small, modular, low-heat LED lights, illumination of leaf material can be miniaturized to sampling within wells. For example, each well in a 48-well microtiter plate was illuminated by a LED and equipped with an O2 luminescence quenching sensor, allowing individual measurements of Arabidopsis thaliana seedling photosynthetic O2 uptake and respiratory release (Loogen et al., 2021). Alternatively, distribution of light to the sample tubes within automated fluorophore systems (Figure 2D) could similarly produce measures of photosynthetic O2 efflux in leaf samples that can combine with later dark OCR measurements. Such a high-throughput fluorophore collection of R/A ratios on leaves may be sufficient to identify hyperspectral signatures that predict R/A ratios. We envisage field-based spectral screening of crops for R/A and R/Vcmax ratios as a means of selecting energy efficient genotypes and best crop husbandry practices in the coming years.
Resolving molecular mechanisms with dynamic, high-throughput respiration measurements in tissues
Despite decades of plant gas-exchange measurements, a clear (quantitative) means to relate variation in tissue respiratory rates to functional change at a molecular level has not emerged. Respiratory rates across tissues, species and physiological conditions are well correlated with several parameters such as photosynthesis, nitrogen content, and growth temperature, but these relationships are not detailed at a molecular, mechanistic level (Atkin et al., 2015). Furthermore, our ability to understand the contribution of specific genes or enzyme isoforms to respiratory flux in a tissue context has often been blocked by apparent redundancies in the network; i.e. no net change in gas-exchange flux is observed despite likely changes in the underlying properties of the respiratory network. This information gap exists partly because our predictive understanding of respiration in controlled assays of isolated mitochondria cannot be applied to intact tissues as we lack sufficient information about cellular conditions, especially metabolite and co-factor levels. However, novel techniques are allowing measurement of respiration in a more dynamic way, including simultaneous measurements of multiple respiratory parameters. These advancements should greatly improve our ability to conceptualize respiratory fluxes and better assign meaning and mechanism to observed variation.
Figure 3 provides an overview of how new molecular measurement techniques can be combined to better understand the mechanisms regulating respiration in tissues. Conceptually, respiration rate can be reduced to a supply and demand relationship, where pools of carbon substrates, especially carbohydrates, represent supply and ATP use represents demand (O’Leary et al., 2019). Four sequential and quantifiable steps interconnect supply and demand: carbon oxidation reactions, reductant pools, mitochondria electron transport chain (mETC) fluxes and adenylate pools. The ability to multiplex measurements across these six elements is critical, as we want to know how changes in one section of the model relate to changes in the other sections. This is analogous to the approach long established by measurements of respiratory quotients (CO2 release:O2 uptake ratios) and by calorespirometry, which combines measurements of CO2 release and net heat (energy) release. Calorespirometry can provide an estimate of entropic growth efficiency (chemical energy retained by net biosynthesis per unit of CO2 release), and has been successfully employed in numerous studies quantify the relationship between respiration, growth rate and temperature (Hansen et al., 2005; Kruse and Adams, 2008a, 2008b; Nogales et al., 2015). However, calorespirometry equipment and respiratory quotient methods, have yet to be adapted to perform high-throughput or dynamic measurements, which are the focus here.
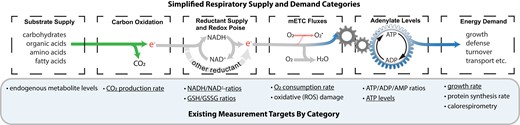
Available measurements for different components in the respiratory supply and demand chain. Measurement targets indicate molecular assays that currently exist. Underlined measurement targets denote those currently amenable to rapid dynamic measurements, making them most suitable for development of further multi-parameter respiration assays. GSH, reduced glutathione; GSSG, oxidized glutathione; ROS, reactive oxygen species; mETC, mitochondrial electron transport chain.
Multi-parameter fluorescence measurements
Perhaps more than anything else, a lack of dynamic ATP measurements has limited our understanding of respiratory relationships at the whole cell and tissue levels. We know from measurements with isolated mitochondria that ATP generation efficiency can be quite variable and respond to environmental changes such as temperature (Kerbler et al., 2019). Outside of isolated mitochondrial preparations, we cannot determine how much ATP respiration is producing. Extraction and quantification of ATP and ADP pools are possible but this does not easily allow for dynamic measurements, which could lead to quantification of respiratory supply-demand relationships. Also, the use of respiratory uncouplers commonly accelerates tissue respiration rates, indicating, in a qualitative way, that respiration rate is often feedback inhibited by high ATP:ADP ratios (Brouquisse et al. 1991). Recently, fluorescent probes have been used to detect ATP concentrations in the cytosol and plastid, which combined with knowledge of a stable total adenylate pool size (ATP, ADP, and AMP) can be used to visualize dynamic ATP level changes. Cytosolic ATP probes in seedling tissues demonstrated that ATP levels decreased sharply over the course of minutes when the mETC activity was compromised by inhibitors or anoxia (De Col et al., 2017; Voon et al., 2018; Wagner et al., 2019). Through combination measurements, De Col et al (2017) captured a modest negative correlation between ATP levels and growth rate in a population of Arabidopsis roots hairs, suggesting that higher growth rate causes sustained ATP depletion. Still, a means to quantitatively estimate respiratory-ATP production (or turnover) in cells or tissues needs to be developed.
The introduction of multiparametric fluorescence-based measurements in Arabidopsis leaf sections by Wagner et al. (2019) was an important step forward in the study of respiratory relationships. Using a suite of cytosolically expressed fluorescent probes, the authors examined in parallel, dynamic changes in the concentration of ATP, protons (pH), and Ca2+ levels alongside NADH/NAD and glutathione (GSH/GSSG) redox ratios during the gradual onset of hypoxia. Respiratory oxygen depletion in the closed wells was also monitored using an O2-sensitive fluorescent dye. The transition from aerobic respiration to anaerobic fermentation caused a dynamic decrease in cytosolic ATP and pH along with increased [Ca+] and an increasingly reduced NAD and glutathione pools. These changes were reproduced, albeit with a different dynamic, by the chemical inhibition of the mETC, demonstrating that loss of mitochondrial respiration is a key determinant of the molecular changes that accompany hypoxia.
Fluorescence monitoring of in situ NADH/NAD and NADPH dynamics in plant tissue has been further developed. Lim et al. (2020) observed multi-second, light-dependent dynamics in cytosolic and stromal NAD(P)H levels of 10-day old Arabidopsis cotyledons that required mitochondrial glycine decarboxylase activity. The results clarify the crucial role of mitochondria in supporting photosynthetic redox balancing (Lim et al., 2020). Using longer time courses of minutes to hours, Steinbeck et al. (2020) observed that both light and external sucrose greatly increased cytosolic NADH/NAD ratios in Arabidopsis leaf discs. The effect of sucrose addition suggests that reductant generation by respiratory carbon metabolism (through glycolysis and the TCA cycle) can outpace consumption of reductant by the mETC, causing large fluctuations in cytosolic NADH/NAD+ ratios. It follows that the relationship between NADH/NAD+ ratios and OCR is likely to be quite variable across conditions. Fluorescent probes also revealed that treatment of Arabidopsis seedlings with the artificial thiol reductant dithiolthreoitol (DTT) caused cellular redox effects that were more severe in mutants lacking the major alternative oxidase (AOX) isoform, AtAOX1a (Fuchs et al., 2022). This metabolic phenotype indicated that mETC fluxes can interface with the thiol redox system as well as the NAD(P)H/NAD(P) and other redox pairings.
Dynamic OCR measurements reveal respiratory regulation in action
The last decade has seen the development of novel dynamic measurements of OCR that are well suited to probing molecular mechanisms controlling respiration rate at the tissue level, including gene function. In one example, high-throughput and long duration OCR measurements of leaf discs floating on solutions were used to screen the influence of metabolite levels and other chemicals on respiration rate (O’Leary et al., 2020). Because most exogenous small molecule treatments lead to an observable increase in the cellular concentration or activity of that chemical (O’Leary et al., 2020), the relation between metabolite levels and OCR is assessable over time and on tissues with functioning signal transduction networks. Surprisingly, of all respiratory substrates, proline and alanine treatments caused the largest OCR stimulations, approximately two-fold stimulations over 14 hours. By pairing these dynamic OCR measurements with transcript analysis, enzyme knockout lines and the use of known chemical inhibitors, several disparate molecular mechanisms underpinning Pro and Ala stimulated respiration were revealed. The Pro dehydrogenase isoforms PDH1 and PDH2 were both activated transcriptionally by elevated Pro levels and were redundant in catalyzing Pro-stimulated respiration. Furthermore, AOX isoforms AOX1a and AOX1d were also strongly expressed, but were redundant in protecting cells from oxidative damage during respiratory acceleration by Pro (Oh et al., 2022). Lastly, combination treatments with additional amino acids (e.g. Ile) blocked Pro or Ala's ability to stimulate respiration. This phenomenon led to the discovery that amino acid signaling via the TOR kinase pathway coordinates amino acid respiratory fluxes in leaf cells (O’Leary et al., 2020). These examples illustrate the use of dynamic OCR measurements in elucidating different aspects of respiratory regulation, such as gene function and signal transduction pathways.
Dynamic OCR measurements were also used to provide information on the physiological status of a plant tissue with regards to defense priming and seed germination (Box 2). Various infections stimulate respiration in plants (Simons et al., 1999; Bolton, 2009). Moreover, if the plant is primed by previous infection or by chemical/hormonal treatments, then the respiratory response upon reinfection or exposure to elicitors is greater and indicative of a more vigorous defense response. Low and high-throughput, dynamic OCR measurements were used to determine specific chemical treatments that can prime plant defense in Arabidopsis seedlings (Schilling et al., 2015; Loogen et al., 2021). Though the OCR stimulations observed in these assays have not been proven to be mitochondrial respiration, they co-occur with a spike in NADH/NAD (Steinbeck et al., 2020) and an ethylene burst that can be measured in parallel using electrodes (Schulte et al., 2018).
In agronomic and horticultural practice, fast and homogeneous crop establishment is highly desirable, but the germination time of seeds within and between batches often varies substantially. Therefore, much effort is devoted to the selection and treatment of seeds to achieve rapid and uniform germination. Within minutes of imbibition, seeds display a rapid and CN-sensitive respiration rate increase that causes increased ATP generation (Hourmant and Pradet, 1981). A second increase in respiration rates is observed in many seeds at the time of radical emergence; this respiration rate is maintained and corresponds to seedling growth (Dahal et al., 1996; Bello and Bradford, 2016). Because of these relationships between respiration and germination progression, high-throughput seed OCR measurements of many individual seeds can reproduce traditional, visual measures of germination rate and seed vigor within a batch (Bello and Bradford, 2016). The quantifiable variations in OCR patterns during germination, once better understood, will elucidate differences in underlying processes of seed germination across species, varieties and conditions (Bradford et al., 2013). For example, the post-imbibition respiratory-ATP dynamic was recently re-investigated using a combination of fluorescent molecular probes, which also captured the rapid reduction of mitochondrial glutathione pool (Nietzel et al., 2020). Mutants perturbed in mitochondrial thiol redox biochemistry demonstrated increased respiratory rates to achieve the same or lesser ATP increases, suggesting that protein thiol redox switches are involved in optimizing respiratory efficiency following imbibition. Due to their versatility, high-throughput and dynamic seed respiration measurements have a clear agricultural application in the selection of genetic variants, environmental conditions and chemical treatments that optimize seed vigor and storage longevity.
Future of dynamic respiration measurements
Most of the fluorescent and dynamic OCR measurements described above have only recently been introduced and many combinations of these measurements have not yet been attempted. Future work should explicitly target the relationships between oxygen consumption, ATP levels and NADH and redox status under a range of physiological conditions. This is most obviously accomplished by developing methods to pair dynamic, fluorescent based OCR measurements with fluorescent probes for ATP levels and redox status. Superimposing images of NADH/NAD ratios and ATP levels across Arabidopsis seedling tissues already indicates potential correlations (Steinbeck et al., 2020); however, direct quantification of this relationships remains to be pursued. Information derived from these experiments could lead to a much needed foundation for predicting respiratory supply and demand dynamics and thereby improve respiratory modeling. Innovation should also continue on other important respiratory measurements, such as RQ, calorespirometry and isotope discrimination, to allow these measures to be incorporated as additional parameters in high-throughput studies of tissue respiration (Arnholdt-Schmitt et al., 2016).
Root respiration—shedding light on the hidden half
Roots are responsible for anchoring the plants to soil, uptake of water and nutrients, and serving as the interface with the soil microbial community (Lynch, 1995; Smith and De Smet, 2012). Depending on plant species, climate, and soil conditions, root respiration may account for as much as 52% of photosynthate use (Lambers et al., 1996). Therefore, understanding the energetic needs of the root system as it relates to function is needed, though roots have often been neglected as the “hidden half” in respiration research (Lambers et al., 1996). Root respiration rates (typically measured by IRGA) vary due to root tissue density, anatomy, activity, chemistry, structure, and substrate supply from leaves (Ben-Noah and Friedman, 2018). Foundational information about plant respiratory responses to carbohydrate starvation were also obtained by measuring excised root respiration using oxygen electrodes (Brouquisse et al., 1991). While these methods are destructive, intact roots have been measured by sealing distal root system portions inside specialized chambers (Bekku et al., 2011). However, new methodological approaches are needed to allow incorporation of root respiration measurements into larger scale studies.
Recently, Guo et al. (2021) used a hydroponics growth platform to grow four replicates of 276 winter wheat genotypes, harvest the excised root system, and measure the entire root system using 3 IRGAs in parallel. Samples were processed sequentially to reduce time out of hydroponics to avoid desiccation. This allowed the recent genetic analysis of root respiration, discovering several single nucleotide polymorphisms and plausible gene candidates correlated to respiration. Every root system was scanned and the images were analyzed with RhizoVision Explorer (Seethepalli et al., 2021), which allowed measurements of the axial (first order) root volume, lateral (second order) root volume, and lateral root tip volume. Multiple regression modeling allowed estimating the contributions of these three root tissue types to total root system respiration, demonstrating that lateral root tips have much greater respiration proportionate to their size. At the same time, residuals of this model identified genotypes with total root system respiration lower than expected based on the root system architecture, potentially indicating enhanced respiratory efficiency. Expanding this type of multivariate approach to include additional measurements of root anatomy and more advanced statistics, such as machine learning, would allow a functional phenomics approach (York, 2019) toward understanding influences upon root respiration.
Luxury respiration—do roots take more than they need?
In literature considering plant respiration as a target for breeding, the concept of plant luxury respiration as, “the use of photosynthate for processes that are not necessary or beneficial for growth” is often invoked in different ways (Evans, 1994; Amthor et al., 2019; Garcia et al., 2022; York et al., 2022). Researchers have suggested possible “redundant”, inefficient or wasteful processes that could contribute to luxury respiration, such as protein turnover or futile cycles of ATP consumption (Amthor et al., 2019). The problem with the concept of luxury respiration is that it is difficult to quantify. Respiration is an aggregate measure that results from hundreds of underlying processes, and is closely coupled to other aspects of cellular metabolism. Because root tissue is heterotrophic, it offers perhaps a simpler model for studying carbon use efficiency of plant respiration, away from the complications of integration with photosynthetic and source tissue metabolism. The work of Guo et al (2021) that showed a negative relationship of root respiration rates to shoot mass, suggesting lower root respiration might improve shoot growth. While such statistical modeling may be useful, we clearly cannot believe the implication that zero respiration will maximize plant growth. Rather, a tension exists between minimizing respiration while maximizing processes that are supported by respiration. For example, Griffiths et al (2021) showed a positive relationship of root respiration and nutrient uptake ability across 26 maize lines. Other work on respiration and nutrient uptake indicated that uncontrolled nutrient efflux from the root could account for a substantial portion of respiration (Scheurwater et al., 1999). Therefore, a balance among competing processes is needed to reduce wasteful luxury respiration while maintaining essential plant processes. How to achieve this balance and identify luxury respiration remains an open question. Development of higher-throughput root respiration methods that allow the simultaneous (or in parallel) measurements of many parameters on diverse samples, such as from diversity panels, may allow researchers to unravel which processes contribute to luxury respiration, or even specific associated genes.
The importance of open protocols, data, and code
For plant respiration advancements to occur more quickly, open science practices such as sharing protocols, data, and code are imperative. Many routes of sharing are possible including protocol repositories such as Protocols IO, or protocol-specific journals such as Plant Methods, Bio-protocols, or Current Protocols. The inclusion of illustrated protocols and R statistical codes are useful, as in recent protocols released for dark respiration in leaves and roots (Guo et al., 2020; Fonseca et al., 2021). Depositing respiration data in plant trait databases such as TRY (Kattge et al., 2020) or Zenodo will facilitate their use beyond a single study and enable global patterns of plant respiration to be ascertained, aiding terrestrial carbon models. Importantly, respiration is sensitive to environmental changes, in particular temperature and light (including from preceding periods), so care should be taken to ensure environmental uniformity, and whenever possible measures of temperature and humidity should be included (see (Freschet et al., 2021). By sharing their methods and protocols researchers increase the integrity and impact of their work and the research community as a whole, promoting inclusion and diversity in the field.
Conclusion
We have outlined major advances and challenges related to the high-throughput, dynamic and multi-dimensional measurements of plant respiration. What is exciting for plant respiration research is the increased data richness, nuance and statistical power these new methods will bring. In the near future, these advancements should facilitate more powerful genetic analyses to link respiratory variation to specific alleles, and not just in leaves but roots and seeds as well (see Outstanding Questions box). Increased understanding of environmental influence will allow better predictions of how plants will respond to climate change at the global scale. Altogether, greater experimental adaptability will allow respiration to become a more integral part of our understanding of plant behavior and performance.
Can development of high-throughput paired measurements of leaf respiration and photosynthesis allow for selection of more energy/carbon-efficient crops?
Can the underlying relationships between respiration rate, NADH/NAD, and ATP levels in plant tissues be quantified using combinations of metabolite and O2 fluorescent probes?
Can high-throughput respiration measurements be better adapted for field measurements?
How can luxury respiration be quantified? What methods can determine if observed rates of respiratory carbon use are fully required for tissue performance in a given environment?
Acknowledgments
This manuscript has been authored in part by UT-Battelle, LLC, under contract DE-AC05-00OR22725 with the US Department of Energy (DOE). The US government retains and the publisher, by accepting the article for publication, acknowledges that the US government retains a nonexclusive, paid-up, irrevocable, worldwide license to publish or reproduce the published form of this manuscript, or allow others to do so, for US government purposes. DOE will provide public access to these results of federally sponsored research in accordance with the DOE Public Access Plan.
Funding
Research in BMO's lab is supported by the Government of Saskatchewan, Agricultural Development Fund grant #20210689. A.P.S. is supported by an Australian Research Council DP22 grant (DP220101882). This work was partially supported by the Center for Bioenergy Innovation (L.M.Y.), a US Department of Energy (DOE) Bioenergy Research Center supported by the Office of Biological and Environmental Research in the DOE Office of Science.
Author contributions
All authors conceived and designed the article and contributed to writing the article.
References
Author notes
The author responsible for distribution of materials integral to the findings presented in this article in accordance with the policy described in the Instructions for Authors (https://dbpia.nl.go.kr/plphys/pages/General-Instructions) is: Brendan O’Leary ([email protected]).
Conflict of interest statement. None declared.