-
PDF
- Split View
-
Views
-
Cite
Cite
Zhixue Wang, Leiyun Yang, Jian Hua, The intracellular immune receptor like gene SNC1 is an enhancer of effector-triggered immunity in Arabidopsis, Plant Physiology, Volume 191, Issue 2, February 2023, Pages 874–884, https://doi.org/10.1093/plphys/kiac543
- Share Icon Share
Abstract
Plants contain many nucleotide-binding leucine-rich repeat (NLR) proteins that are postulated to function as intracellular immune receptors but do not yet have an identified function during plant–pathogen interactions. SUPPRESSOR OF NPR1-1, CONSTITUTIVE 1 (SNC1) is one such NLR protein of the Toll-interleukin 1 receptor (TIR) type, despite its well-characterized gain-of-function activity and its involvement in autoimmunity in Arabidopsis (Arabidopsis thaliana). Here, we investigated the role of SNC1 in natural plant–pathogen interactions and genetically tested the importance of the enzymatic activities of its TIR domain for its function. The SNC1 loss-of-function mutants were more susceptible to avirulent bacterial pathogen strains of Pseudomonas syringae containing specific effectors, especially under constant light growth condition. The mutants also had reduced defense gene expression induction and hypersensitive responses upon infection by avirulent pathogens under constant light growth condition. In addition, genetic and biochemical studies supported that the TIR enzymatic activity of SNC1 is required for its gain-of-function activity. In sum, our study uncovers the role of SNC1 as an amplifier of plant defense responses during natural plant–pathogen interactions and indicates its use of enzymatic activity and intermolecular interactions for triggering autoimmune responses.
Introduction
Plants use the cell-surface localized pattern recognition receptors (PRRs) and the intracellular nucleotide-binding leucine-rich repeat (NLR) proteins to detect pathogens (Jones and Dangl, 2006). PRRs recognize conserved features of pathogens called pathogen-associated molecular patterns (PAMPs) to activate pattern-triggered immunity (PTI), while NLRs directly or indirectly recognize effectors and toxins secreted from pathogens to activate effector-triggered immunity (ETI). Based on their distinct N-terminal domains, most plant NLRs are categorized into three groups: toll-interleukin 1 receptor (TIR)-NLR (TNL), coiled-coil-NLR (CNL), and RPW8-type CC-NLR (RNL) (Monteiro and Nishimura, 2018). NLRs can also be classified as sensor NLRs that detect pathogen effectors and helper NLRs that initiate immune signaling (Jubic et al., 2019). Among 207 NLR or NLR-like proteins in Arabidopsis (Arabidopsis thaliana) ecotype Col-0 (hereafter, Col-0) (Meyers et al., 2003), 24 NLRs have been experimentally validated as helper NLRs (10 total) and sensor NLRs (14) with known cognate effectors (Kourelis et al., 2021). The molecular mechanism of NLR activation has been revealed by identifying the structure of several NLR protein complexes. Upon activation, NLRs undergo oligomerization to activate downstream signaling events (Wang et al., 2019; Martin et al., 2020; Ma et al., 2020). The formation of oligomers could create calcium permeable cation channels for defense activation which were shown for the CNL protein HOPZ-ACTIVATED RESISTANCE 1 (ZAR1) and two RNL proteins N REQUIREMENT GENE 1 (NRG1) and ACTIVATED DISEASE RESISTANCE 1-LIKE 1 (ADR1-L1) (Bi et al., 2021; Jacob et al., 2021). Oligomerization has also been shown to activate distinct enzymatic activities of the TIR domain in TNLs. Self-associated TIRs exhibit NADase activity that degrades oxidized nicotinamide adenine dinucleotide (NAD+) into nicotinamide and variants of cyclic adenosine diphosphate ribose (v-cADPR) (Horsefield et al., 2019; Wan et al., 2019). The product v-cADPR is essential but not sufficient for cell death and defense activation (Duxbury et al., 2020). TIR also has an ADPR polymerase-like activity which together with the NADase activity enables the activation of the downstream immune signaling (Jia et al., 2022; Huang et al., 2022). In addition, some TIR domain proteins exhibit nuclease activity and 2′,3′-cAMP/cGMP synthetase activity which are also required for TIR-mediated cell death in plants (Yu et al., 2022a, 2022b).
SUPPRESSOR OF NPR1-1, CONSTITUTIVE 1 (SNC1) is an extensively studied NLR in Arabidopsis. Its loss of function (LOF) mutation suppresses or reduces enhanced disease resistance and growth defects in autoimmune mutants defective in genes encoding a wide range of proteins. They include, but not limited to, a plasma membrane-localized protein BONZAI 1 (BON1; Yang and Hua, 2004) and its interactor BON ASSOCIATION PROTEIN 1 (BAP1; Yang et al., 2006a, 2006b), transcriptional repressor like protein SUPPRESSOR OF rps4-RLD1 (SRFR1; Kim et al., 2010) and histone deacetylase interacting protein HIGH EXPRESSION OF OSMOTICALLY RESPONSIVE GENES 15 (HOS15, Yang et al., 2020), as well as primary and secondary metabolites biosynthesis enzymes such as ENOLASE 2 (ENO2; Yang et al., 2022), NUCLEOSIDE DIPHOSPHATE LINKED TO SOME MOIETY X 6 (NUDT6) and NUDT7 (Wang et al., 2013), and UDP-GLUCOSYL TRANSFERASE 73C7 (UGT73C7; Huang et al., 2021). Likewise, autoimmunity induced by a natural ACD6 allele was also suppressed by a SNC1 natural variant (Zhu et al., 2018). Overexpression of SNC1 or activation of SNC1 by glutamate at position 561 to lysine mutation (SNC1-1) led to autoimmunity characterized by inhibition of plant growth and constitutive defense gene expression (Li et al., 2001; Yang and Hua 2004). By exploiting the autoimmune mutants bon1 and snc1-1, the regulation of SNC1 at the transcriptional and post-transcriptional levels has been extensively studied (Gou and Hua, 2012; Johnson et al., 2012; Zou et al., 2014; Li et al., 2015; Zou et al., 2017; Yang et al., 2021).
The function of the wild-type SNC1 in natural plant–pathogen interactions remains largely unknown despite its prominent role in autoimmunity and extensive study of its gain-of-function mutants. The snc1 single LOF mutants have not been reported to have defects in disease resistance, although the rps4 snc1 double mutant exhibited a reduced resistance to Pst DC3000 carrying AvrRps4 compared with rps4 (Kim et al., 2010). Here, we investigated the role of SNC1 by characterizing its Arabidopsis LOF mutants snc1-11 and snc1-12 in disease resistance and defense responses after infection with the nonvirulent, virulent, and avirulent strains of the bacterial pathogen Pseudomonas syringae pv tomato (Pst) DC3000. We found that the snc1-11 and snc1-12 mutants display similar disease resistance to nonvirulent and virulent stains and are more susceptible to some but not all avirulent strains compared with the wild-type plants, especially under constant light conditions. In addition, the mutants exhibit delayed and attenuated defense gene induction after Pst DC3000 AvrRpt2 and Pst DC3000 AvrRps4 infection, accompanied by reduced hypersensitive response (HR). Furthermore, we provide genetic evidence that SNC1 functions as oligomers and that the NADase catalytic residue glutamate is required for its gain-of-function activity in triggering autoimmunity. Our study thus uncovers a general role of SNC1 in natural plant–pathogen interaction and suggests an intermolecular interaction in enzymatic activation of the SNC1 protein for defense response activation.
Results
SNC1 contributes to resistance against Pst DC3000 with AvrRpt2, AvrRps4, or HopAE1
To reveal the function of the wild-type SNC1 gene in plant disease resistance, we characterized two Arabidopsis LOF mutants snc1-11 and snc1-12 in response to different strains of Pst DC3000 including the nonvirulent strain Pst DC3000 hrcC-, virulent strain Pst DC3000, and avirulent strains carrying one of the following effectors: AvrRpt2, AvrRps4, AvrPphB, AvrB, AvrA1, HopAE1, HopAH1, HopAG1, HopAS1, HopW1, or HopS1 (Mazo-Molina et al., 2019; Ahn et al., 2022). The first four Avr genes have known corresponding NLR genes of both the CNL and TNL type (Supplemental Table 1). Under the 12 h light/12 h dark (12 h light) growth condition (Figure 1, A–M and Supplemental Figure 1), neither snc1-11 nor snc1-12 showed significant differences for the growth of Pst DC3000 hrcC- or Pst DC3000 compared with the wild-type Col-0 (hereafter, WT) (Figure 1, A and B and Supplemental Figure 1), which is consistent with previous analyses (Yang and Hua, 2004). All avirulent strains tested had the same growth in the mutants and the WT except for the Pst DC3000 strain with AvrRpt2 which had more growth in the snc1-11 and snc1-12 mutants than that in the WT (Figure 1, C–L and Supplemental Figure 1).
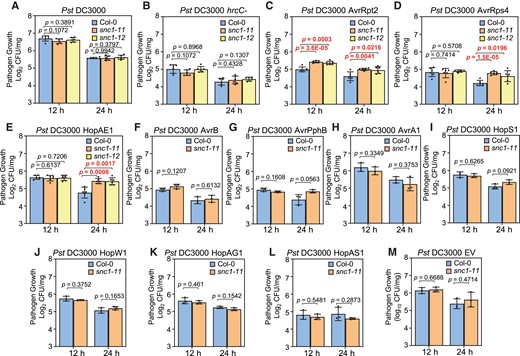
The loss of function mutants of SNC1 are more susceptible to avirulent strains of Pst DC3000. Growth of Pst DC3000 (A), Pst DC3000 hrcC- (B), Pst DC3000 carrying effectors (C-L) or empty vector (M) in Col-0, snc1-11 and snc1-12 plants at three days post-infection. Plants were grown under 12-h light or 24-h light conditions. Error bars represent Sd of six biological replicates for (A–E) or three biological replicates for (F–M). Significant differences were tested by Student’s t-test. P values < 0.05 were highlighted.
Because autoimmunity mediated by SNC1 is enhanced under constant light (Yan et al., 2019), we also examined disease resistance phenotypes of the snc1 LOF mutants under the constant 24-h light condition. All the strains of Pst DC3000 had reduced growth in WT plants under the constant light condition compared with that under the 12-h light condition (Figure 1), indicating an enhanced resistance under the constant light condition. Growth of Pst DC3000 AvrRpt2 was higher in the snc1-11 and snc1-12 mutants compared with the WT under the 24 h light condition, similar to that under the 12-h light condition (Figure 1C). Interestingly, growth of Pst DC3000 AvrRps4 and Pst DC3000 HopAE1 was also higher in the snc1-11 and snc1-12 mutants compared with the WT under the constant light condition, which was not observed under the 12-h light condition (Figure 1, D and E). Growth of Pst DC3000 strains with other effectors or the empty vector was the same in the mutants as in the WT under 24 h light, as under 12 h light (Figure 1, F–M).
Taken together, these results indicate that SNC1 contributes to disease resistance triggered by AvrRpt2, HopAE1, and AvrRps4, and this function is light dependent. It is worth noting that AvrRpt2 and AvrRps4 are recognized by CNL RPS2 and TNL pair RPS4/RRS1, respectively.
SNC1 contributes to the NLR-mediated hypersensitive response triggered by AvrRpt2 and AvrRps4
Initiation of NLR-mediated effector-triggered immune responses is often accompanied by localized programmed cell death known as the hypersensitive response (HR). We monitored the HR over time in response to Pst DC3000 AvrRpt2 and Pst DC3000 AvrRps4 in the snc1-11 and snc1-12 mutants under the 24-h light condition. Relative ion leakage analysis revealed that the onset of HR was not altered in the two snc1 LOF mutants compared with the WT (Figure 2). However, the intensity of AvrRpt2-triggered HR was significantly reduced in the mutants compared with the WT starting from 17 h post-inoculation, and the difference was sustained throughout the duration of the treatment (Figure 2A). Similarly, a reduced HR was observed for Pst DC3000 AvrRps4, and the difference was significant from 10 h post-inoculation on (Figure 2B). These results indicate that SNC1 contributes to the HR triggered by two NLRs, one CNL (RPS2) and one TNL (RRS1/RPS4).
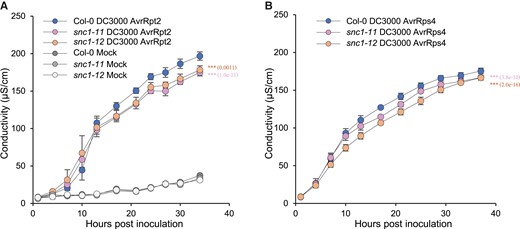
NLR-mediated hypersensitive responses are reduced in the loss of function mutants of SNC1. A-B, Quantification of cell death of Col-0, snc1-11 and snc1-12 leaves inoculated with Pst DC3000 AvrRpt2 (A) or Pst DC3000 AvrRps4 (B). Cell death was assessed by conductivity at the indicated time points. Data are presented as mean ± Sd of four biological replicates. Significant differences were tested by two-way ANOVA (***, P < 0.001) and P values assessing genotype effects were provided in brackets.
SNC1 is required for the full induction of defense gene expression by Pst DC3000 AvrRpt2
We next examined the expression of defense-related genes in the snc1 LOF mutants. PATHOGENESIS-RELATED GENE 1 (PR1), a SA-responsive defense marker gene, was examined at 0, 1, 4, 9, and 24 h post-infection with Pst DC3000 AvrRpt2, Pst DC3000, and Pst DC3000 hrcC- under the constant light condition. Interestingly, prior to pathogen inoculation (0 h), both snc1-11 and snc1-12 had reduced basal PR1 expression compared with the WT, but this was not observed under the 12-h light condition (Figure 3A). In response to Pst DC3000 AvrRpt2, the expression of PR1 was rapidly induced in the WT as early as 4 h post-infection, consistent with previous reports (Figure 3B, Mine et al., 2018). The induction of PR1 by Pst DC3000 AvrRpt2 was observed at 9 h but not 4 h post-infection in the snc1-11 and snc1-12 mutants (Figure 3B). In addition, the snc1-11 and snc1-12 mutants had an overall lower PR1 expression compared with the WT throughout the treatment (Figure 3B). The reduction of PR1 expression was associated with the reduced resistance against Pst DC3000 AvrRpt2 with the loss of SNC1 function (Figure 1C). Strains of Pst DC3000 and Pst DC3000 hrcC- induced PR1 expression at a later time point and a lower level than Pst DC3000 AvrRpt2 in the WT (Figure 3, C and D). The induction of PR1 by Pst DC3000 was slightly reduced in the snc1-11 and snc1-12 mutants compared with the WT at 4 h, but not at 9 and 24 h (Figure 3C). The induction of PR1 by Pst DC3000 hrcC- was not different between the mutants and the WT at 4 and 9 h but was lower at 24 h compared with the WT (Figure 3D). In addition, we assayed the expression of the SA biosynthesis gene SALICYLIC ACID INDUCTION DEFICIENT 2 (SID2) in the snc1-11 and snc1-12 mutants. The basal expression of SID2 was not altered in the mutants compared with the WT (Figure 3E). The induction of SID2 by Pst DC3000 AvrRpt2 infection at 1 and 4 h post-infection was reduced in the snc1-11 and snc1-12 mutants (Figure 3E). These data indicate that SNC1 contributes to defense gene induction and SA biosynthesis upregulation triggered by Pst DC3000 AvrRpt2 but not significantly by virulent and non-virulent strains of Pst DC3000.
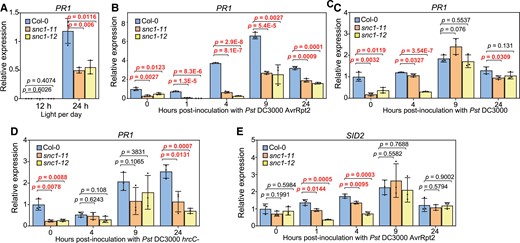
Induction of defense genes by pathogen strains of Pst DC3000 is reduced in the SNC1 loss of function mutants. A, Analysis of gene expression of PR1 in two-week-old Col-0, snc1-11, and snc1-12 plants grown under 12-h light (12 h) or 24-h light (24 h) conditions. B–D, Analysis of gene expression of PR1 in Col-0, snc1-11 and snc1-12 plants inoculated with Pst DC3000 AvrRpt2 (B), Pst DC3000 (C) or Pst DC3000 hrcC- (D) at the indicated time points. E, Analysis of gene expression of SID2 in Col-0, snc1-11 and snc1-12 plants inoculated with Pst DC3000 AvrRpt2 at the indicated time points. Two-week-old plants grown under constant light were used. Error bars represent Sd of three biological replicates. Significant differences were tested by Student’s t-test and the P values <0.05 were highlighted.
SNC1 does not have a significant function in flg22-triggered immune responses
The expression of SNC1 is induced by all seven PTI-inducing molecular patterns tested, flg22, elf18, Pep1, nlp20, OGs, CO8, and 3-OH-FA, according to an RNA sequencing (RNA-seq) dataset (Figure 4A; Bjornson et al., 2021). All these seven patterns induced the SNC1 transcript within 30 min post-treatment (Figure 4A). Four molecular patterns, flg22, elf18, Pep1, and nlp20, induced a more drastic and prolonged SNC1 expression compared with the other three (Figure 4A). Therefore, we tested whether or not SNC1 has an effect on PTI by analyzing both the expression of PTI marker genes and the early ROS production in the snc1-11 and snc1-12 mutants after flg22 treatment. Two PTI-responsive genes FLG22-INDUCED RECEPTOR-LIKE KINASE 1 (FRK1) and WRKY29 were quickly induced by flg22 at 1 h after treatment in the WT, which is consistent with a previous report (Figure 4, B and C; Bjornson et al., 2021). The snc1 LOF mutants had a WT expression level of FRK1 and WRKY29, although their expression in one mutant allele exhibited a significant difference from the WT at some time points (Figure 4, B and C). ROS production was also compared between the WT and the snc1 LOF mutants. Its induction occurred at 14 min post-flg22 treatment in the WT, and the snc1-11 and snc1-12 mutants had a comparable ROS accumulation as to the WT (Figure 4D). These data indicate that SNC1 does not have a significant role in flg22 triggered-early defense responses.
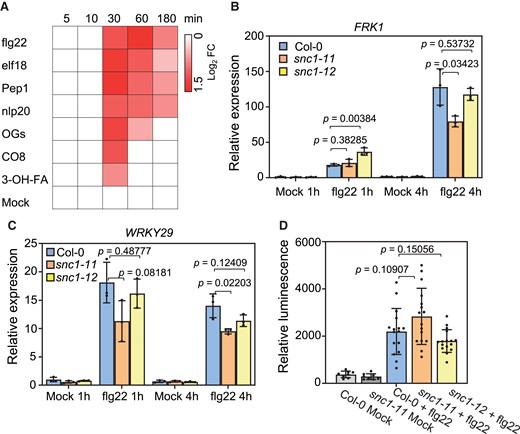
PTI responses to flg22 were not significantly altered by the loss of SNC1 function. A, Induction of SNC1 gene expression by different immunity triggering patterns at the indicated time points. Data were extracted from Bjornson et al., 2021 B and C, Analysis of gene expression of FRK1 (B) and WRKY29 (C) in Col-0, snc1-11 and snc1-12 plants treated with 1 μM flg22 at the indicated time point. D, Analysis of flg22 triggered ROS production in Col-0, snc1-11 and snc1-12 plants. Relative luminescence was quantified at 14 min post-treatment. Error bars represent Sd of three biological replicates for (B-C) and eight or sixteen biological replicates for (D). The P values of Student’s t-test for significant differences are indicated.
The E93 catalytic residue of SNC1 is important for autoimmunity from SNC1-1
NADase activity of the TIR domain of SNC1 has been shown to be required for cell death induction when heterogeneously expressed in Nicotiana benthamiana (N. ben) plants. We asked if the NADase activity of SNC1 is required for its function in Arabidopsis. We resorted to the active form of the SNC1 protein, SNC1-1, for structure–function studies because it causes a more trackable phenotypic effect than the wild-type SNC1 especially considering the large phenotypic variations of transgenic plants carrying the SNC1 transgene (Zhu et al., 2010a, 2010b). The glutamate 93 (E93) residue, designated as E84 in Horsefield et al. (2019), of the protein from the primary transcript (annotation in TAIR 11) is the putative NADase catalytic site and is also important for the putative 2′,3′-cAMP/cGMP synthetase activity. This E93 residue was mutated to alanine (A) in the gain-of-function form SNC1-1 (Figure 5A). SNC1-1 and the E93A form of SNC1-1 (SNC1-1E93A) were expressed as GFP fusions under the SNC1 native promoter, and the resulting pSNC1:SNC1-1:GFP (SNC1-1-GFP) and pSNC1:SNC1-1-E93A:GFP (SNC1-1E93A-GFP) were transformed into Col-0. The T1 plants of SNC1-1-GFP/Col and SNC1-1E93A-GFP/Col exhibited reduced rosette growth to varying degrees. Based on the rosette size, they were categorized into four groups, A (largest) and B–D (smallest) (Figure 5B). The effect of a transgene was measured by the growth inhibition score defined by “0×A% + 1×B% + 2×C% + 3×D%” from all T1 plants with this transgene (Figure 5C). The SNC1-1E93A-GFP had a growth inhibition score of 131% and SNC1-1-GFP had a score of 153% in Col-0 (Figure 5C). This indicates that both of the SNC1-1 forms inhibit plant growth but SNC1-1E93A has a lower activity than SNC1-1.
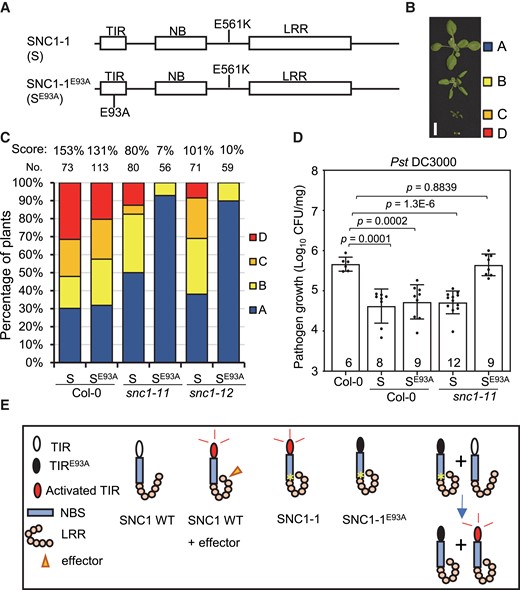
The NADase catalytic residue of SNC1 is required for autoimmunity from SNC1-1. A, Schematic diagram of TIR, NBS and LRR domains of SNC1 and the position of the E561K (SNC1-1 or S) and E93A (SNC1-1E93A, or SE93A) mutations in SNC1 protein. B, Representative phenotypes of three-week-old T1 plants containing the pSNC1:SNC1-1:GFP (S) or pSNC1:SNC1-1-E93A:GFP (SE93A) transgene. They are grouped into A, B, C and D. Scale bar, 1 cm. C, Distribution of four groups of phenotypes (as defined in B) in T1 plants with S or SE93A transgenes in Col-0, snc1-11 and snc1-12. The number (No.) of total T1 plants for each genotype is shown above the bar. The growth inhibition score (score) as defined by (0×A% + 1×B% + 2×C% + 3×D%) for each genotype is shown on the top. D, Growth of Pst DC3000 in Col-0, the S and SE93A transgenic plants in Col-0 and snc1-11 backgrounds at three days post-infection. Data are presented as mean ± Sd. The number of Col-0 plants or independent lines of transgenic plants that were used in the assay are indicated inside the bar. Significant differences were tested by Student’s t-test, and the P values were provided. E, Schematic diagram of immune signaling through the SNC1 TIR enzymatic function. Under nonpathogenic condition, SNC1 WT is in a resting state and there is no immune signaling. Pathogen effectors or the SNC1-1 mutation activate SNC1 TIR enzymatic function (likely through protein conformation changes) and induce signaling. The E93A mutation in the TIR domain abolishes the TIR enzymatic function, and SNC1-1E93A cannot induce signaling. However, SNC1-1E93A and SNC1 WT together induce signaling likely through their protein–protein interaction.
Genetic interaction of SNC1-1E93A and wild-type SNC1
We then asked whether or not endogenous wild-type SNC1 has any interaction with the two SNC1-1 forms expressed from the transgene. To this end, we generated SNC1-1-GFP and SNC1-1E93A-GFP transgenic plants in the snc1-11 and snc1-12 mutants. SNC1-1-GFP had a lower plant inhibition score in snc1-11 and snc1-12 (80% and 101%) than in Col-0 (153%) (Figure 5C), suggesting that SNC1-1-GFP activity might be dependent on the endogenous wild-type SNC1. Strikingly, the SNC1-1E93A-GFP had a much lower plant inhibition score in snc1-11 (7%) and snc1-12 (10%) than SNC1-1E93A-GFP in Col-0 (131%) (Figure 5C). The low growth inhibition activity in the snc1 LOF mutants was also reflected in the absence of transgenic plants of the SNC1-1E93A-GFP/snc1-11 and SNC1-1E93A-GFP/snc1-12 in groups C and D (Figure 5C).
We further examined the disease resistance properties of SNC1-1-GFP/Col, SNC1-1-GFP/snc1-11, SNC1-1E93A-GFP/Col, and SNC1-1E93A-GFP/snc1-11 transgenic plants to Pst DC3000. Multiple transgenic lines (six to nine each) were selected from the A group for each of SNC1-1-GFP/Col, SNC1-1-GFP/snc1-11, SNC1-1E93A-GFP/Col, and SNC1-1E93A-GFP/snc1-11. Growth of Pst DC3000 was lower in the SNC1-1-GFP/Col and SNC1-1E93A-GFP/Col transgenic plants compared with the WT, indicating that both SNC1-1-GFP and SNC1-1E93A-GFP transgenes enhance immune responses in the WT background (Figure 5D). Interestingly, the growth of Pst DC3000 was higher in the SNC1-1E93A-GFP/snc1-11 transgenic plants compared with the SNC1-1-GFP/snc1-11 transgenic plants, to a similar level as in the WT, suggesting SNC1-1E93A-GFP could not enhance immunity without the endogenous wild-type SNC1 (Figure 5, D and E). Taken together, these data suggest that the NADase activity or the E93 residue of SNC1-1 is required for its function in plant immunity.
Discussion
SNC1 is an important NLR protein in Arabidopsis contributing to autoimmunity caused by mutations in a large number of genes. Despite the intensive studies on SNC1 gene regulation and its important contribution to autoimmunity, the role of SNC1 in natural plant–pathogen interactions has been enigmatic. In this study, we established the role of SNC1 in accelerating and enhancing effector-triggered immune responses including defense gene induction and HR. SNC1 contributes to disease resistance induced by a number of effectors of P. syringae, and its function is more pronounced under constant light conditions. Furthermore, we show that the SNC1–SNC1 protein interaction and the catalytic residue glutamate in the TIR domain are critical for its signaling activity.
Findings from this study shed insight into the role of the wild-type SNC1 in plant–pathogen interactions. As an NLR protein, SNC1 could function as (1) a sensor NLR for effector detection and signaling initiation, (2) a helper NLR for transducing signal, or (3) an NLR with other functions. Evidence supporting SNC1 as a sensor NLR as in hypothesis 1 is weak. SNC1 is a positive regulator of resistance to Pst DC3000 AvrRps4 in the absence of RPS4 (Kim et al., 2010), suggesting that it may function as a minor sensor for AvrRps4. However, the loss of SNC1 function, unlike the loss of sensor NLRs, does not abolish ETI, and the snc1 LOF mutants only reduce ETI that is induced by AvrRpt2, AvrRps4, or HopAE1 (Figures 1 and 2). Its function in ETI is light dependent, which is not expected from a sensor NLR. However, it remains possible that SNC1 is responsible for detecting some unknown effectors, or it functions redundantly with other NLRs as a sensor NLR. It is not likely that SNC1 is a classical helper NLR as defined by mediating signaling from sensor NLRs such as NLRs in the ADR1 and NRG1 families. SNC1 does interact with EDS1 (ENHANCED DISEASE SUSCEPTIBILITY 1), similar to other helper NLRs (Bhattacharjee et al., 2011; Sun et al., 2021; Wu et al., 2021). If so, it would play a minor role compared with other helpers because its effect on ETI is small. Further genetic interaction analysis between SNC1 and helper NLRs might shed light on this potential role for SNC1. However, the function of SNC1 may require helper NLRs. The gain-of-function snc1-1 mutant requires the helper ADR1 and NRG1 for its signaling (Wu et al., 2019), suggesting that the helper NLRs function downstream of the active form of SNC1. Therefore, SNC1 may not be a helper NLR as defined now. Alternatively, immune signaling may use multiple relaying steps each of which involves distinct groups of helpers, and SNC1 is a helper that functions at a different relaying step from the classical helper NLRs. 3) It is possible that SNC1 functions as a signaling amplifier, which is not required for initiating immune responses but is important for the amplitude of immune responses. The snc1 LOF mutants have a reduced and delayed defense gene expression in response to Pst DC3000 AvrRpt2 (Figure 3). SNC1 may function as a transcriptional coregulator in immune responses. Early study reveals that the nuclear localization of SNC1 is crucial for its activity in plant immunity (Zhu et al., 2010a, 2010b; Xu et al., 2014; Lüdke et al., 2021; Jia et al., 2021). The nuclear-localized SNC1 could interact with TOPLESS proteins to regulate downstream immune responses (Cai et al., 2018; Griebel et al., 2020; Garner et al., 2021). In addition, SNC1 interacts with EDS1 (Bhattacharjee et al., 2011) which was recently found to act as a transcriptional coactivator in defense gene regulation (Chen et al., 2021). Further biochemical characterization of the SNC1 protein and the genetic analysis of the mutant might differentiate these models.
This study also found that the enzymatic function of the TIR domain is required for its immune signaling function. The snc1-1 gain-of-function mutation causes autoimmunity, likely due to the activation of its signaling activity brought about by conformation changes induced by this mutation in the junction region of the NBS domain and LRR domain. This signaling activity in Arabidopsis is dependent on the catalytic site E93, as the SNC1-1E93A form could not induce autoimmunity in the snc1 LOF mutants (Figure 5). We cannot determine which one or two enzymatic activities this residue is important for with this E93A mutation. In addition to the NADase activity, the TIR of SNC1 may also possess 2′,3′-cAMP/cGMP synthetase activity similar to the TIR-only protein RBA1 (RESPONSE to HopBA1) and the TNL protein L7 (Yu et al., 2022a, 2022b). Recently, Tian et al. showed that the NADase activity but not the potential 2′,3′-cAMP/cGMP synthetase activity was indispensable for SNC1-mediated autoimmunity in Arabidopsis (Tian et al., 2022). This is consistent with the recent findings that the NADase-generated small molecules are required for the formation of downstream signaling complexes (Huang et al., 2022; Jia et al., 2022).
Genetic analysis of this study strongly suggests the formation of a SNC1–SNC1 protein complex and an intermolecular activation in the protein complex. The E93A mutant form SNC1-1 as a transgene was able to induce autoimmunity in the WT plants but not the snc1 LOF mutant. This can result from the formation of a hetero oligomer between the SNC1-1E93A form and the SNC1 WT form, neither of which alone induce autoimmunity. It is likely that the snc1-1 mutation induces an active conformation not only in SNC1-1E93A itself but also in its interacting wild-type SNC1 in the oligomer. The activated wild-type SNC1 that has intact enzymatic activity could activate downstream immune responses (Figure 5E). Consistent with this model, protein interaction between SNC1 and itself was previously observed in the co-IP assay (Xu et al., 2014).
This study revealed that SNC1 has a light-dependent regulation of immune responses. The snc1 LOF mutants have reduced defense gene expression in basal resistance and a reduced ETI to Pst DC3000 AvrRps4 and Pst DC3000 AvrHopAE1 when plants are grown under the constant light condition but not a 12-h light condition (Figure 1). Plant disease resistance to Pst DC3000 is reduced by a shortened day length (Gangappa et al., 2017; Gangappa and Kumar, 2018). Longer light exposure after pathogen infection generally induces a stronger SA-associated systemic acquired resistance (Liu et al., 2011). The preferential function of SNC1 under constant light suggests that SNC1 is involved in the enhancement of SA signaling by light. Indeed, the early induction of SA biosynthesis and responsive genes was reduced and delayed in the snc1 LOF mutants under the constant light condition (Figure 3). The role of SA signaling enhancement is consistent with the model that SNC1 acts as a transcriptional regulator of defense responses. Alternatively, the light- or photoperiod-dependent function of SNC1 might reflect the regulation of SNC1 gene itself. A master circadian regulator LATE ELONGATED HYPOCOTYL 1 (LHY1) is implicated in SNC1 transcript regulation (Yu et al., 2022a, 2022b), so it is possible that SNC1 gene expression is light dependent, and its function is more pronounced when it has a high expression. Future genetic and biochemical studies of SNC1 should further reveal its role and mode of action in plant immunity.
Materials and methods
Plant materials and growth condition
Arabidopsis (A. thaliana) accession Col-0 was the wild-type for all mutants in this study. The T-DNA insertion mutants SALK_047058 (snc1-11) and SALK_052814 (snc1-12) were described previously (Alonso et al., 2003; Yang and Hua, 2004). All plants were grown at 22°C under constant (24 h) light (∼100 µmol m−2 s−1 of fluorescent lamp) or under the 12-h light/12-h dark (12 h light) condition and 50% humidity conditions.
Plasmid construction and transgenic plants generation
The pSNC1:SNC1-1:GFP plasmid was described earlier (Zhu et al., 2010a, 2010b). For the construction of the pSNC1:SNC1-1-E93A:GFP plasmid, the E93A mutation was introduced into the pSNC1:SNC1-1:GFP binary vector (Zhu et al., 2010a, 2010b) by PCR amplification with primers with desired mutations and the use of ClonExperess II One Step Cloning kit (Vazyme, Cat. No. C112). Primers used are listed in Supplemental Table 2. Agrobacterium tumefaciens strain GV3101 (pMP90) carrying the desired construct was used for floral dipping to generate transgenic plants or for transient expression in N. ben. plants.
Pathogen growth assay
For the Pst DC3000 pathogen growth assay, a bacterial suspension at OD600 of 0.05 in 10 mM MgCl2 and 0.02% (v/v) Silwet L-77 was dip-inoculated on the shoots of 14-day-old seedlings grown in a 12-h light condition or on the shoots of 12-day-old seedlings grown under constant light. Bacterial growth was analyzed at 1 h (0 day) post-inoculation and 3 days post-inoculation (dpi). The shoots of plants were weighted and homogenized in 10 mM MgCl2, and bacteria were counted by plating serial dilutions as described earlier (Yang et al., 2020). Three individual plants from one pot were pooled as one biological replicate. At least three biological replicates from different pots were performed.
Reverse transcription quantitative PCR (Rt-qPCR)
For RT-qPCR, total RNA was extracted from whole seedlings using TRIzol reagent. cDNA was synthesized from 0.5 µg RNA using the PrimeScript RT Reagent Kit with gDNA Eraser (Takara, Cat. No. RR047A). RT-qPCR was performed on a QuantStudio 7 Pro instrument (ThermoFisher Scientific) using iQ SYBR Green supermix (Bio-Rad, Cat. No. 1708880). Three individual plants from one pot were pooled as one biological replicate and at least three biological replicates from different pots were performed. Primers used for RT-qPCR are listed in Supplemental Table 2.
Measurement of electrolytic conductivity
The electrolytic conductivity measuring cell death was performed as previously described (Hatsugai and Katagiri, 2018) with modifications. Briefly, avirulent strain Pst DC3000 AvrRpt2 or Pst DC3000 AvrRps4 (OD600 of 0.1 with 10 mM MgCl2) was infiltrated into the 7th and 8th leaves of three-week-old plants grown under 24 h light condition. Two leaf discs from one plant were pooled as one biological replicate and four biological replicates from different plants were performed. Leaf discs were floated in sterilized ultrapure water and the electrolytic conductivity was measured with a LAQUAtwin EC-22 conductivity meter (Horiba).
Measurement of flg22 triggered ROS production
Elicitor flg22 triggered ROS production was measured by a luminol-based assay as described earlier (Sang and Macho, 2017). The 5th and 6th leaves of a two-week-old plant grown under constant light were used.
Statistical analysis
Two-tailed Student's t-tests were performed using Excel. One-way analysis of variance (ANOVA) followed by Duncan's new multiple range tests were performed using R 3.6.3 with the “agricolae” package (https://cran.r-project.org/web/packages/agricolae/). Statistical tests and biological replicate numbers are described in the figure legends or methods.
Accession numbers
DNA sequences of all genes in this study were extracted from The Arabidopsis Information Resource (TAIR, https://www.arabidopsis.org/) with the gene ID listed below: SNC1 (AT4G16890); SID2 (AT1G74710); ACTIN2 (AT3G18780); PAD4 (AT3G52430); PR1 (AT2G14610); FRK1 (AT2G19190); and WRKY29 (AT4G23550).
Supplemental data
The following materials are available in the online version of this article.
Supplemental Figure S1. The loss of function mutants of SNC1 are more susceptible to avirulent strains of Pst DC3000, supporting Figure 1.
Supplemental Table S1. Effectors and their cognate NLRs used in this study.
Supplemental Table S2. Primers used in this study.
Acknowledgments
We thank Dr. Greg Martin at Boyce Thompson Institute for sharing the avirulent strains of Pst DC3000.
Funding
This work has been supported by National Science Foundation USA (NSF) IOS-1946174 to JH.
References
Author notes
Present address: State Key Laboratory of Crop Genetics and Germplasm Enhancement, College of Agriculture, Nanjing Agricultural University, Nanjing 210095, China.
J.H. conceived this study. Z.W. and L.Y. performed the experiments. Z.W., L.Y., and J.H. wrote the manuscript.
The author responsible for distribution of materials integral to the findings presented in this article in accordance with the policy described in the Instructions for Authors (https://dbpia.nl.go.kr/plphys/pages/General-Instructions) is: Jian Hua ([email protected]).
Conflict of interest statement. The authors declare no conflict of interest for this work.