-
PDF
- Split View
-
Views
-
Cite
Cite
Antonella Ferela, Juan Manuel Debernardi, Santiago Rosatti, Daniela Liebsch, Carla Schommer, Javier F Palatnik, Interplay among ZF-HD and GRF transcription factors during Arabidopsis leaf development, Plant Physiology, Volume 191, Issue 3, March 2023, Pages 1789–1802, https://doi.org/10.1093/plphys/kiad009
- Share Icon Share
Abstract
The growth-regulating factor (GRF) family of transcriptional factors are involved in the control of leaf size and senescence, inflorescence and root growth, grain size, and plant regeneration. However, there is limited information about the genes regulated by these transcriptional factors, which are in turn responsible for their functions. Using a meta-analysis approach, we identified genes encoding Arabidopsis (Arabidopsis thaliana) zinc-finger homeodomain (ZF-HD) transcriptional factors, as potential targets of the GRFs. We further showed that GRF3 binds to the promoter of one of the members of the ZF-HD family, HOMEOBOX PROTEIN 33 (HB33), and activates its transcription. Increased levels of HB33 led to different modifications in leaf cell number and size that were dependent on its expression levels. Furthermore, we found that expression of HB33 for an extended period during leaf development increased leaf longevity. To cope with the functional redundancy among ZF-HD family members, we generated a dominant repressor version of HB33, HB33-SRDX. Expression of HB33-SRDX from HB33 regulatory regions was seedling-lethal, revealing the importance of the ZF-HD family in plant development. Misexpression of HB33-SRDX in early leaf development caused a reduction in both cell size and number. Interestingly, the loss-of-function of HB33 in lines carrying a GRF3 allele insensitive to miR396 reverted the delay in leaf senescence characteristic of these plants. Our results revealed functions for ZF-HDs in leaf development and linked them to the GRF pathway.
Introduction
Leaves are initiated at the flanks of the shoot apical meristem, first as a rod-shaped primordium that grows to generate a flat lamina. At an early stage, cells in the whole primordium undergo rapid cycles of cell divisions. As the organ grows, cell proliferation becomes restricted to the base of the leaf, then it ceases, and cell expansion becomes the main driving force of the organ growth (reviewed in Gonzalez et al., 2012; Tsukaya, 2013; Rodriguez et al., 2014; Nikolov et al., 2019; Vercruysse et al., 2019). Once organ maturation is achieved, the leaf switches from a metabolic sink to being the primary source of carbohydrates for the plant. At its final stage, the leaf enters the process of senescence, a complex program aimed at recycling valuable leaf resources and involving the decrease of photosynthesis and chlorophyll content until eventually leading to the death of the leaf cells (Lim et al., 2007; Woo et al., 2019).
Leaf developmental stages require the interplay of many transcription factors and signaling pathways. The growth-regulating factors (GRFs) are a plant-specific family of transcription factors, key regulators of plant growth and development (reviewed in Kim and Tsukaya, 2015; Omidbakhshfard et al., 2015; Kim, 2019; Liebsch and Palatnik, 2020). The GRF family is defined by the presence of WRC and QLQ domains, involved in DNA-binding and protein–protein interaction, respectively. Most of the GRFs in angiosperms are regulated by microRNA miR396 (Debernardi et al., 2012; Beltramino et al., 2021). In Arabidopsis (Arabidopsis thaliana), seven GRFs have a target site for miR396, limiting their expression in leaves to younger tissues harboring proliferating cells (Rodriguez et al., 2010; Debernardi et al., 2014). Combination of mutations in several GRFs (GRF1–5) (Kim et al., 2003; Kim and Kende, 2004; Horiguchi et al., 2005; Kim and Lee, 2006; Kim et al., 2012) or overexpression of miR396 (Liu et al., 2009; Rodriguez et al., 2010; Wang et al., 2011a) cause a reduction in cell proliferation and leaf size, indicating a partial functional redundancy of the GRFs. On the other hand, higher expression levels in several GRFs, achieved by the overexpression of GRF5 or the introduction of silent mutations in the miR396-binding site of GRF2 (rGRF2) or GRF3 (rGRF3) that eliminate the post-transcriptional repression by the miRNA, generate bigger leaves with more cells (Gonzalez et al., 2010; Rodriguez et al., 2010; Debernardi et al., 2014; Beltramino et al., 2018). Plants overexpressing GRF5 or rGRF3 also have a delay in leaf senescence (Debernardi et al., 2014; Vercruyssen et al., 2015), suggesting that GRFs have diverse functions in leaf development. Interestingly, it is possible to uncouple the effects of GRF3 on the increase in cell number and leaf longevity by expressing the gene encoding this transcription factor from developmental stage-specific promoters (Debernardi et al., 2014).
While the miR396-GRF module has been implicated in diverse developmental processes, such as the leaf, inflorescence and root growth, grain size and plant regeneration (Kim and Tsukaya, 2015; Omidbakhshfard et al., 2015; Kim, 2019; Liebsch and Palatnik, 2020), little is known about the genes directly regulated by GRF transcription factors, which are, in turn, responsible for their specific functions. By performing a meta-analysis of transcriptomic data, we found a connection between the GRFs and a group of transcription factors belonging to the zinc-finger homeodomain (ZF-HD) family. The ZF-HD family harbor a zinc-finger (ZF) domain that enables the formation of homo- or heterodimers, and a homeodomain (HD), which binds to DNA (Windhovel et al., 2001; Tan and Irish, 2006; Hu et al., 2008; Bollier et al., 2022). In Arabidopsis, the ZF-HD family is composed of 14 members whose known functions include control of cell size (Hong et al., 2011; Bueso et al., 2014) and the regulation of seed development (Street et al., 2008; Hong et al., 2011; Bueso et al., 2014), hypocotyl and root hair elongation (Perrella et al., 2018; Zhao et al., 2021), the response to abiotic and biotic stresses (Tran et al., 2007; Barth et al., 2009; Nishiyama et al., 2012), and hormone signaling (Wang et al., 2011b). Here, we found that the ZF-HDs are an essential family of transcription factors that regulate leaf size and senescence. We further demonstrate that GRF3 directly activates HOMEOBOX PROTEIN 33 (HB33), which encodes a ZF-HD transcription factor, to regulate leaf senescence, providing a mechanistic link between the two families of transcription factors.
Results
A transcriptome meta-analysis revealed a connection of GRFs and ZF-HD transcription factors
To gain insight into the regulatory network controlled by the GRFs, we performed a meta-analysis of the transcriptome of plants with high GRF expression, p35S:GRF5 (Gonzalez et al., 2010) and pGRF3:rGRF3 × p35S:GIF1 (Debernardi et al., 2014), and seedlings and vegetative apices of p35S:MIR396 plants with reduced transcript levels of miR396-targeted GRFs (Rodriguez et al., 2010; Schommer et al., 2014). This analysis revealed that the expression of 644 genes positively associated (P < 0.001) and 203 genes negatively associated with GRF activity (P < 0.001) (Figure 1, A and B and Supplemental Tables S1 and S2).
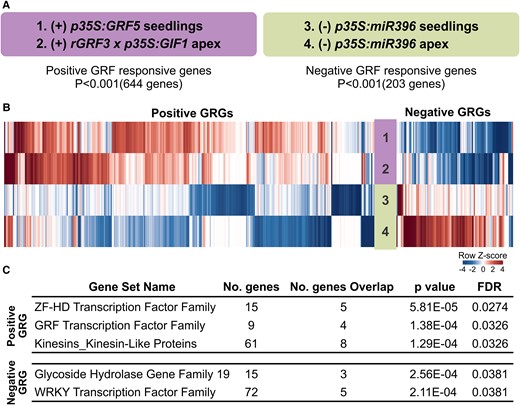
Identification of gene families regulated by GRF transcription factors. A, GRF responsive genes obtained from a meta-analysis of microarrays of plants with increased GRF levels (+) and decreased GRF levels (−). B, Heatmap showing the expression of GRF responsive genes from the four different microarrays analyzed in A. C, Gene families enriched among the positively and negatively associated genes identified in the meta-analysis. GRG = GRF responsive genes.
Next, we performed a family-based gene set enrichment analysis (Yi et al., 2013). As expected, members of the GRF family (GRF1–3, GRF5) were positively correlated with GRF activity, which served as a positive control for our analysis (Figure 1C). In addition, we identified eight genes encoding kinesin-like proteins that were positively correlated with GRF activity (P = 1.29E−04). These proteins belong to a superfamily of microtubule-base motor proteins essential for intracellular transport, cell shape, and cell division (Reddy and Day, 2001; Zhu and Dixit, 2012; Figure 1C), which might be linked to the known functions of the GRFs in the control of cell proliferation (Horiguchi et al., 2005; Rodriguez et al., 2010). Among the negatively correlated genes, we observed enrichment for five genes encoding members of the WRKY transcription factor superfamily and three Glycoside Hydrolase Family 19 genes (Figure 1C), which are usually linked to stress and pathogen responses (Samac et al., 1990; Eulgem et al., 2000). Interestingly, at the top of our analysis, we found five genes encoding ZF-HD family transcription factors (HB22, HB28, HB31, HB32, and HB33) that were positively correlated with GRF activity (P = 5.81E−05) (Figure 1C), which prompted us to study a potential link between GRFs and ZF-HD transcription factors.
GRF3 directly activates HB33 expression
A phylogenetic analysis of the Arabidopsis ZF-HD family revealed that four of the five ZF-HD genes that were positively associated with GRF expression clustered in closely related clades (Figure 2A). Interestingly, an analysis of ZF-HD expression during plant development using publicly available data from wild-type plants (Schmid et al., 2005; Toufighi et al., 2005) revealed similar expression patterns between the five ZF-HDs and the four GRFs identified in our meta-analysis (Figure 2, B and C).
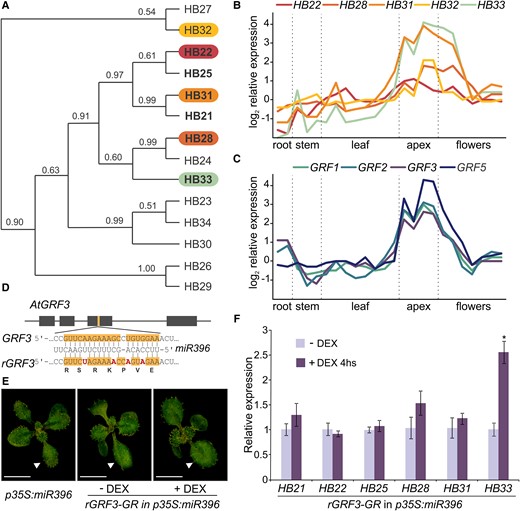
Expression analysis of ZF-HD family members. A, Phylogenetic tree of the ZF-HD family in Arabidopsis (adapted from Tan and Irish, 2006); the five ZF-HD genes selected as positively regulated by GRFs in our meta-analysis are highlighted. B and C, Expression profile of the five ZF-HD positive GRF responsive genes and four of the GRFs enriched in the meta-analysis in different plant tissues. Data obtained from AtGenExpress corresponding to Col-0 samples (Schmid et al., 2005; Toufighi et al., 2005). D, Scheme of the synonymous mutations introduced in rGRF3 to dampen the interaction with miR396. E, Image of phenotype complementation of p35S:miR396 plants transformed with pGRF3:rGRF3-GR-GFP (rGRF3-GR), grown in petri dishes with MS in the presence (+ DEX) or absence (− DEX) of 5 μM DEX. The white arrowheads show the position of leaf #1 or #2. Scale bar: 1 cm. F, Relative expression of six ZF-HDs in apices from rGRF3-GR p35S:miR396 plants treated with 30 µM DEX for 4 h, using the same line without DEX treatment (−DEX) as a control. Bars represent means ± standard error of the mean (SEM; n = 3) and the asterisk indicates a statistically significant difference to the non-treated control line, as determined by Student's t-test (P < 0.05).
To test if GRFs could directly regulate the ZF-HDs, we used a GRF3-inducible system (pGRF3:rGRF3-GR-GFP [rGRF3-GR]), which expresses a miR396-resistant GRF3 (Figure 2D) fused to Green Fluorescent Protein (GFP) and a Glucocorticoid Receptor (GR) domain that translates into a GRF3 protein which becomes activated upon treatment with dexamethasone (DEX) (Rodriguez et al., 2015). These experiments were performed in a p35S:miR396 background, which has low levels of endogenous miR396-targeted GRFs, resulting in smaller plants in the absence of DEX (Figure 2E).
We measured the transcript levels of HB22, HB28, HB31, and HB33, which were positive GRF responsive genes in our meta-analysis (Figure 1), and of two phylogenetically related ZF-HDs genes (HB21 and HB25; Figure 2A) in mock- and DEX-treated rGRF3-GR plants (Figure 2, E and F). We found that HB33 showed the highest response to the activation of GRF3-GR, with a significant increase of more than 2.5-fold (Figure 2F). In addition, we observed a small increase in the transcript levels of three other ZF-HDs (HB21, HB28, and HB31) (Figure 2F).
To study the link between GRF3 and HB33 in more detail, we generated a transcriptional reporter for HB33 by fusing 3.1 kb upstream of the coding sequence of HB33 with the β-glucuronidase (GUS) reporter gene (Figure 3A). The reporter revealed its expression in young leaves and flower buds, in agreement with microarray data (Figure 2B). Interestingly, a reporter with a shorter regulatory region (2.2 kb of the promoter region) had lower expression levels (Supplemental Figure S1), suggesting the existence of regulatory elements further apart from the coding sequence.
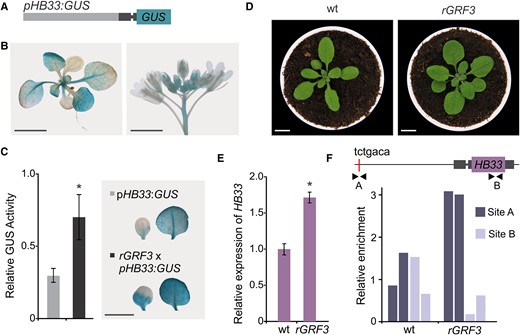
GRF3 directly regulates HB33 expression. A, Schematic representation of the vector expressing HB33 promoter (the 5′ Untranslated region, UTR, is dark gray with an intron that is indicated as a thinner line). B, GUS staining of representative 12-day-old and inflorescence Arabidopsis transgenic plants of pHB33:GUS reporter. Scale bar: 1 cm. C, GUS activity in cotyledons and leaf #1 and 2 of pHB33:GUS (light gray) and pHB33:GUSxpGRF3:rGRF3-GFP (rGRF3; dark gray) plants, relative GUS activity to pHB33:GUS activity. Bars represent means ± SEM and the asterisk indicates a statistically significant difference from the wild type (P < 0.05) by Student's t-test (n = 10). Representative GUS staining of a cotyledon and a leaf from each genotype are shown on the right. Images were digitally extracted for comparison. Scale bar: 1 cm. D, 25-day-old rosettes from wild-type (wt) and transgenic rGRF3 lines. Scale bars: 1 cm. E, Relative expression of HB33 in rGRF3 inflorescences. Bars represent means ± SEM and the asterisk indicates a statistically significant difference to the wild-type (P < 0.05) by Student's t-test (n = 3). F, ChIP-qPCR analysis of apex samples from wild-type (wt) and rGRF3 plants. Enrichment was tested in the putative GRF-binding site (site A, tctgaca) and in HB33 coding region (site B) indicated by black arrowheads in the schematic HB33 genomic region. Data from two biological replicates.
Next, we turned to pGRF3:rGRF3-GFP (rGRF3) plants, which have a moderate increase in GRF3 due to the lack of repression by miR396, resulting in leaves larger than wild-type leaves (Debernardi et al., 2014; Beltramino et al., 2018; Figure 3D). The transcript levels of HB33 were increased in rGRF3 plants, as expected (Figure 3E and Supplemental Figure S2). In addition, we analyzed a miR396ab mutant (Hou et al., 2019) and found a significant increase of HB33 transcript levels (Supplemental Figure S2). Overall, our data show that the GRFs, and in particular GRF3, activate HB33 expression.
Next, we crossed rGRF3 with a pHB33:GUS reporter line and performed a quantitative determination of GUS activity. We found a significant increase in GUS activity (approximately 3 times) in rGRF3 plants compared with in wild-type plants (Figure 3C). Visualization of the HB33 reporter activity in whole seedlings evidenced a higher and expanded pattern of expression in cotyledons and leaves (Figure 3C).
Available data about the transcription factor binding sites in Arabidopsis show that GRFs can bind to TGTCAGA sequences (Kim et al., 2012; O’Malley et al., 2016; Kim, 2019). An analysis of the HB33 regulatory sequences identified a perfect match of this sequence approximately 3 kb upstream of the HB33 coding sequence (Figure 3F). We performed chromatin immunoprecipitation followed by quantitative Polymerase Chain Reaction (ChIP-qPCR) on apices from rGRF3 and wild-type plants using GFP as a tag for the immunoprecipitation. PCR amplification was done with oligonucleotides flanking the TGTCAGA sequence (site A) and another site located in the coding region of HB33 (site B). We observed at least a two-fold enrichment at the GRF-binding site in the HB33 promoter (site A) in rGRF3 plants compared to in control plants in independent experiments (Figure 3F). As expected, no enrichment was observed at the B site (Figure 3F). Taken together, these data indicated that GRF3 can directly bind to the HB33 promoter region and activate its expression.
ZF-HDs are critical for plant development
A T-DNA knockout line for HB33 (hb33, SALK_097388) did not reveal any obvious growth defect, in agreement with previous results (Tan and Irish, 2006; Hu et al., 2008; Bueso et al., 2014; Supplemental Figure S3), and suggested that single ZF-HD mutants do not have obvious developmental defects due to their functional redundancy. As ZF-HDs can form homo- and heterodimers, we generated a dominant repressor version of HB33 by fusing it to the C-terminus of the Ethylene-responsive element binding factor-associated Amphiphilic Repression (EAR) motif (SRDX, pHB33:HB33-SRDX) (Hiratsu et al., 2003; Kagale and Rozwadowski, 2011; Figure 4A). The resulting primary transformed Arabidopsis plants harboring pHB33:HB33-SRDX displayed strong developmental defects (35 out of 35 analyzed plants) (Figure 4B). Few plants survived after two weeks in soil, which remained very small with few leaves (Figure 4C), demonstrating the importance of the ZF-HD family of transcription factors in plant development.
![A dominant negative version of HB33 (HB33-SRDX) affects plant growth and development. A, Schematic representation of the vectors expressing pHB33:HB33-SRDX (light gray [HB33 promoter, the 5′ UTR harbors an intron indicated in dark gray], purple [HB33], and orange [SRDX]) and pANT:HB33-SRDX. B, Control plants transformed with an empty vector (left) and three independent pHB33:HB33-SRDX seedlings (right). Scale bar: 1 mm. C, 16-day-old rosettes of plants transformed with an empty vector or pHB33:HB33-SRDX. Scale bars: 1 cm. Zoom in of the pHB33:HB33-SRDX plant (scale bar: 1 mm). D, 21-day-old rosettes of empty vector or pANT:HB33-SRDX plants. Scale bars: 1 cm. E–G, Leaf area, cell area, and cell number of fully expanded first pair of leaves of wild-type plants transformed with the empty vector or pANT:HB33-SRDX. Bars represent means ± SEM and an asterisk indicates a significant difference compared to the empty vector (P < 0.05) by Student's t-test.](https://oup.silverchair-cdn.com/oup/backfile/Content_public/Journal/plphys/191/3/10.1093_plphys_kiad009/1/m_kiad009f4.jpeg?Expires=1747970256&Signature=fc7UvzR8eRSl5tgNUVnqNFx7L4XY33Coo6PfYpalAGJ1NoJ8cX8tErkkKLBQ9xix2ttB49Gdcf38vAm4s3-8v5oPLfRdETXgZGRBrwg8xD-pVUnCaJ~r8nU~f-7SNVGM5fzNHr~f95Gp3QtMCC4nKFJDD~eCrFTuUMsujB5fZlBDXaSIzNxTi6WtICo41dTpfglt33qX5n1BH9gIni0SZvE6b262AE3Z~AKNFm8VxNxAi09BWGPLFmZiW3TLzEdLD~VboZkcnMu8ApCbMq5S4-Nl8g8MmZAQi0~KO2ceic-M920TqLJAgdTINIOT5WAvkxBK3VmMoLScJUADQc6aTw__&Key-Pair-Id=APKAIE5G5CRDK6RD3PGA)
A dominant negative version of HB33 (HB33-SRDX) affects plant growth and development. A, Schematic representation of the vectors expressing pHB33:HB33-SRDX (light gray [HB33 promoter, the 5′ UTR harbors an intron indicated in dark gray], purple [HB33], and orange [SRDX]) and pANT:HB33-SRDX. B, Control plants transformed with an empty vector (left) and three independent pHB33:HB33-SRDX seedlings (right). Scale bar: 1 mm. C, 16-day-old rosettes of plants transformed with an empty vector or pHB33:HB33-SRDX. Scale bars: 1 cm. Zoom in of the pHB33:HB33-SRDX plant (scale bar: 1 mm). D, 21-day-old rosettes of empty vector or pANT:HB33-SRDX plants. Scale bars: 1 cm. E–G, Leaf area, cell area, and cell number of fully expanded first pair of leaves of wild-type plants transformed with the empty vector or pANT:HB33-SRDX. Bars represent means ± SEM and an asterisk indicates a significant difference compared to the empty vector (P < 0.05) by Student's t-test.
We also expressed HB33-SRDX from the AINTEGUMENTA (ANT) promoter, which is active during early leaf development (Schoof et al., 2000), albeit at lower levels than pHB33 (Supplemental Figure S4). While a few plants died during seedling development (2 plants out of 35 primary transgenic plants), most of them (23 out of 35 analyzed plants) displayed a reduction in leaf size and narrower leaves (Figure 4, D and E). Cellular analysis of pANT:HB33-SRDX plants revealed a reduction in both cell size and number (Figure 4, F and G). Taken together, these results indicate that ZF-HDs have several relevant roles in leaf and plant development.
Effects of increased levels of HB33 expression on leaf size
We then tested the effects of increased expression of HB33 by preparing a vector expressing it under the control of the UBIQUITIN10 (UBQ10) promoter (pUBQ10:HB33). We analyzed 70 independent transgenic plants and observed that pUBQ10:HB33 plants could be classified into two groups based on their phenotype. Around 20% of the pUBQ10:HB33 primary transformants had larger leaves than those of control plants, while 40% of the primary transformants had smaller and darker leaves (Figure 5, A, C, and D). We determined HB33 transcript levels and found that plants with larger leaves had approximately three to five times higher HB33 transcript levels compared to levels in control plants carrying the empty vector, while plants with smaller and darker leaves had at least an eight-fold increase (Figure 5B). At the cellular level, leaves of pUBQ10:HB33 plants with moderate expression of HB33 exhibited an increase in both cell size and number compared with control plant leaves, while leaves of pUBQ10:HB33 plants with higher levels of HB33 had a reduced cell size but similar cell number (Figure 5, E and F). Overall, the results show that decreasing HB33 expression (Figure 4, D–G) reduced cell number and leaf size, while a moderate increase in HB33 expression caused the opposite effects (Figure 5). In addition, strong overexpression of HB33 can cause deleterious effects reducing leaf size.
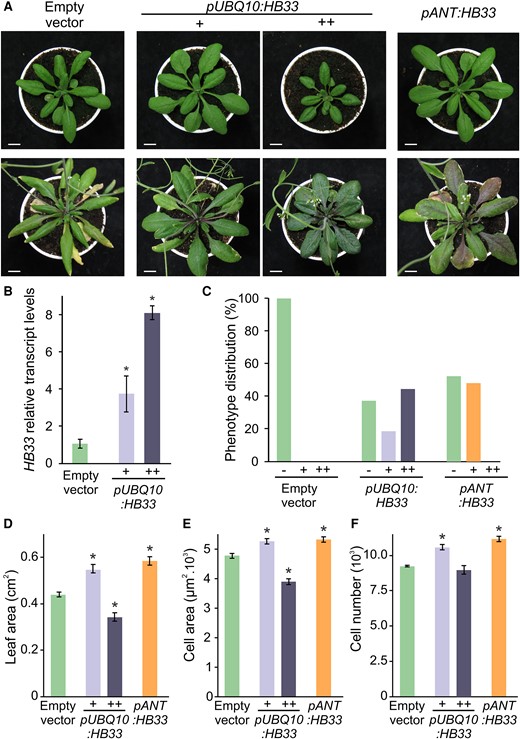
Effects of HB33 levels on leaf development. A, Phenotypes of 3-week-old (top) and 5-week-old (bottom) rosette of wild-type plants transformed with empty vector, plants overexpressing HB33 under UBQ10 promoter (pUBQ10:HB33) with larger (+) and smaller darker (++) leaves, and plants expressing HB33 under ANT promoter (pANT:HB33). Scale bars: 1 cm. B, Relative expression levels of HB33 in plants transformed with an empty vector and pUBQ10:HB33 with larger (+) and smaller darker (++) leaves. Bars represent means ± SEM and asterisks indicate significant differences compared to the empty vector (P < 0.05) by Student's t-test. C, Distribution of leaf phenotype in empty vector, pUBQ10:HB33 and pANT:HB33 primary transformant. D–F, Leaf area, cell area, and estimation of cell number of fully expanded first pair of leaves of wild-type plants transformed with empty vector, pUBQ10:HB33 with larger (+) and smaller darker (++) leaves, and pANT:HB33. Bars represent means ± SEM and an asterisk indicates a significant difference compared to the empty vector (P < 0.05) by Student's t-test.
We then generated a vector that expressed HB33 from the ANT promoter (pANT:HB33). Analysis of 43 pANT:HB33 primary transformants revealed that none had the smaller and dark leaf phenotype (Figure 5C) caused by high expression of HB33 from the UBQ10 promoter. Instead, nearly half of them had larger leaves (Figure 5, A, C, and D), with increased cell size and number, similar to plants expressing moderate levels of HB33 under the UBQ10 promoter (Figure 5, E and F). These results indicate that both the dosage and the expression pattern of HB33 can affect leaf phenotype.
Control of leaf longevity by HB33
During the analysis of pUBQ10:HB33 plants, we noticed that they had a delay in leaf senescence. While eight-week-old wild-type plants displayed obvious signs of senescence, including yellowish leaves, pUBQ10:HB33 plants were still green and turgid (Figure 6A). pUBQ10:HB33 plants expressing moderate or high HB33 levels showed delayed senescence, although the strong overexpressors having small leaves showed a greater delay (Figure 6, B and C). This was not due to a general delay in development, as pUBQ10:HB33 plants did not show any obvious change in flowering time (all the analyzed lines flowered 22 days after planting with 13 leaves, similar to wild-type plants). On the other hand, plants expressing HB33 from the ANT promoter did not display signs of delayed leaf senescence (Figure 6A), suggesting that the leaf size phenotype can be uncoupled from the effect on senescence by restricting HB33 expression to younger leaf stages (Figure 6A).
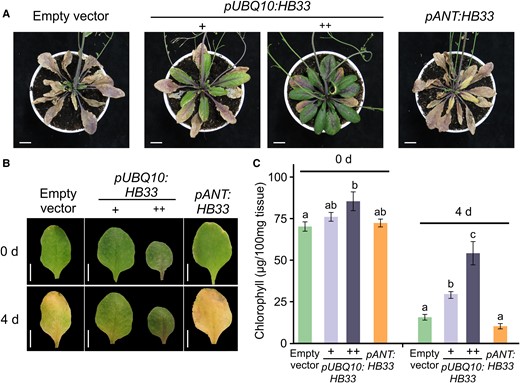
Ectopic expression of HB33 increases leaf longevity. A, Representative 55-day-old rosettes of Col-0 plants transformed with an empty vector, pUBQ10:HB33 with larger (+) and smaller darker (++) leaves, and pANT:HB33. Scale bars: 1 cm. B, Leaves of indicated plants before (0 days) and after 4 days of dark-induced senescence treatment. Images were digitally extracted for comparison. Scale bars: 1 cm. C, Total chlorophyll levels of leaves incubated 4 days in the dark. Bars represent means ± SEM and different letters indicate statistically significant differences (P < 0.05) by Analysis of Variance (ANOVA) followed by Tukey's multiple comparison test (n = 15).
Next, we performed dark-induced senescence experiments by incubating detached leaves in the dark (Figure 6, B and C). After 4 days of incubation in the dark, wild-type and pANT:HB33 leaves lost almost 90% of their chlorophyll content, while pUBQ10:HB33 plants maintained much higher levels. Strong overexpressors retained more than 50% of their chlorophyll levels and moderate overexpressors more than 30% (Figure 6C). These results suggested that constitutive overexpression of HB33 (pUBQ10:HB33), but not its expression in early leaf stages (pANT:HB33), delays senescence.
The ectopic expression of HB33 delays leaf senescence in rGRF3 plants
Higher levels of GRF activity, as seen in plants with a direct up-regulation of the transcription factors, p35S:GRF5, rGRF3, and rGRF2 (Gonzalez et al., 2010; Debernardi et al., 2014; Beltramino et al., 2018), or in plants with low miR396 activity, such as mir396ab knockouts or plants expressing a miR396 target mimicry (Beltramino et al., 2018; Hou et al., 2019), result in larger leaves than those of wild-type plants. The up-regulation of GRF transcription factor genes also results in an increase in cell longevity (Debernardi et al., 2014; Vercruyssen et al., 2015). We observed that mir396ab knockouts and MIM396 plants that have a reduction in miR396 activity had an increased longevity (Supplemental Figures S5 and S6). In addition, single and multiple mutants of GRFs show accelerated senescence (Debernardi et al., 2014; Vercruyssen et al., 2015; Supplemental Figure S6). These results demonstrate that the miR396-GRF node controls both leaf size and longevity. As we found that GRFs regulate HB33 expression, we decided to explore whether the phenotypes of rGRF3 plants are partially caused by the activation of HB33. To test this hypothesis, we generated crosses of rGRF3 with hb33 plants. We noticed that rGRF3 and rGRF3×hb33 had similar leaf sizes, both larger than wild-type leaves (Figure 7, A–C), indicating that increased leaf size in rGRF3 plants does not depend on HB33. Interestingly, while rGRF3 had an extended leaf longevity, remaining green and turgent eight weeks after planting (Figure 7A), we observed that the rGRF3×hb33 rosette life span was shorter than that of rGRF3 plants and similar to that of hb33 and wild-type plants (Figure 7A).
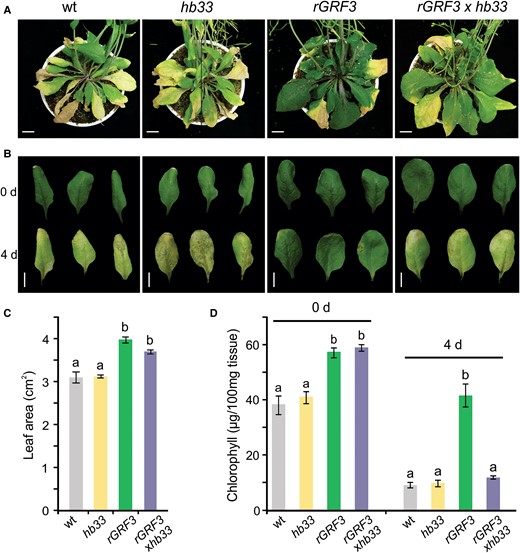
rGRF3 requires HB33 to increase leaf longevity. A, Rosette of 55-day-old wild-type (wt), hb33, rGRF3, and rGRF3×hb33 plants. Scale bars: 1 cm. B, Leaves of indicated plants before (0 days) and after 4 days of dark-induced senescence treatment. Images were digitally extracted for comparison. Scale bars: 1 cm. C, Area of fully expanded fifth leaves of wild-type (wt), hb33, rGRF3, and rGRF3×hb33 plants. D, Total chlorophyll content before and after 4 days of dark incubation of the leaves shown in B. In B and D, bars represent means ± SEM and different letters indicate statistically significant differences (P < 0.05) by ANOVA followed by Tukey's multiple comparison test (n = 12).
Next, we analyzed dark-induced senescence of hb33, wild-type, rGRF3, and rGRF3×hb33 leaves (Figure 7B). Strikingly, leaves of rGRF3×hb33 and rGRF3 plants before treatment had more chlorophyll and a larger size than leaves of control plants (Figure 7, B and D), suggesting that HB33 does not influence these aspects in rGRF3 plants. However, after 5 days of dark incubation, the levels of chlorophyll decreased significantly (approximately a 90% reduction) in rGRF3×hb33 plants to similar levels as those in wild-type plants, while chlorophyll levels remained high in rGRF3 leaves (Figure 7D), indicating that HB33 is required for the delay in leaf senescence observed in rGRF3 plants and, likely, in other GRF gain-of-function contexts.
Discussion
A wide range of transcription factors, small RNAs, and hormone pathways have been shown to participate in leaf growth and development (reviewed in Gonzalez et al., 2012; Tsukaya, 2013; Debernardi et al., 2014; Nikolov et al., 2019; Vercruysse et al., 2019). However, their mechanistic relationships are in many cases unknown. Here, we describe functions for ZF-HD transcription factors in the control of leaf growth and longevity and show that at least part of these functions are coordinated with transcription factors of the GRF class.
The ZF-HD family is composed of 14 members in Arabidopsis, which have been implicated in response to abiotic and biotic stress, hormone signaling, hypocotyl and root hair elongation, cell size, and seed development (Tran et al., 2007; Street et al., 2008; Barth et al., 2009; Hong et al., 2011; Wang et al., 2011b; Nishiyama et al., 2012; Bueso et al., 2014; Perrella et al., 2018; Zhao et al., 2021). Previous studies have shown that single mutants, such as mutants of HB22, HB23, HB25, HB27, HB31, HB32, HB33, and HB34, did not display obvious developmental defects (Tan and Irish, 2006; Hu et al., 2008; Bueso et al., 2014; Supplemental Figure S3); however, their similar expression patterns suggested that redundancy might be hindering the observation of additional functions. To cope with the potential redundancy of ZF-HDs, we generated a repressor version of HB33, HB33-SRDX, expressed from its own regulatory regions. This repressor allele, pHB33:HB33-SRDX, turned out to impair plant development at an early stage, sometimes causing death (Figure 4). The pHB33:GUS reporter is active during early leaf development, including proliferative tissues (Figure 3), in agreement with a role of HB33 and other ZF-HDs in leaf development (Hong et al., 2011; Hauser et al., 2013). Interestingly, expressing HB33-SRDX from the ANT promoter allowed us to recover adult plants that displayed a strong reduction in leaf size (Figure 4), indicating that ZF-HDs play key roles in development and specifically in the promotion of leaf growth.
MINI ZINCFINGER1 (MIF1) harbors a ZF dimerization domain that can interact with ZF-HD transcription factors; however, as it lacks the HD and DNA-binding activity, it has been proposed to act as an inhibitor of ZF-HD activity (Hu and Ma, 2006; Hong et al., 2011). Overexpression of MIF1 was shown to reduce leaf size (Hong et al., 2011; Hu et al., 2011), a phenotype similar to that of pANT:HB33-SRDX plants.
Extensive works in different plant species have shown that GRF transcription factors are central regulators of leaf development. In Arabidopsis, loss of GRF expression results in smaller plants with decreased leaf size, while plants with higher expression levels of GRFs have larger leaves (Kim et al., 2003; Kim and Kende, 2004; Horiguchi et al., 2005; Kim and Lee, 2006; Liu et al., 2009; Gonzalez et al., 2010; Rodriguez et al., 2010; Wang et al., 2011a; Kim et al., 2012; Mecchia et al., 2012; Liang et al., 2013; Debernardi et al., 2014; Beltramino et al., 2018; Hou et al., 2019). Besides, the GRFs have been shown to promote growth in other species and organs. Most conspicuously, they regulate grain size in crops (Che et al., 2015; Duan et al., 2015; Hu et al., 2015; Li et al., 2016, 2018). In addition, rice (Oryza sativa) OsGRF4 has been shown to regulate genes involved in nitrogen assimilation (Li et al., 2018) and MYB61, which controls cellulose biogenesis (Gao et al., 2015). However, despite their wide range of functions described in the control of plant development, little is known about the interplay with other pathways. By performing a meta-analysis of the transcriptome of plants with different levels of the GRFs, we identified a connection to ZF-HD transcription factors. Activation of pGRF3:GRF3-GR by DEX revealed the induction of several ZF-HDs, including HB33, which prompted us to study whether some of the roles of the GRFs are achieved through the up-regulation of ZF-HDs. Moreover, HB33 was among the genes induced by AN3, a GRF-interacting factor (Vercruyssen et al., 2014). Furthermore, transcriptional reporter analysis and ChIP-qPCR studies confirmed that GRF3 directly activates HB33.
We found that a moderate increase or decrease in HB33 activity concomitantly affected leaf size through the control of cell number and size. In addition, the constitutive expression of HB33 through the UBQ10 promoter increased leaf longevity. The delay in the onset of senescence was observed in mild and strong transgenic plants, suggesting it was not strictly dependent on the previous stage of leaf development or the size of the organ.
Interestingly, the expression of HB33 from the ANT promoter, which is expressed early in leaf development (Schoof et al., 2000), uncoupled the effects in leaf size and senescence, causing an increase in leaf size without an obvious modification in leaf longevity. These results suggest that an extended period of HB33 expression during leaf development is required to increase leaf longevity. GRFs also affect leaf longevity; plants with increased expression of GRF3 (pGRF3:rGRF3) and GRF5 (35S:GRF5) had larger leaves that displayed delayed senescence (Debernardi et al., 2014; Vercruyssen et al., 2015). Similar to HB33, the effects of rGRF3 in leaf size and longevity can be uncoupled by the ANT promoter (Debernardi et al., 2014), indicating that both ZF-HDs and GRFs increase leaf longevity when expressed for an extended period during the progression of leaf development.
While the loss-of-function of HB33 did not cause any obvious development defects (Tan and Irish, 2006; Hu et al., 2008; Supplemental Figure S3), we tested whether it had a role in the developmental changes induced by high GRF expression such as in rGRF3. Crosses between rGRF3 and hb33 plants continued to produce large leaves as observed in rGRF3 plants, however, we noticed that the long leaf longevity typical of rGRF3 plants was decreased by the mutation in HB33, demonstrating that the activation of HB33 is responsible for the increased longevity of rGRF3 leaves.
Interestingly, cultivated rice varieties with mutations in the miR396-binding site of OsGRF4 developed large grains due to the excess of GRF4 activity, a phenotype caused by an increase in both cell number and size (Che et al., 2015; Duan et al., 2015; Hu et al., 2015; Li et al., 2016, 2018). We also observed that a mild increase in HB33 expression caused an increase in both cell numbers and size in leaves, and increased expression levels of ZF-HDs can modify seed size and development in Arabidopsis (Bueso et al., 2014). Therefore, it will be interesting to test whether there is a coordination between GRFs and ZF-HDs during the growth of other organs and in other species, especially crop grains.
The results presented here allowed us to connect a miRNA and two families of transcription factors during leaf development. In this model, under normal growing conditions, miR396 restricts GRF expression to proliferative tissues at the base of the leaf. GRF activity is necessary to control HB33 expression and likely the expression of other ZF-HDs. Impairing miR396 activity during leaf development, like in MIM396 or rGRF3 plants, results in a higher dosage with an extended expression pattern of the GRFs and, concomitantly, HB33. We speculate that modulating the activity of any of the components of this genetic module (miR396-GRFs-ZF-HDs) could be important for fine-tuning the number of cells and longevity of the leaves.
Materials and methods
Plant material and leaf analysis
Arabidopsis (Arabidopsis thaliana) ecotype Col-0 was used in the experiments. Plants were grown either on soil or on Murashige and Skoog (MS) medium (Sigma; http://www.sigmaaldrich.com) in long (16 h light/8 h dark) photoperiods at 23°C. Leaf areas were measured using the ImageJ program (https://imagej.net/ij/index.html) after dissection of individual leaves. The mutant hb33 (SALK_097388) was obtained from the Arabidopsis Biological Resource Center (ABRC). The multiple mutant grf1357 was kindly provided by professor Jeong Hoe Kim (Lee et al., 2018) and miR396ab knock out line by Ning Han (Hou et al., 2019).
Microscopy techniques
To obtain paradermal views of palisade cells, leaves were fixed with formalin acetic acid alcohol and cleared with chloral hydrate solution (200 g chloral hydrate, 20 g glycerol, and 50 ml dH2O) as described (Horiguchi et al., 2005). Palisade leaf cells were observed using differential interference contrast microscopy. The density of palisade cells per unit area was determined, and the area of the leaf blade was divided by this value to calculate the total number of palisade cells in the subepidermal layer. To determine the cell area, 20 palisade cells were measured in each leaf. Experiments were carried out in duplicate with 10 leaves.
Cloning and generation of transgenic lines
See Supplemental Table S3 for a detailed description of the constructs used in this study. For the analysis of expression pattern of HB33 and ANT, the reporter vectors were generated by cloning the GUS coding sequence downstream of HB33 promoter sequences in pCHF3 binary vector (Yin et al., 2002) or 5.2 kb of ANT promoter sequences in MX202 (Wu et al., 2003), respectively. The pHB33:HB33-SRDX and pANT:HB33-SRDX constructs were obtained by cloning 3.1 kb of the HB33 promoter or 5.2 kb of ANT promoter together with the HB33 coding sequence. Then, the SRDX repression domain was fused to the C-terminus of HB33 protein. mir396ab, MIM396, rGRF3, and rGRF3-GR lines were described before (Gonzalez et al., 2010; Rodriguez et al., 2010; Debernardi et al., 2014; Rodriguez et al., 2015; Beltramino et al., 2018; Hou et al., 2019).
Transcriptome meta-analysis
The microarray dataset was analyzed using a Z-score-based meta-analysis to identify GRF responsive genes as previously described (Busch et al., 2010). First, we calculated the Z-score for all genes in every dataset. Then we created a GRF regulation score for each gene by adding up the Z-scores from the datasets with increased GRF activity and subtracting the Z-scores from the datasets with down-regulated GRFs. An empirical P-value for the GRF regulation score was calculated by 10,000-fold random sampling of individual GRF regulation score values.
RT-qPCR analysis
RNA samples were extracted from inflorescence tissue using Tripure isolation reagent (Roche). Approximately 10 inflorescences were collected from each sample at 40 days after germination. The numbers of biological replicates are stated in the corresponding figure legends. Total RNA (1 µg) was treated with RQ1 RNase-free DNase (Promega). First-strand complementary DNA (cDNA) synthesis was performed using SuperScript III Reverse Transcriptase (Invitrogen). PCR was performed in a Mastercycler ep realplex thermal cycler (Eppendorf) using SYBR Green I (Roche) to monitor double-stranded DNA synthesis. The relative transcript level was determined for each sample and normalized using PROTEIN PHOSPHATASE 2 A (Czechowski et al., 2005). The normalization was performed as described previously (Livak and Schmittgen, 2001). Primer sequences are given in Supplemental Table S3.
GUS staining and GUS activity assays
To visualize reporter activity, transgenic plants harboring GUS reporters were subjected to GUS staining, as described (Donnelly et al., 1999). The tissue was collected in 90% (v/v) acetone on ice, and then incubated 20 min at room temperature. Acetone was removed by washing with staining solution without X-Gluc. The tissues were infiltrated in staining solution with X-Gluc for 30 min breaking the vacuum each 7 min. Samples were incubated for 4 h at 37°C and then stored in 70% (v/v) ethanol. GUS activity was determined by measuring 4-methylumbelliferone fluorometric quantity as previously described (Jefferson et al., 1987).
ChIP
ChIP experiments were performed as described by Kaufmann et al. (2010) with some modifications. The assay was performed using apices of 12-day-old seedlings of wild-type plants and pGRF3:GRF3-GFP expressing plants. Briefly, the apices were collected (0.5 g per sample) on ice, the tissue was fixed in 1% (v/v) formaldehyde, homogenized, and the nuclei isolated and lysed. Crosslinked chromatin was sonicated using a Bioruptor UCD-200 (Diagenode) water bath (30 s on/60 s off pulses: twelve times). The complexes were immunoprecipitated with 1 µl of anti-GFP antibody (ChIP Grade; Abcam ab290, lot GR76757-1) for 1 h at 4°C with gentle shaking, followed by incubation for 50 min at 4°C with 50 ml of Protein A agarose beads (Invitrogen). Immunoprecipitated DNA was then recovered and analyzed by qPCR. An aliquot of untreated sonicated chromatin was processed in parallel as the total input DNA control. Fold enrichment was calculated in comparison with the wild-type sample. The primers used in the ChIP experiments are listed in Supplemental Table S3.
Dark-induced leaf senescence
Fully expanded fourth or fifth leaves were detached and incubated for 4 days in Petri dishes with moistened cotton at 23°C in darkness. Chlorophyll and carotenoid content were determined as described by Porra (Porra, 2002) and Lichtenthaler and Wellburn (Lichtenthaler and Wellburn, 1983), respectively. The photochemical efficiency of Photosystem II (PSII) was deduced from the characteristics of chlorophyll fluorescence (Oh et al., 1997). Variable fluorescence intensity/maximum fluorescence intensity (Fv/Fm) corresponds to the photochemical efficiency of PSII (Oh et al., 1997).
Accession numbers
Sequence data from this article can be found in Supplemental Table S4.
Supplemental data
The following materials are available in the online version of this article.
Supplemental Figure S1. GUS expression analysis of the HB33 upstream regulatory region.
Supplemental Figure S2. Expression of HB33 in a mir396ab mutant.
Supplemental Figure S3. An hb33 mutant does not have obvious effects on Arabidopsis rosette development.
Supplemental Figure S4. Expression of pHB33:GUS and pANT:GUS.
Supplemental Figure S5. MIM396 delays plant senescence.
Supplemental Figure S6. Dark-induced senescence in mir396 and grf mutants.
Supplemental Table S1. Negative GRF responsive genes.
Supplemental Table S2. Positive GRF responsive genes.
Supplemental Table S3. Transgenic constructs used in this study.
Supplemental Table S4. Relevant gene identifiers and oligonucleotides used in this study.
Acknowledgments
We thank Jeong Hoe Kim for the grf1357 mutant line; Ning Han for the mir396ab knock out line; members of the J.F.P. laboratory for comments and discussions; and Diego Aguirre for plant technical assistance.
Funding
A.F., J.D., S.R., and D.L. were supported by fellowships from CONICET and J.F.P. and C.S. are members of the same Institution. Research is supported by grants from Agencia Nacional de Promoción Científica y Técnológica (PICT), CONICET (PUE 086) and ICGEB (CRP/ARG17-01).
References
Author notes
A.F. performed most of the experiments and analysis, participated in experimental design, and contributed the first draft. J.M.D. participated in the experimental design and performed experiments. S.R., D.L., and C.S. performed experiments, J.F.P. designed the research, supervised most of the co-authors, and wrote the paper with A.F. All authors reviewed the manuscript and contributed corrections and suggestions.
The author responsible for distribution of materials integral to the findings presented in this article in accordance with the policy described in the Instructions for Authors (https://dbpia.nl.go.kr/plphys/pages/General-Instructions) is Javier F. Palatnik.
Conflict of interest statement. None declared.