-
PDF
- Split View
-
Views
-
Cite
Cite
Hongfeng Wang, Zhichao Lu, Yiteng Xu, Jing Zhang, Lu Han, Maofeng Chai, Zeng-Yu Wang, Xianpeng Yang, Shiyou Lu, Jianhua Tong, Langtao Xiao, Jiangqi Wen, Kirankumar S Mysore, Chuanen Zhou, Roles of very long-chain fatty acids in compound leaf patterning in Medicago truncatula, Plant Physiology, Volume 191, Issue 3, March 2023, Pages 1751–1770, https://doi.org/10.1093/plphys/kiad006
- Share Icon Share
Abstract
Plant cuticles are composed of hydrophobic cuticular waxes and cutin. Very long-chain fatty acids (VLCFAs) are components of epidermal waxes and the plasma membrane and are involved in organ morphogenesis. By screening a barrelclover (Medicago truncatula) mutant population tagged by the transposable element of tobacco (Nicotiana tabacum) cell type1 (Tnt1), we identified two types of mutants with unopened flower phenotypes, named unopened flower1 (uof1) and uof2. Both UOF1 and UOF2 encode enzymes that are involved in the biosynthesis of VLCFAs and cuticular wax. Comparative analysis of the mutants indicated that the mutation in UOF1, but not UOF2, leads to the increased number of leaflets in M. truncatula. UOF1 was specifically expressed in the outermost cell layer (L1) of the shoot apical meristem (SAM) and leaf primordia. The uof1 mutants displayed defects in VLCFA-mediated plasma membrane integrity, resulting in the disordered localization of the PIN-FORMED1 (PIN1) ortholog SMOOTH LEAF MARGIN1 (SLM1) in M. truncatula. Our work demonstrates that the UOF1-mediated biosynthesis of VLCFAs in L1 is critical for compound leaf patterning, which is associated with the polarization of the auxin efflux carrier in M. truncatula.
Introduction
The shoot apical meristem (SAM) is a structure at the tip of the shoot that is responsible for generating almost all of the above-ground tissue of the plant, such as leaves and flowers (Furner I and Pumfrey, 1992). According to the tunica-corpus theory, the SAM is divided into three distinct cell layers: the L1 epidermal, L2 subepidermal, and L3 layers (Schmidt, 1924; Sussex, 1989). The L1 epidermal is a composite tissue that forms the outer layers of plant organs, playing an important role in connecting the internal genetic factors and external environment signals (Barton, 2010; Perales and Reddy, 2012). During leaf development, cells of the L1 epidermal of the SAM divide anticlinally and then differentiate into pavement cells, guard cells, and trichomes.
A characteristic feature of the epidermis is that it is covered by the cuticle, a barrier to protect organisms away from stress (Lolle and Pruitt, 1999; Kunst and Samuels, 2009). In plants, the cuticle is composed of hydrophobic cuticular waxes and cutin (PostBeittenmiller, 1996; Samuels et al., 2008). Cuticular waxes are synthesized predominantly in epidermal cells and are mainly composed of very long-chain fatty acids (VLCFAs) and their derivatives, such as wax esters, alkanes, and aldehydes (Li-Beisson et al., 2013; Yeats and Rose, 2013). The C16- and C18-CoAs synthesized by plastids are elongated into VLCFAs in the endoplasmic reticulum (ER) membrane by a fatty acid elongase (FAE) complex, which is composed of β-ketoacyl-CoA synthase (KCS), β-ketoacyl-CoA reductase (KCR), β-hydroxy acyl-CoA dehydratase (HCD), and enoyl-CoA reductase (ECR) (Kunst and Samuels, 2009; Bernard and Joubes, 2013; Hegebarth and Jetter, 2017). The rate-limiting step of VLCFAs biosynthesis is a condensation of a malonyl-CoA unit to the acyl-CoA that is catalyzed by KCS (Lee and Suh, 2013; Yeats and Rose, 2013). In Arabidopsis (Arabidopsis thaliana), 21 KCS encoding genes are annotated and they have different or similar expression patterns and substrate specificities (Millar and Kunst, 1997; Trenkamp et al., 2004; Joubes et al., 2008). Among the KCS, mutation of the FIDDLEHEAD (FDH) leads to the fusion of leaves and floral organs (Lolle et al., 1992; Yephremov et al., 1999; Pruitt et al., 2000). In Antirrhinum (Antirrhinum majus), organ fusion phenotype can be induced by chloroacetamide, an inhibitor of β-ketoacyl-CoA synthases (Efremova et al., 2004). Furthermore, the VLCFAs are modified into primary alcohols by the alcohol-forming pathway and into ketones and secondary alcohols by the alkane-forming pathway, which are also important components of sphingolipids, seed triacylglycerols, and suberin (Li-Beisson et al., 2013). Cutin, the skeleton of the cuticle, is a plant-specific biopolymer that consists of C16 and/or C18 long-chain fatty acids and glycerol (Pollard et al., 2008; Dominguez et al., 2011). The formation of the extracellular cutin polymer is divided into three sequential steps. The first step is to synthesize cutin monomers in ER by the plastid-derived C16 and C18 fatty acids. Enzymes involved in this process are cytochrome P450 CYP77A6, long-chain acyl-CoA-synthetases (LACS), sn-2 glycerol phosphatase-acyltransferase (GPAT), ABERRANT INDUCTION OF TYPE THREE1 (ATT1), and HOTHEAD (HTH) (Shockey et al., 2002; Schnurr et al., 2004; Tang et al., 2007; Li-Beisson et al., 2009; Lu et al., 2009; Sauveplane et al., 2009; Pulsifer et al., 2012). Among them, ATT1 encodes CYP86A2, a cytochrome P450 monooxygenase catalyzing fatty acid oxidation (Xiao et al., 2004). The att1 mutant displays a loosely structured cuticle membrane, indicating CYP86A2 functions in cuticle development. Finally, the cutin monomers are exported from the ER to the nascent cuticular membrane through the lipid transfer proteins (LTP) and the ATP-binding cassette G (ABCG) transporters (Bird et al., 2007; Debono et al., 2009; Bessire et al., 2011; Kim et al., 2012), and polymerization is catalyzed by the α/β-hydrolase BODYGUARD (BDG) and acyltransferase DEFECTIVE IN CUTICULAR RIDGES (DCR) (Kurdyukov et al., 2006; Panikashvili et al., 2009).
Auxin regulates many developmental processes in plants. During leaf development, auxin coordinates the phyllotaxis of leaf initiation from the SAM and determines the location of serrations and the initiation of leaflets from the margin of leaf primordia (Barkoulas et al., 2008; Koenig et al., 2009; Shwartz et al., 2016). Auxin transport at the shoot apical is mediated by the PIN-FORMED (PIN) family members (Shi et al., 2017). PIN1 is one of the auxin transporters with a polar localization within the SAM responsible for forming auxin gradients in the L1 that in turn control the initiation of leaf primordia and the formation of leaf serrations (Benková et al., 2003; Xiong and Jiao, 2019). The PIN1 polar targeting and cell polarity proliferation/expansion depend largely on the composition of the plasma membrane, such as VLCFAs and their derivatives. PASTICCINO1 (PAS1) encodes a large molecular weight member of the immunophilin-type binding protein family that is required for VLCFA synthesis (Vittorioso et al., 1998). Loss of PAS1 function results in the reduction of sphingolipids levels and the mistargeting of the PIN1, resulting in the local alteration of polar auxin distribution in the embryo apex cells (Roudier et al., 2010). STEROL METHYLTRANSFERASE1 (SMT1) encodes an enzyme that is required for appropriate sterol levels (Diener et al., 2000). SMT1 is involved in the C24 alkylation of sterols, and the columella cells of the smt1 mutant display defective apical-basal polarity and disturbed localization of the PIN proteins (Schrick et al., 2002; Willemsen, 2003). Furthermore, the rice (Oryza sativa) ONION1 (ONI1), an ortholog of FDH, is also required for VLCFAs synthesis and correct fatty acid composition, and loss of ONI1 function mutants show the abnormal L1, causing altered auxin distribution in the shoot (Ito et al., 2011; Takasugi and Ito, 2011).
Although much progress on cuticle formation and its functions on plant development has been made in different species, however, the roles of cuticle components, especially VLCFAs and their derivatives, in compound leaf patterning are still unclear. In this study, we identified two types of mutants with unopened flower phenotype in barrelclover (Medicago truncatula), named unopened flower1 (uof1) and uof2. Both UOF1 and UOF2 encoded enzymes that were involved in the biosynthesis of VLCFAs and the formation of the cuticle. Both uof1 and uof2 had a reduced amount of VLCFAs and cuticular wax, however, only the leaves of uof1 mutants showed an increased number of leaflets. Comparison of the expression pattern between UOF1 and UOF2 indicated that UOF1, instead of UOF2, was specifically expressed in epidermal cells of different organs. We presented a model for the roles of UOF1-mediated biosynthesis of VLCFAs in L1 in regulation of elaboration of compound leaves, which is associated with proper polar localization of PIN1 ortholog in M. truncatula.
Results
Identification of two types of unopened flower mutants in M. truncatula
To identify regulators that control compound leaf morphogenesis and flower development in legumes, about 22,000 independent lines of the Tnt1-tagged M. truncatula mutant population (Tadege et al., 2008) were screened. Two types of mutants with similar unopened flower phenotypes were identified. These mutants were named unopened flower1 (uof1) and uof2, respectively. The flower in wild-type had normally expanded petals at the early development stage (Figure 1, A and B; Supplemental Figure 1A), however, the corolla of uof1-1 flowers was tightly enclosed by the enlarged sepals, preventing petal expansion, and the stigma expands outward (Figure 1, E and F; Supplemental Figure 1B). In addition, the petals of uof2-1 were also manacled by sepals resulting in the closed flower and the exposed stigma (Figure 1, I and J; Supplemental Figure 1C). At the late development stage, wild-type plants could develop seed pods (Figure 1, C and D). In both uof1-1 and uof2-1 mutants, one or two pod spirals were observed, but they normally dropped later due to lack of pollination, leading to the sterility in mutant plants (Figure 1, G, H, K, and L).

Phenotypic analysis of the uof1-1 and uof2-1 flowers. A–C, Flowers of wild-type plants at different developmental stages. Early stage flower (A), mature flower (B), and pod (C) of wild-type plants are shown. D, SEM analysis of the pod of wild-type plant. E–G, Flowers of uof1-1 mutants. Early stage flower (E), mature flower (F), and late stage flower (G) of uof1-1 mutants are shown. Arrows in (F and G) indicate the outward expanded stigmas in uof1-1 mutants. H, SEM analysis of the late stage flower of uof1-1 mutants. The arrow in (H) indicates the enclosed sepals of the uof1-1 flower. I–K, Flowers of uof2-1 mutants. Early stage flower (I), mature flower (J), and late stage flower (K) of uof2-1 mutants are shown. Arrows in (J and K) indicate the outward expanded stigmas in uof2-1 mutants. L, SEM analysis of the late stage flower of uof2-1 mutants. The arrow in (L) indicates the enclosed sepals of the uof2-1 flower. Bars, 2 mm in (A–L).
To further investigate the reason for sterility in uof1 and uof2 flowers, the developmental processes of floral organ primordia between the wild-type and mutants were compared by a scanning electron microscope (SEM). At stage 6, the sepal, petal, and stamen primordia were completely formed and the central carpel became visible in the wild-type, uof1-1, and uof2-1 flowers, indicating that the initiation of the primordia of the floral organs is normal in both wild-type and mutants (Supplemental Figure 1, D–F). Moreover, pollen staining revealed that pollens in mutants were fertile, similar to those of the wild-type (Supplemental Figure 1, G–I). These observations suggest that unopened flowers induced by the restraint of sepals in uof1 and uof2 lead to the increased physical distance between stigma and anthers, resulting in the sterility.
Molecular cloning and genetic complementation analysis
To identify the genes responsible for the unopened flower phenotype of uof1 and uof2 mutants, we first analyzed the flanking sequences derived from the uof1 mutants as described (Tadege et al., 2008), and found that one sequence was associated with the unopened flower phenotype of uof1 mutants. Then, we used this sequence to align in the M. truncatula genome database. A gene with a full-length genomic sequence of 3349 nucleotides was obtained (Figure 2A). Further analysis showed that the Tnt1 insertions were located at the second exon in uof1-1, uof1-2, and uof1-3, and at the third exon in uof1-4 (Figure 2A). Subsequent co-segregation analyses on the progeny of self-pollinated heterozygous uof1-1/+ and uof1-2/+ plants were performed, respectively (Supplemental Figure 2, A–D). The ratio between wild-type-like plants and uof1 mutants was approximately 3:1 (Supplemental Figure 2, A and B), suggesting that the uof1 mutant phenotype was caused by the mutation of a single recessive gene. Furthermore, genomic PCR and reverse transcription PCR (RT-PCR) analysis showed that homozygous insertion of Tnt1 resulted in the interruption of UOF1 expression (Figure 2, B and C). Phylogenetic analysis revealed that UOF1, the ortholog of Arabidopsis KCS10/FDH and a rate-limiting enzyme of VLCFAs synthesis, was required for the correct formation of the cuticle (Supplemental Figures 3 and 4). For genetic complementation, a vector containing the UOF1 genomic sequence and its native promoter was introduced into uof1-1 mutants using Agrobacterium tumefaciens-mediated transformation. Phenotypic analysis indicated that complementary UOF1 expression fully rescued the unopened flower phenotype of uof1-1 mutants (Figure 2, G and H). These data indicate that the loss-of-function mutation of UOF1 is responsible for the unopened flower phenotype of the uof1 mutants.
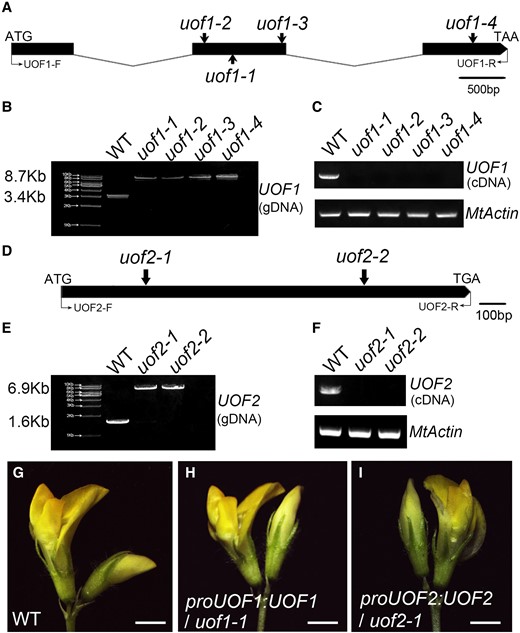
Molecular cloning of the UOF1 and UOF2 genes and genetic complementation analysis. A, The gene structure of UOF1 and Tnt1 insertion sites of different uof1 alleles. Black boxes represent exons and lines represent introns. The start codon (ATG) and stop codon (TAA) are indicated. B, PCR amplification of the UOF1 genomic sequences from wild-type and different uof1 alleles using primers UOF1-F/UOF1-R. A single Tnt1 insertion (∼5.3 kb) was detected in each uof1 mutant line. C, RT-PCR analysis of UOF1 transcripts in the wild-type and different uof1 alleles using primers UOF1-F/UOF1-R. MtActin was used as the control. D, The gene structure of UOF2 and Tnt1 insertion sites of different uof2 alleles. The start codon (ATG) and stop codon (TGA) are indicated. E, PCR amplification of the UOF2 genomic sequences from wild-type and different uof2 alleles using primers UOF2-F/UOF2-R. A single Tnt1 insertion (∼5.3 kb) was detected in each uof2 mutant line. F, RT-PCR analysis of UOF2 transcripts in wild-type and different uof2 alleles using primers UOF2-F/UOF2-R. G, Flower of the wild-type plants. H and I, Flowers of uof1-1 and uof2-1 mutants complemented with proUOF1:UOF1 (H) and proUOF2:UOF2 (I), respectively. Bars, 2 mm in (G–I).
The UOF2 gene was also cloned by PCR-based genotyping of flanking sequence tags in the segregating populations. Alignment of the coding sequence and full-length genomic sequence of the UOF2 gene indicated that UOF2 was composed of only one exon, and the Tnt1 retrotransposons were inserted at positions 243 and 1151 bp of uof2-1 and uof2-2, respectively (Figure 2D). Co-segregation analyses showed that the uof2 mutant phenotype was also caused by a single recessive nuclear gene mutation (Supplemental Figure 2, E–H). Genomic PCR and RT-PCR results revealed that all uof2 mutant lines were knockout alleles of the UOF2 gene due to Tnt1 insertions (Figure 2, E and F). Phylogenetic analysis showed that UOF2 encodes a member of the CYP86A subfamily of cytochrome P450-dependent fatty acid ω-hydroxylase, and it is homologous to Arabidopsis CYP86A2/ATT1, implying its function in cuticle formation (Supplemental Figures 5 and 6). Further genetic complementation confirmed that complementary UOF2 expression fully rescued the unopened flower phenotype of uof2-1 mutants (Figure 2I).
The defects in wax load and cuticle permeability in the uof mutants
To investigate whether UOF1 and UOF2 play roles in the development of cuticles, we examined the epidermal phenotype of uof1-1 and uof2-1 mutants. Firstly, the toluidine blue (TB) staining test was executed to assay the cuticle permeability. The results showed that the flowers and leaves of uof1-1 and uof2-1 mutants displayed increased staining compared with that in wild-type plants (Figure 3, A–F), indicating that cuticle permeability was increased in uof1-1 and uof2-1 mutants. Secondly, the leaf surface was examined by SEM to check the possible changes in wax. Compared with the wild-type leaves, the epidermal wax crystals load of uof1-1 and uof2-1 mutants was substantially reduced (Figure 3, G–I). In agreement with this notion, the leaves of uof1-1 and uof2-1 mutants showed a more rapid water loss than those of the wild-type (Figure 3J). To further characterize the defects in epidermal wax, the total wax load and wax constituents were analyzed. Compared with the wild-type, the total amount of wax on leaves was significantly decreased by 23% in uof1-1 and 55% in uof2-1 (Figure 3K). Most of the changes in the amount of leaf wax in uof1-1 and uof2-1 were caused by the reduction of C30 and C28 alcohols, and C30 aldehydes (Figure 3M), which were the dominant wax components throughout leaf development. However, the loss-of-function of UOF1 and UOF2 resulted in a slight increase in the cutin amount (Figure 3, L and N). These observations demonstrate that the defects in cuticle in uof1 and uof2 mutants are mainly caused by the loss of very long-chain alcohols and aldehydes.
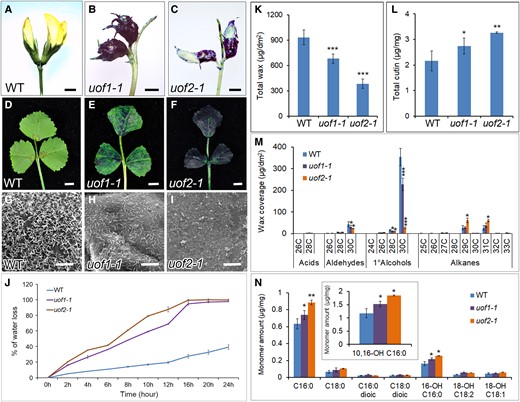
Mutation of UOF1 and UOF2 leads to defects in cuticle development. A–F, Toluidine blue stained flowers (A–C) and leaves (D–F) of wild-type, uof1-1, and uof2-1 plants. Bars, 2 mm in (A–C) and 5 mm in (D–F). G–I, SEM analyses of the cuticular wax crystals in leaves of wild-type (G), uof1-1 (H), and uof2-1 (I) plants. Bars, 10 μm in (G–I). J, Water loss rates of wild-type, uof1-1, and uof2-1 leaves were measured. Values are the mean and SD of three replicate assays. K and L, The contents of total wax (K) and cutin (L) in the leaves of wild-type, uof1-1, and uof2-1 plants. Values are the means and SD of three biological replicates. M and N, Cuticular wax (M) and fatty acid (N) composition in leaves of wild-type, uof1-1, and uof2-1 plants. Values are the means and SD of three biological replicates. *P < 0.05; **P < 0.01; ***P < 0.001. The two-sided Student's t test was used to estimate if the difference is significant.
In addition, it was reported that many mutants lacking epidermal wax exhibit organ adhesion or fusion in the shoot (Yephremov et al., 1999; Wellesen et al., 2001; Ito et al., 2011; Panikashvili et al., 2011). Morphological analysis showed that organ adhesions occur between flowers and leaves and between leaflets in both uof1 and uof2 mutants when the leaves and flowers just developed in the apical shoot tips (Supplemental Figure 7). However, the adhesion between organs was recovered along with the plant growth. These results indicate that UOF1 and UOF2 play conserved roles in regulating leaf and floral organ separation.
UOF1, but not UOF2, is required for compound leaf patterning and leaf margin formation
In the wild-type, all adult leaves were in trifoliate form with a terminal leaflet and two lateral leaflets (Figure 4A). All four alleles of UOF1 showed a substantial increase in leaflets number that about 50% of the adult leaves produced one to three ectopic leaflets (Figure 4, B–E and G; Supplemental Figure 8, A–C and E). Phenotypic observation confirmed that complementary UOF1 expression fully rescued the defects in leaf patterning and leaf margin of uof1-1 mutant (Supplemental Figure 8, D and E). However, the compound leaf patterning in uof2-1 mutants was unchanged (Figure 4, F and G). Moreover, the leaves of the wild-type and uof2-1 mutants formed a serrated leaf margin (Figure 4, H–J and N–P), but the leaf margin of uof1 mutants was relatively smooth (Figure 4, K–M; Supplemental Figure 8, F–H). These observations suggest that loss-of-function in UOF1 affects the development of both compound leaf and leaf margin serrations. To investigate the genetic relationship between UOF1 and UOF2, we generated the uof1-1 uof2-1 double mutant by crossing. The leaf type proportion of uof1-1 uof2-1 was similar to that of uof1-1 mutant (Supplemental Figure 8E). In some cases, the compound leaf patterning of uof1-1 uof2-1 was the same as that of the uof1-1 mutant (Supplemental Figure 8, J–L). Moreover, the leaf margin and flowers in uof1-1 uof2-1 double mutants resembled those of uof1-1 (Supplemental Figure 8, I and M–O). These observations indicate that uof1 was genetically epistatic to uof2 in both leaf and flower development.
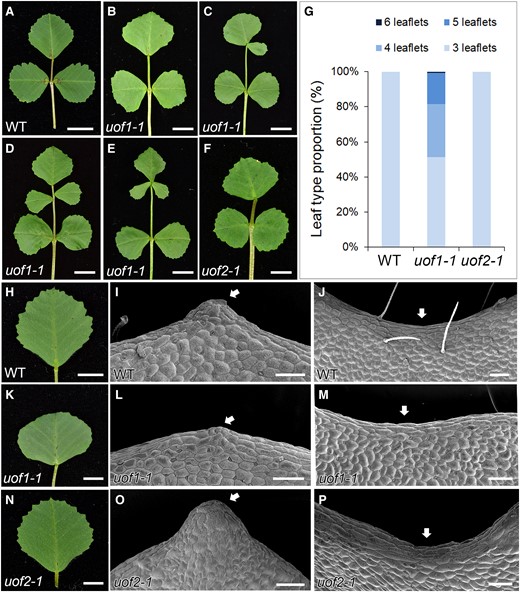
The uof1-1 mutants show defects in compound leaf development. Adult leaves of wild-type (A), uof1-1 (B–E), and uof2-1 (F) plants. Leaflet number is substantially increased in some leaves of uof1-1 mutant (C–E). Bars, 1 cm in (A–F). G, Leaf type proportion in wild-type (n = 150), uof1-1 (n = 132), and uof2-1 (n = 125) plants. Development of leaf margins in wild-type (H–J), uof1-1 (K–M), and uof2-1 (N–P). Observations of marginal cells at the teeth tips (I, L, and O) and leaf sinus (J, M, and P) in wild-type (I and J), uof1-1 (L and M), and uof2-1 (O and P) by SEM. Arrows indicate the relatively smooth leaf margin serrations and sinus in the uof1-1 mutant (L and M) compared with the wild-type (I and J) and uof2-1 mutant (O and P). Bars, 5 mm in (H, K, and N) and 100 µm in (I–J, L–M, and O–P).
Expression patterns of UOF1 and UOF2
To explore the possible reason for the distinct compound leaf patterning between uof1 and uof2 mutants, the expression patterns of UOF1 and UOF2 were compared. Reverse transcription quantitative PCR (RT-qPCR) data showed that UOF1 was expressed at higher levels than UOF2 in most organs (Figure 5A; Supplemental Figure 9A). To analyze the expression levels in more detail, the proUOF1:GUS and proUOF2:GUS transgenic plants were obtained. Consistent with the RT-qPCR results, UOF1 and UOF2 showed a similar expression pattern, in which GUS signals were detected in sepals of flowers and whole leaves (Figure 5, B–E). To gain better spatial expression patterns of UOF1 and UOF2, RNA in situ hybridization was performed on the shoot apex of wild-type plants. The data showed that UOF1 transcripts were specifically expressed in the L1 of SAM, emerging leaf primordia, and floral meristem (Figure 5, F and G). However, UOF2 transcripts were detected in the center of SAM and floral meristem with relatively low expression levels (Figure 5, H and I). As negative controls, the sense probes did not give any hybridization signals (Figure 5, J and K). To further confirm the L1-specific expression pattern of UOF1, wild-type plants transformed with the proUOF1:GFP reporter were obtained. GFP signals were detected not only in the L1 of SAM and leaf primordia but also in developing leaves and leaf margin serrations (Figure 5, L–P). Strong GFP signals were also detected in the epidermis of floral primordia, anthers, and seeds (Supplemental Figure 9, B–G). These observations demonstrate that UOF1 and UOF2 display the distinct expression patterns, implying that the specific transcription of UOF1 in the L1 may be the key to the formation of compound leaf patterning.
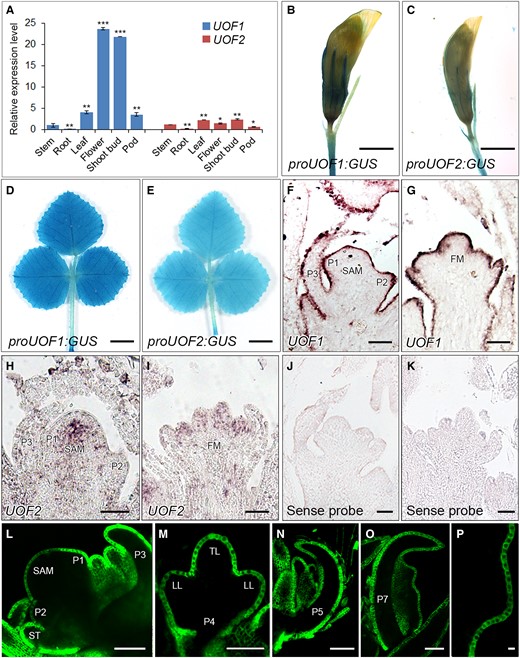
The expression patterns of UOF1 and UOF2 genes. A, The relative expression levels of UOF1 and UOF2 genes in different wild-type tissues. Values are the means and SD of three biological replicates; *P < 0.05; **P < 0.01; ***P < 0.001. The two-sided Student's t test was used to estimate if the difference is significant. B–E, Promoter-GUS fusion studies of UOF1 and UOF2 expression in transgenic plants. UOF1 and UOF2 promoters driven GUS were expressed in the flowers (B and C) and adult leaves (D and E). Bars, 2 mm in (B and C) and 5 mm in (D and E). F–I, The expression patterns of UOF1 (F and G) and UOF2 (H and I) were detected by RNA in situ hybridization. SAM, shoot apical meristem; P, leaf primordium; FM, floral meristem. J, K, The sense probes were hybridized and used as control. Bars, 50 μm in (F–K). L–P, Promoter-GFP fusion study of the UOF1 expression pattern in transgenic plants. GFP signals were specifically localized to the plasma membrane of epidermal cells of SAM and leaf primordia (L–O) and leaf margin serration cells (P). ST, stipule primordium; TL, terminal leaflet primordium; LL, lateral leaflet primordia. Bars, 50 μm in (L–P).
The expression pattern of SMOOTH LEAF MARGIN1 (SLM1) is unchanged in uof1
Our previous study showed that SLM1, the PIN1 ortholog in M. truncatula, was apically localized in the L1 of the SAM and leaf primordia (Zhou et al., 2011). In addition, loss-of-function of SLM1 led to increased leaflet number and smoothed leaf margin, which was also observed in uof1 mutant (Figure 6, A–C). To investigate the possible relationship between UOF1 and SLM1 gene, RT-qPCR was performed to analyze the expression of SLM1. Compared with the wild-type, the expression level of SLM1 was unchanged in uof1-1 mutants (Figure 6D). To further investigate whether the leaf developmental defects in uof1-1 were caused by the disruption of SLM1 expression pattern, the proSLM1:GUS construction was introduced into wild-type and uof1-1 plants, respectively. However, GUS staining showed the similar expression patterns of SLM1 between wild-type and uof1-1 mutants (Figure 6, E and F). Then, RNA in situ hybridization was performed in the shoot apex of wild-type and uof1-1 mutants. In the wild-type, SLM1 mRNA was detected in SAM at the sites where leaf primordia gave rise and in the developing leaf primordia (Figure 6, G and H). The uof1-1 mutant showed the same SLM1 expression pattern as those in the wild-type (Figure 6, I and J). As a negative control, the sense SLM1 probe did not give any hybridization signal (Figure 6K). These results indicate that the mutation of UOF1 does not change the expression level and pattern of SLM1.
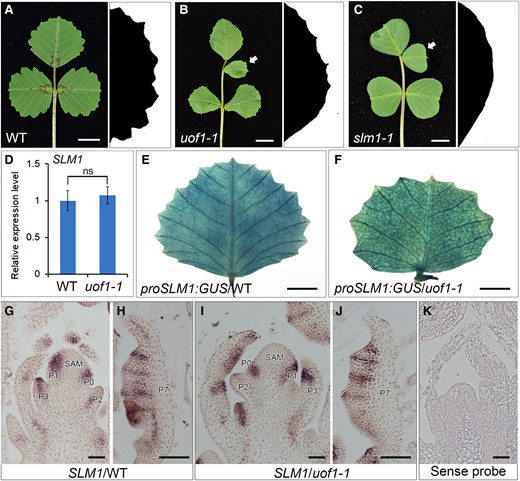
Developmental defects in uof1 resemble those in slm1. A–C, Leaf and leaf margin phenotypes of wild-type (A), uof1-1 (B), and slm1-1 (C) plants. Compared with the wild-type leaf (A), the uof1-1 and slm1-1 mutant exhibit similar defects in compound leaf patterning (B and C; left) and leaf margin development (B and C; right). White arrows in (B and C) indicate the ectopic leaflets in uof1-1 and slm1-1 mutants. D, The relative expression levels of SLM1 in wild-type and uof1-1 leaves were detected by RT-qPCR. There was no significant difference in SLM1 expression between wild-type and uof1-1 mutants. Values are the means and SD of three biological replicates. E and F, The expression patterns of SLM1 in fully expanded leaves of the wild-type (E) and uof1-1 mutants (F), as determined by detecting the proSLM1:GUS activity. G–J, The expression patterns of SLM1 in shoot buds (G and I) and leaf primordia (H and J) of the wild-type and uof1-1 mutants, as determined by RNA in situ hybridization. SAM, shoot apical meristem; P, leaf primordium. K, The sense probe of SLM1 was hybridized and used as control. Bars, 1 cm in (A–C), 5 mm in (E and F), and 50 μm in (G–K).
Global changes in gene expression in uof1-1 mutant
To further explore the roles of UOF1 in the formation of compound leaf patterning, RNA-sequencing analyses were performed. Comparing the whole transcriptome profiles between wild-type and uof1-1 mutants, a total of 930 differentially expressed genes (DEGs) (ratio ≥ 2) were identified, among which 679 genes were up-regulated and 251 were down-regulated in the leaves of uof1-1 mutants relative to the wild-type (Figure 7B; Supplemental Table 2). Gene ontology (GO) analysis revealed that the changed genes were highly enriched for biological processes associated with metabolic processes, cellular processes, single-organism processes, response to stimulus, and localization, and were also enriched for cellular component and molecular function categories related to cell part, membrane part, and catalytic activity (Figure 7A; Supplemental Figure 10).
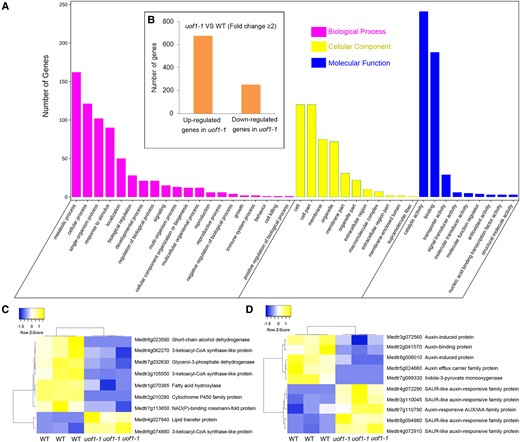
Comparison of wild-type and uof1-1 transcriptomes. A, GO enrichment of genes with changed expression in shoot buds of uof1-1 mutants. B, The number of differentially expressed genes (DEGs, ≥2-fold change) of uof1-1 compared with wild-type. C, Heatmap of fatty acids biosynthesis related DEGs. D, Heatmap of auxin biosynthesis, signaling, and response-related DEGs.
Many down-regulated genes in the uof1-1 mutants encode enzymes that were involved in the biosynthesis of VLCFAs and their derivatives, and membrane components (Figure 7C). In addition to VLCFAs, auxin biosynthesis and auxin-induced genes were also substantially down-regulated in the uof1-1 mutants (Figure 7D). These results suggest that VLCFA-mediated plasma membrane integrity and auxin content may decrease in uof1 mutants.
PIN1 localization is changed in uof1 mutant
As UOF1 was specifically expressed in the L1 cells and involved in the synthesis of VLCFAs which were also major components of the plasma membrane, we hypothesized that the plasma membrane in L1 was defective. The Arabidopsis PLASMA MEMBRANE INTRINSIC PROTEIN2A (AtPIP2A) is a member of the plasma membrane intrinsic protein subfamily and specifically localizes to the plasma membrane (Verdoucq et al., 2008; Wudick et al., 2015; Yoo et al., 2016; Byrt et al., 2017). To verify this, the marker 35S:AtPIP2A-GFP (Luo and Nakata, 2012) was introduced into wild-type and uof1-1 mutants. In wild-type, the leaf epidermal cells and margin cells showed continuous GFP signals in the plasma membrane (Figure 8, A and C). However, an intermittent GFP signal was presented in the uof1-1 mutants (Figure 8, B and D), indicating that the integrity of the plasma membrane of L1 is largely defective in the uof1-1 mutants. Furthermore, SEM analysis showed that the epidermal cells of leaves were indistinguishable between uof1-1 and wild-type (Supplemental Figure 11), suggesting that the intermittent GFP signal in the uof1-1 was not caused by leaf morphological changes. These observations indicate that loss of UOF1 function leads to defects in plasma membrane integrity in L1 cells.
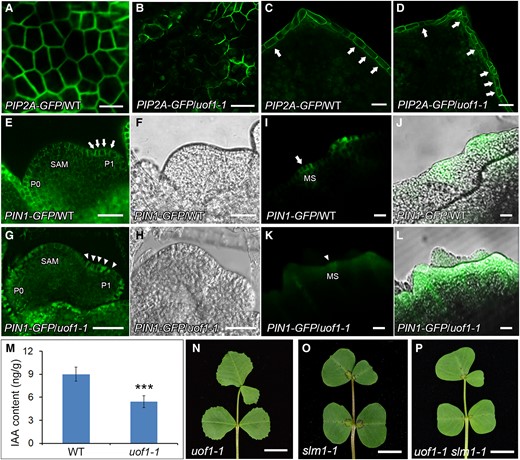
The localization of PIN1/SLM1 in wild-type and uof1 mutants. A–D, The integrity of the plasma membrane of leaf epidermal cells (A and B) and leaf marginal cells (C and D) of wild-type and uof1-1 mutants were analyzed, as determined by detecting the 35S:AtPIP2A-GFP marker. Arrows in (C) mark the continuous GFP signal in leaf marginal cells of wild-type, and in (D) mark the intermittent GFP signal in leaf marginal cells of uof1-1 mutants. Bars, 50 μm in (A–D). E–L, Distribution of the proAtPIN1:AtPIN1-GFP marker (green signal) in leaf primordia (E and G) and leaf margin serrations (I and K) of wild-type and uof1-1 mutants. Arrows mark the polar localization of PIN1 in leaf primordia (E) and leaf margin serration (I) of wild-type, while arrowheads mark the disturbed polar localization of PIN1 in leaf primordia (G) and leaf margin serration (K) of the uof1-1 mutants. The bright fields of SAM and leaf primordial of (E and G) are shown in (F and H), the merged images between GFP signals and bright fields of leaf margin serrations are shown in (J and L). SAM, shoot apical meristem; P, leaf primordium; MS, leaf margin serration. Bars, 50 μm in (E–H) and 100 μm in (I–L). M, The concentration of free indole-3-acetic acid (IAA) in 4-week-old leaves of wild-type and uof1-1 mutants. Values are the means and SD of three biological replicates; ***P < 0.001. The two-sided Student's t test was used to estimate if the difference is significant. N–P, Leaves of uof1-1 (N), slm1-1 (O), and uof1-1 slm1-1 double mutant (P). Bars, 1 cm in (N–P).
It was reported that polar localized PIN1-mediated auxin maxima in L1 are critical for the proper initiation of leaf primordia and the formation of leaf serrations (Reinhardt et al., 2003; Heisler et al., 2005; de Reuille et al., 2006). Our previous study showed that SLM1 is functionally equal to AtPIN1 (Zhou et al., 2011). Therefore, the proAtPIN1:AtPIN1-GFP reporter was introduced into wild-type and uof1-1 mutants to investigate whether the defective plasma membrane affected the polar localization of PIN1. In wild-type, PIN1 was apically localized at the L1 of the SAM and leaf primordia (Figure 8, E and F). However, the polar localization of PIN1 was disturbed in uof1-1 (Figure 8, G and H). Such defective localization of PIN1 was also observed in the marginal cells at the tip of serrations in uof1-1 leaves (Figure 8, I–L). These results demonstrate that the mutation of UOF1 leads to the defective in plasma membrane, resulting in the disordered polar localization of PIN1. To further investigate the role of auxin in uof1 mutant, the endogenous auxin level was determined. The free indole-3-acetic acid (IAA) concentration of the uof1-1 mutants was significantly lower than that of the wild-type plants (Figure 8M). In addition, genetic interaction analysis showed that the leaf patterning of uof1-1 slm1-1 double mutants was similar to those of slm1 (Figure 8, N–P), suggesting that slm1 is epistatic to uof1 in terms of leaf complexity. However, the uof1-1 slm1-1 double mutants still exhibited the organ adhesion and the defects in cuticular wax (Supplemental Figure 12), indicating that disruption of SLM1 does not change the abnormal wax biosynthesis in uof1-1.
SINGLE LEAFLET1 (SGL1) and class I MtKNOX (MtKNOXI) in L1 play limited roles in the regulation of the indeterminacy during leaf formation
Previous studies showed that the FLORICAULA (FLO)/LEAFY (LFY) putative ortholog SGL1 functioned in place of KNOXI to regulate compound leaf development in M. truncatula (Champagne et al., 2007; Wang et al., 2008). SGL1 was expressed in the SAM and emerging leaf primordia, and the leaves of sgl1 mutants were simple rather than compound (Figure 9, A and B; Wang et al., 2008). To investigate the genetic relationship between UOF1 and SGL1, the uof1-1 was crossed with the sgl1-1 mutant. Similar to sgl1-1, the uof1-1 sgl1-1 double mutant formed simple leaves (Figure 9C), indicating that sgl1 was genetically epistatic to uof1 in the regulation of compound leaf development.
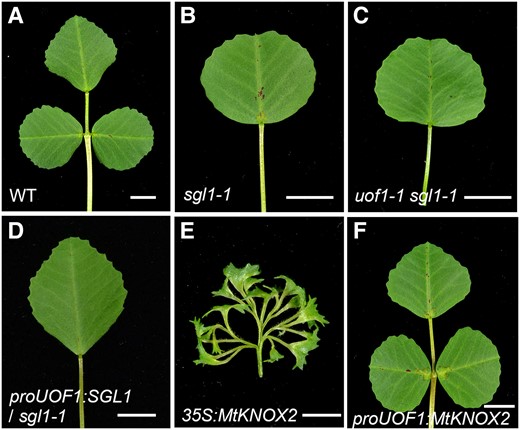
The function of the L1 in compound leaf patterning. Leaves of wild-type (A), sgl1-1 (B), uof1-1 sgl1-1 (C), proUOF1:SGL1/sgl1-1 (D), 35S:MtKNOX2 (E), and proUOF1:MtKNOX2 (F). Bars, 5 mm in (A–F).
Furthermore, it has been shown that L1 is necessary for the maintenance of indeterminacy in the underlying meristem layers (Kessler et al., 2006). To investigate the effects of L1 on compound leaf development, different genes driven by the UOF1 promoter were transformed into mutant or wild-type plants. The proUOF1:SGL1 cannot rescue the leaf defect of the sgl1-1 mutant (Figure 9D; Supplemental Figure 13, A and C), indicating that L1-specific expression of SGL1 did not sufficient for proper compound leaf development. KNOXI genes were essential for the regulation of indeterminacy of SAM, and overexpression of SHOOTMERISTEMLESS (STM)/BREVIPEDICELLUS (BP)-like MtKNOXI genes increased leaf complexity by producing ectopic leaflets along the petiolules in M. truncatula (Figure 9E; Supplemental Figure 13, B and C; Zhou et al., 2014). To explore whether the L1-specific expression of MtKNOXI was able to form ectopic leaflets, the proUOF1:MtKNOX2 construction was introduced into wild-type plants. The transgenic plants showed the normal compound leaf patterning (Figure 9F; Supplemental Figure 13, B and C), suggesting that increased MtKNOX2 activity in L1 did not alter the indeterminacy during leaf development. These data indicate that SGL1 and MtKNOXI in L1 play limited roles in the regulation of the indeterminacy during leaf formation.
Discussion
The roles of UOF1 and UOF2 genes in cuticle formation and organ development
In plants, the outer epidermal cells and cuticle present a barrier that maintains the external integrity of the plant (Samuels et al., 2008; Yeats and Rose, 2013). The plant cuticle is composed of lipophilic polymer cutin and cuticular waxes, which are synthesized from long-chain fatty acids, C16 or C18 fatty acids (Lee and Suh, 2013; Fich et al., 2016). The synthesized fatty acids are further elongated into VLCFAs. VLCFAs are major components of cuticular waxes, seed storage triacylglycerols, sphingolipids, suberin, and ω-hydroxylated fatty acids which involve in cutin monomer synthesis (Becraft, 1999; Pollard et al., 2008; Kunst and Samuels, 2009; Dominguez et al., 2011; Bernard and Joubes, 2013). In this study, the total amount of wax of both uof1 and uof2 mutants was significantly decreased, indicating that UOF1 and UOF2 play a similar role in the formation of the cuticle. It is notable that the amount of cutin was slightly increased in uof1-1 and uof2-1 mutants. The possible reason is that the blocked wax biosynthesis may lead to more C16 or C18 fatty acid precursors which subsequently participate in the cutin biosynthesis pathway.
The cuticle plays an important role in defining organ boundaries and allows organs to fully separate from each other during organogenesis (Fich et al., 2016). Thus, structural integrity of the cuticle is necessary to prevent organ fusion. In Arabidopsis, the LACERATA (LCR) enzyme CYP86A8, the BAHD family acyltransferase DCR, and the FAD-containing oxidoreductase HTH are required for cutin biosynthesis. Loss-of-function mutants of these genes display defective cuticle and exhibit strong fusion between rosette leaves and inflorescences (Wellesen et al., 2001; Krolikowski et al., 2003; Panikashvili et al., 2009). FDH, ONI1, and ECERIFERUM10 (CER10) encode FAE that involved in the synthesis of cuticular waxes, and their mutants show a strong organ fusion in leaves (Yephremov et al., 1999; Zheng et al., 2005; Ito et al., 2011). Furthermore, Arabidopsis CER8 encodes LACS1 that has overlapping functions with LACS2 in wax and cutin synthesis, and the lacs1 lacs2 double mutants display organ fusion between leaves (Lu et al., 2009; Weng et al., 2010). Expression of WAX INDUCER1 (WIN1) chimeric repressor (35S:WIN1-SRDX) also severely impaired the cuticular wax and cutin composition, which leads to adhesions between leaves and buds (Oshima et al., 2013). In M. truncatula, mutation of both UOF1 and UOF2 results in plants with organ adhesions between flowers and leaves. These findings indicate that the organ fusion is a typical characteristic of cuticle mutants among species.
Previous studies showed that the changes in composition and content of wax and cutin have effects on leaf size. In Arabidopsis, GROW FAST ON CYTOKININS1 (GFC1) and DESPERADO (DSO) encode enzyme and transporter that involved in cutin synthesis and secretion (Panikashvili et al., 2007; Wu et al., 2015). The gfc1 mutants produce larger leaves, while the dso mutant develop smaller leaves. In rice, the leaves of oni3 mutant and the curly flag leaf1 (cfl1) dominant mutant are covered with less wax, and show a shortened and curled leaf phenotype, respectively (Wu et al., 2011; Akiba et al., 2014). Moreover, the leaf margin formation was affected in these kinds of mutants. BDG encodes a cell wall localized α/β-hydrolase fold protein and participates in cutin synthesis (Kurdyukov et al., 2006). The bdg mutants produce elongated leaves with a smooth margin. In this study, the smooth leaf margin was also produced in uof1 mutant, instead of uof2 mutant, indicating that the specific expression of UOF1 in leaf margin is involved in the marginal serration formation. To our surprise, uof1 mutants showed increased leaflet number, which is different from the previous reports in other compound-leafed species. For example, stkcs6, the mutant of wax synthesis in potato (Solanum tuberosum L.), and cutin deficient2, the mutant of cutin synthesis in tomato (Solanum lycopersicum) did not show the defects in the compound leaf pattern (Serra et al., 2009; Nadakuduti et al., 2012; Kimbara et al., 2013). These findings suggest that UOF1 may play a species-specific function in leaf patterning in M. truncatula (Figure 10).
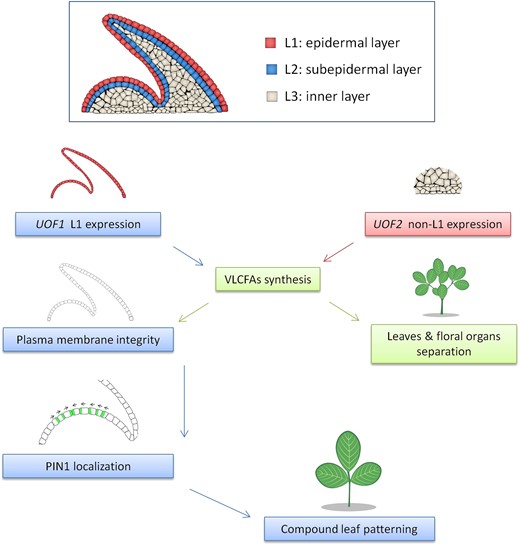
A proposed model of very long-chain fatty acids (VLCFAs) involved compound leaf patterning formation in M. truncatula. The shoot apical meristem (SAM) is divided into three distinct cell layers called L1/epidermal, L2/subepidermal, and L3/inner cell layer. Both UOF1 and UOF2 encode enzymes involved in the synthesis of VLCFAs, which is required for proper epidermal cuticular wax deposition, and loss of UOF1 and UOF2 function lead to cuticular wax-dependent organ adhesions between flowers and leaves. The UOF1 gene is specifically expressed in the L1 of the SAM and developing leaf primordia, whereas the UOF2 gene shows a non-L1-specific expression pattern. Furthermore, the L1-specific VLCFAs are also essential for the integrity of the plasma membrane, which is associated with the correct polar localization of PIN1 ortholog SLM1 during compound leaf patterning formation.
UOF1-dependent VLCFAs synthesis is critical for L1 integrity
The epidermis is formed by the L1 in the SAM and plays a critical role in the establishment and maintenance of the plant body (Yadav et al., 2014; Shapiro et al., 2015). The mutants with defects in L1 cell specification exhibited disorganized morphology (Ingram, 2007; Savaldi-Goldstein and Chory, 2008). FDH and ONI1 are specifically expressed in L1 and control epidermis identification in Arabidopsis and rice (Yephremov et al., 1999; Ito et al., 2011). Two receptor-like protein kinases, the Arabidopsis thaliana homolog of CRINKLY4 (ACR4) and ABNORMAL LEAF SHAPE2 (ALE2), are also specifically expressed in L1 and necessary for the proper differentiation of epidermal cells by regulating the expression of FDH in A. thaliana (Watanabe et al., 2004; Tanaka et al., 2007). Moreover, the integrity of L1 cells is affected by the biosynthesis of membrane lipids. In plants, phosphatidylcholine is a major lipid component in cell membranes, accounting for up to 60% of total membrane lipids (Moreau et al., 1998). The main step in phosphatidylcholine biosynthesis is the triple, sequential N-methylation of phosphoethanolamine, catalyzed by S-adenosyl-L-methionine:phosphoethanolamine N-methyltransferase (PEAMT) (Bolognese and McGraw, 2000; McNeil et al., 2000). Mutation in PEAMT changes the root epidermal cell integrity and root architecture (Cruz-Ramírez et al., 2004). In this study, UOF1 is specifically expressed in L1, which is confirmed by RNA in situ hybridization and promoter-GFP reporter plants. Additionally, the analysis of plasma membrane by observation of the 35S:AtPIP2A-GFP showed that loss of UOF1 function causes the defects in membrane integrity in L1. Therefore, these observations demonstrate a link between VLCFAs biosynthesis and the proper development of L1 in M. truncatula (Figure 10).
UOF1 regulates compound leaf patterning formation by modulating the polarity localization of AtPIN1/SLM1
The plant hormone auxin is an important regulator of plant growth and development (Benjamins and Scheres, 2008). Auxin is known to regulate the initiation of leaf primordia from the SAM, and the formation of leaf serrations (Benková et al., 2003; Barkoulas et al., 2008; Koenig et al., 2009). Directional auxin transport in the L1 of SAM is mainly mediated by the polar distribution of PIN1 (Leyser, 2011; Band et al., 2014; Adamowski and Friml, 2015). The polar localization of PIN1 can be modified by several factors, including the Ser/Thr protein kinase PINOID (PID), the fatty acids composition of the L1 plasma membrane, and protein-mediated subcellular trafficking and redirection (Christensen et al., 2000; Friml et al., 2004; Huang et al., 2010; Marhava, 2022). PID activates auxin efflux through phosphorylation of the four serines S1–S4 at PIN1, and acts as a binary switch in the apical-basal polar targeting of PIN proteins (Christensen et al., 2000; Luschnig and Vert, 2014). PINs cycle between their plasma membrane polar domains and endosomal compartments in a continuous and dynamic manner, and several components, such as cytoskeletal components and membrane lipid composition, are involved in this delivery (Kleine-Vehn et al., 2008; Naramoto, 2017; Sauer and Kleine-Vehn, 2019). Mutants with defects in fatty acids and their derivatives, such as sterols, sphingolipids, and phosphatidylcholine, showed disturbed cell polarity, PIN protein positioning, and auxin distribution (Prud'homme and Moore, 1992). A mutation in the CEPHALOPOD (CPH), also referred to as SMT1, results in a significant decrease in the level of sitosterol and an increase in the level of cholesterol, leading to conspicuous cell polarity defects and redistribution of PIN1 protein in membranes of elongated vascular cells (Willemsen, 2003). In M. truncatula, both leaf margin formation and compound leaf development are cooperatively regulated by the auxin/SLM1 module (Zhou et al., 2011, 2013). Accordingly, uof1 mutant also displayed the similar defects, which are relatively smooth leaf margin and altered compound leaf patterning. We demonstrate that UOF1 is involved in the leaf patterning formation by regulating L1 plasma membrane integrity and subsequent polar distribution of PIN1. Taken together, these observations provide evidence that UOF1-mediated biosynthesis of VLCFAs in L1 play important roles in compound leaf development, which is associated with the polarization of auxin efflux carrier in M. truncatula (Figure 10).
L1-specific expression of MtKNOXI and SGL1 play limited roles in maintaining indeterminacy of the leaf primordia
Activating indeterminacy in leaf primordia will increase leaf complexity. KNOXI genes play important roles in leaf development in many species (Hay and Tsiantis, 2010). In tomato and cardamine (Cardamine hirsuta), up-regulation of KNOXI genes delays leaf differentiation and prolongs the morphogenetic window, causing increased leaf complexity, indicating that KNOXI genes are necessary and sufficient for leaflet formation (Hay and Tsiantis, 2006; Shani et al., 2009). In M. truncatula, ectopic expression of MtKNOXI genes is sufficient for increasing the leaflet number (Zhou et al., 2014). Pea (Pisum sativum) UNIFOLIATA (UNI) and Medicago SGL1 are two orthologs of Arabidopsis LFY gene, promoting the transient indeterminate growth of the leaf (Gourlay et al., 2000). UNI is expressed in the leaf blastozone, and the uni mutant displays reduced leaf complexity. In M. truncatula, SGL1 activities are necessary for compound leaf formation (Wang et al., 2008). However, L1-specific expression of MtKNOX2 and SGL1 activity cannot produce ectopic leaflet and complement the defects in sgl1 mutant, respectively, indicating that SGL1 and MtKNOXI are not responsible for the compound leaf patterning in L1.
Materials and methods
Plant materials and growth conditions
The wild-type used in this study was barrelclover (Medicago truncatula) R108 ecotype. Mutant lines uof1-1, uof1-2, uof1-3, uof1-4, uof2-1, uof2-2, slm1-1 (Zhou et al., 2011), and sgl1-1 (Wang et al., 2008) were identified from the Tnt1 retrotransposon-tagged mutant collection of M. truncatula R108 ecotype (Tadege et al., 2008). Scarified seeds were germinated in moist filter paper and placed at 4°C for 5 days in the dark. Plants were grown at 22°C:20°C, day:night temperatures with a 16 h:8 h, day:night photoperiod and 75% relative humidity, and 150 μmol m−2 s−1 light intensity.
Genetic complementation and promoter-GUS analysis
For genetic complementation, a 2512 and 2231 bp promoter sequence plus the genomic sequence of UOF1 and UOF2 were amplified using primer pairs UOF1-com-F/UOF1-com-R and UOF2-com-F/UOF2-com-R, and cloned into the gateway destination vector pEarleyGate 301. For expression pattern analysis, the 2512 and 2231 bp promoter sequences of UOF1 and UOF2 were amplified using primer pairs proUOF1-F/proUOF1-R and proUOF2-F/proUOF2-R, and transferred into the gateway destination vector pBGWFS7. The primers used are listed in Supplemental Table 1. All final destination vectors were introduced into the disarmed Agrobacterium tumefaciens EHA105 strain. For genetic complementation, leaves of uof1-1 and uof2-1 were transformed with EHA105 harboring proUOF1:UOF1 and proUOF2:UOF2 vectors, respectively. For expression pattern analysis, leaves of wild-type were transformed with proUOF1:GUS-GFP and proUOF2:GUS-GFP vectors.
RNA extraction, reverse transcription PCR (RT-PCR), and reverse transcription quantitative PCR (RT-qPCR)
To measure the transcript levels of UOF1, UOF2, and SLM1 in wild-type and mutant plants, fully expanded leaves were harvested from wild-type and different mutant lines. To measure the tissue/organ expression patterns of UOF1 and UOF2, the RNA samples were isolated from different wild-type tissues. RNA was extracted using the TRIzol reagent (Invitrogen, USA), according to the manufacturer's instructions. cDNA was synthesized with 2 μg of total RNA using the PrimeScript II cDNA Synthesis Kit (Takara, Japan). The cDNA was used as templates for RT-PCR and RT-qPCR. RT-qPCR was performed using the SYB RT-qPCR mix (TaKaRa, Japan), and three biological samples were repeated. The 2−ΔΔCT method was used to calculate relative expression levels, respectively. The MtActin gene was used as an internal control (Livak and Schmittgen, 2001). The primers used for RT-PCR and RT-qPCR analysis are listed in Supplemental Table 1.
RNA in situ hybridization
For RNA in situ hybridization, the probe fragments of 450 bp UOF1 CDS, 380 bp UOF2 CDS, and 553 bp SLM1 CDS were polymerase chain reaction amplified using primers listed in Supplemental Table 1. These PCR products were cloned into pGEM-T vector (Promega, USA) and then labeled with digoxigenin-11-UTP (Roche, Switzerland). RNA in situ hybridization was performed on vegetative and reproductive shoot buds of wild-type or uof1-1 plants as described previously (Zhou et al., 2011). The results were visualized using a fluorescence microscope (Olympus, Japan).
Sequences alignment and phylogenetic analysis
For sequences alignment, the full-length protein sequences of the KCS and CYP86A family members from M. truncatula and Arabidopsis (Arabidopsis thaliana) were aligned using online CLUSTALW (http://www.genome.jp/tools/clustalw/). The phylogenetic trees of the KCS and CYP86A family members were constructed using MEGA6 software suite (http://www.megasoftware.net/) by the Neighbor-Joining (NJ) method with 1000 bootstrap replicates in the p-distance model.
Scanning electron microscopy (SEM) analysis and toluidine blue (TB) staining
For SEM analysis, the leaves, flowers, pods, and reproductive shoot buds were fixed in 3% (v/v) glutaraldehyde, washed five times in 1 × PBS every 30 min, dehydrated in a series of ethanol (30%, 50%, 60%, 70%, 85%, 95%, 100% [v/v]) every 30 min, and then carbon dioxide dried and sprayed with gold powder. The samples were viewed under a Tecnai electron microscope (FEI, USA) using an accelerating voltage of 10 kV. The TB staining protocol was followed as described previously (Tanaka et al., 2004). Briefly, an aqueous solution of 0.05% (w/v) TB (Sigma, USA) was poured onto the plates on which samples were submerged. After 10 min, the TB solution was removed, and the leaves and flowers were washed with water.
Pollen staining and β-glucuronidase (GUS) staining
To determine pollen viability, flowers of the wild-type, uof1-1, and uof2-1 were collected and fixed by Carnoy's fixative for 2 h at room temperature, and then stained with Alexander's solution for 2 h at room temperature as described (Alexander, 1969). For GUS staining, flowers and fully expanded leaves were collected. The GUS activity was histochemically detected as previously described (Jefferson et al., 1987).
GFP fluorescence imaging
The marker lines, proAtPIN1:AtPIN1-GFP/WT (Zhou et al., 2011) and proAtPIP2A-GFP/WT (Luo and Nakata, 2012), were obtained as described. For fluorescent analysis of proAtPIN1:AtPIN1-GFP and proAtPIP2A-GFP in wilt-type and uof1-1 plants. The marker plants were crossed with uof1-1 (+/−), respectively, and then selfed to generate the proAtPIN1:AtPIN1-GFP/uof1-1 (−/−) and proAtPIP2A-GFP/uof1-1 (−/−) plants. Apical shoot buds and leaves were collected and observed with a Zeiss 780 confocal laser scanning microscope (Zeiss, Germany). The GFP signals were excited at 488 nm line of an argon laser, and emission was collected at 510 nm. All experiments are representative of at least 10 observed samples from three independent experiments.
Cuticular waxes and cutin analysis
The cuticular waxes and cutin composition of 4-week-old leaves of wild-type, uof1-1, and uof2-1 were determined as described (Lu et al., 2011). For identification of monounsaturated primary alcohols, thin layer chromatography (TLC) analysis was used to purify the primary alcohols. The total wax mixtures from leaves of wild-type, uof1-1, and uof2-1 were separated on silica gel plates using hexane-diethylether-acetic acid (50:50:1 [v/v/v]) (Sigma-Aldrich, USA). The alcohol fraction was scraped off and extracted in chloroform, dried under nitrogen, and derivatized with N,O-bis(trimethylsilyl)trifluoroacetamide (BSTFA) (Sigma-Aldrich, USA), then analyzed on gas chromatography-flame ionization detector (GC-FID) and gas chromatography-mass spectrometer (GC-MS). To determine the location of the double bond, the monounsaturated fatty alcohols were first converted to acetic ester by acetic anhydride and then derivatized by dimethyl disulfide (DMDS) (Sigma-Aldrich, USA), and finally analyzed by GC-MS using the method as described (Leonhardt and Devilbiss, 1985).
Transcriptomic assay
For transcriptomic analysis, shoot buds were harvested from 4-week-old wild-type and uof1-1 mutant plants. Three biological replicates of each sample were prepared. The total RNA of each sample was extracted, and all samples were sequenced on a BGISEQ-500 platform at the BGI Genomics Institute (BGI-Shenzhen, China). For each replicate, RNA sequencing generated more than 20 million raw reads. Raw reads were first purified by Trimmomatic (v0.37, BGI-Shenzhen, China) (Chen et al., 2018). Adapter sequences, low-quality reads, and reads containing more than 5% unknown nucleotides were filtered out from raw reads. Then, clean reads were aligned against the annotated M. truncatula reference transcriptome using Bowtie (v4.0, Baltimore, USA). RSEM (RNA-Seq by Expectation Maximization) was used for gene expression analysis and R package DEGseq (Differentially Expressed Gene Identification for RNA-seq data) was used for identifying DEGs. All DEGs characterized were up-/down-regulated more than two-fold and had a false discovery rate (FDR) < 0.001. The hypergeometric test of P-value adjusted by the FDR method was used to evaluate the enrichment of GO terms and the KEGG pathway. The heatmap was created by Helm software (Heatmap Illustrator, version 1.0). The DEGs are listed in Supplemental Table 2.
Measurements of free IAA contents
For quantification of free IAA content, the leaves from 4-week-old wild-type and uof1-1 mutants were harvested and weighed (each sample 200 mg), and then ground into powder in liquid nitrogen. Add 1 ml ethyl acetate, containing 200 ng 15N-IAA served as an internal standard, to the samples and then vortexed for 10 min. After centrifugation at 15,000g for 10 min at 4°C, the supernatants were collected and the pellets were re-extracted with 0.5 ml ethyl acetate. The combined supernatants were evaporated to dryness in a vacuum concentrator. The acquired residues were resuspended in 0.5 ml of 70% (v/v) methanol, and then supernatants were pipetted to glass vials for high performance liquid chromatography-tandem mass spectrometry (HPLC-MS/MS) analysis (Prominence LC-20A and LCMS-8040; Shimadzu, Kyoto, Japan).
Measurement of water loss
For water loss rate analysis, 4-week-old leaves from wild-type, uof1-1, and uof2-1 plants were collected and maintained at room temperature. Weight was measured at different time points. Then the leaves were put in a 60°C oven overnight. Total water was defined as the fresh weight less the dry weight after the heat treatment. The water loss at each time point was expressed as the percentage of the water loss over total water.
Statistical analysis
Error bars in RT-qPCR, free IAA content, water loss, and wax and cutin measurement figures show the standard deviation of three biological replicates, as indicated in the legends. Most of the pairwise comparisons between the means were performed using a two-sided Student's t test, using GraphPad Prism version 9 software.
Data availability
The data used to support the findings of this study appeared in the article and are available from the corresponding author.
Accession numbers
Sequence data from this article can be found in the Medicago truncatula R108_HiC website (https://medicago.toulouse.inrae.fr/MtrunR108_HiC/): UOF1, Medtr3g105550; UOF2, Medtr8g030590; SLM1, Medtr7g089360; SGL1, Medtr3g098560; MtKNOX2, Medtr1g017080.
Supplemental data
The following materials are available in the online version of this article.
Supplemental Figure S1. SEM analysis and pollen staining of flower organs of wild-type, uof1-1, and uof2-1 mutants.
Supplemental Figure S2. Genetic segregation ratio and co-segregation analysis of uof1 and uof2 mutants.
Supplemental Figure S3. Phylogenetic analysis of the KCS family proteins in A. thaliana (At) and M. truncatula (Mt).
Supplemental Figure S4. Alignment of amino acid sequences of UOF1 and its homologs from different species.
Supplemental Figure S5. Phylogenetic analysis of the cytochrome P450 CYP86 subfamily proteins in A. thaliana (At) and M. truncatula (Mt).
Supplemental Figure S6. Alignment of amino acid sequences of UOF2 and its homologs from different species.
Supplemental Figure S7. Organ adhesion phenotypes of uof1 and uof2 mutants.
Supplemental Figure S8. Leaves and flowers phenotype of the single and double mutants and genetic complementation analysis.
Supplemental Figure S9. The expression pattern of UOF1.
Supplemental Figure S10. Comparison of functional categories of differentially expressed genes in uof1-1 and wild-type shoot buds.
Supplemental Figure S11. SEM images of epidermal cells of wild-type and uof1-1 leaves.
Supplemental Figure S12. Leaf and cuticle wax phenotype of the uof1-1 slm1-1 double mutants.
Supplemental Figure S13. The function of UOF1 in compound leaf patterning.
Supplemental Table S1. Primers used in this study.
Supplemental Table S2. The differentially expressed genes (DEGs) in WT and uof1-1 mutant shoot buds.
Acknowledgments
We thank Dr Sen Wang from the State Key Laboratory of Microbial Technology, Shandong University for the assistance in SEM analysis.
Funding
This work was supported by grants from the National Natural Science Foundation of China (31871459, 31900172, and 32201446), the Natural Science Foundation of Shandong Province (ZR2020KC018 and ZR2021QC032), and the China Postdoctoral Science Foundation (2021M691948). Development of M. truncatula Tnt1 mutant population was, in part, funded by the National Science Foundation, USA (DBI-0703285 and IOS-1127155).
References
Author notes
H.W. and C.Z. designed the research; H.W., Z.L., Y.X., and L.H. performed the experiments; J.Z. performed the RNA in situ hybridization experiments; M.C., Z.W., J.W., and K.S.M. contributed to the Tnt1-tagged mutants; X.Y., S.L., J.T., and L.X. analyzed the waxes and cutin composition and auxin level; H.W. and C.Z. wrote the paper. All the authors read and approved the contents of this manuscript.
The author responsible for distribution of materials integral to the findings presented in this article in accordance with the policy described in the Instructions for Authors (https://dbpia.nl.go.kr/plphys/pages/General-Instructions) is Chuanen Zhou ([email protected]).
Conflict of interest statement: The authors have no conflicts of interest to declare.