-
PDF
- Split View
-
Views
-
Cite
Cite
David S Domozych, Kaylee Bagdan, The cell biology of charophytes: Exploring the past and models for the future, Plant Physiology, Volume 190, Issue 3, November 2022, Pages 1588–1608, https://doi.org/10.1093/plphys/kiac390
- Share Icon Share
Abstract
Charophytes (Streptophyta) represent a diverse assemblage of extant green algae that are the sister lineage to land plants. About 500–600+ million years ago, a charophyte progenitor successfully colonized land and subsequently gave rise to land plants. Charophytes have diverse but relatively simple body plans that make them highly attractive organisms for many areas of biological research. At the cellular level, many charophytes have been used for deciphering cytoskeletal networks and their dynamics, membrane trafficking, extracellular matrix secretion, and cell division mechanisms. Some charophytes live in challenging habitats and have become excellent models for elucidating the cellular and molecular effects of various abiotic stressors on plant cells. Recent sequencing of several charophyte genomes has also opened doors for the dissection of biosynthetic and signaling pathways. While we are only in an infancy stage of elucidating the cell biology of charophytes, the future application of novel analytical methodologies in charophyte studies that include a broader survey of inclusive taxa will enhance our understanding of plant evolution and cell dynamics.
Introduction
If you walk the paths near the thermal outflows at Yellowstone National Park, or gaze at the purple algal mats found in the Tyrol region of the Alps, or crunch through the biological soil crusts of Arizona deserts or simply note the vibrant green floating masses found in ephemeral pools, you have met charophytes. If you could go back in time, let us say 500–600+ million years ago (mya), and wander along the edges of ancient freshwater wetland or even possibly the thawed edge of a glacier, you might also encounter a charophyte and possibly one that has an impressive preadapted tool kit that will allow it to colonize land and ultimately give rise to land plants (de Vries and Archibald, 2018; Schreiber et al., 2022; Žárský et al., 2022). The charophytes (or “basal” streptophytes) are a diverse group of green algae that have intrigued plant biologists for decades and now provide exciting opportunities for future studies in many areas of biology. Charophytes have piqued this interest because they are: (1) the key algal group that yielded land plants, an event that profoundly changed the biogeochemistry and natural history of the planet as well as serving as a basis for human cultural evolution; (2) important residents of many modern day aquatic and terrestrial habitats with much to reveal about stress acclimation in green plants; and (3) valuable yet underused experimental models for deciphering many of the intricacies of plant cell dynamics.
Prior to the late 1960s, the phylogeny of the green algae was not well resolved. The application of transmission electron microscopy (TEM) in green algal studies by ground-breaking pioneers Jeremy Pickett-Heaps and Marchant (1972), Pickett-Heaps (1975), Mattox and Stewart (1984), and others paved the way for a host of other researchers and the emergence of a paradigm in phycology, that is, the charophytes are the assemblage of green algae that are most closely related and ancestral to land plants. During this time, various charophyte taxa were also used in nonphylogenetically themed studies including those based in the studies of gravitropism, photomorphogenesis, and cell biology. There has been a major upsurge in charophyte-based research publications over the past 5+ decades, and recently sequenced genomes and transcriptomes of several taxa (see Table 1) have substantially refined if not transformed our knowledge of these algae. These now form a solid foundation for the next level of charophyte studies especially at the cellular and subcellular levels. Additionally, charophytes have some distinct features that make them outstanding model organisms for plant and algal cell biology (Domozych et al., 2017) including: (1) simple body plans (e.g. unicell or simple multicellular thallus); (2) a relative ease in culturing and laboratory experimentation; (3) being amenable to live cell labeling with antibodies and other labeling probes; and (4) being relatively simple to prepare cells for diverse technologies including rapid screening of targeted probes (e.g. chemical biology) and high-resolution microscopy imaging and analysis.
Group and specific taxon . | Source . |
---|---|
Mesostigmatophyceae | Wang et al. (2020), doi: 10.1038/s41477-019-0560-3; |
Mesostigma viride | Liang et al. (2019), doi:_ 10.1002/advs.201901850 |
Chlorokybophyceae | Irisarri et al. (2021), doi: 10.1098/rspb.2021.2168;Wang et al. (2020), doi: 10.1038/s41477-019-0560-3 |
Chlorokybus atmophyrticus and other Chlorokybus isolates | |
Klebsormidiophyceae | Hori et al. (2014), doi: 10.1038/ncomms4978 |
Klebsormidium flaccidum | |
Charophyceae | Nishiyama et al. (2018), doi: 10.1016/j.cell.2018.06.033 |
Chara braunii | |
Zygnematophyceae | Cheng et al. (2019), doi: 10.1016/j.cell.2019.10.019 |
Spirogloea muscicola | |
Zygnematophyceae | Cheng et al. (2019), doi: 10.1016/j.cell.2019.10.019 |
Mesotaenium endlicherianum | |
Zygnematophyceae | Jiao et al. (2020), doi: 10.1016/j.cell.2020.04.019 |
Peniun maragaritaceum |
Group and specific taxon . | Source . |
---|---|
Mesostigmatophyceae | Wang et al. (2020), doi: 10.1038/s41477-019-0560-3; |
Mesostigma viride | Liang et al. (2019), doi:_ 10.1002/advs.201901850 |
Chlorokybophyceae | Irisarri et al. (2021), doi: 10.1098/rspb.2021.2168;Wang et al. (2020), doi: 10.1038/s41477-019-0560-3 |
Chlorokybus atmophyrticus and other Chlorokybus isolates | |
Klebsormidiophyceae | Hori et al. (2014), doi: 10.1038/ncomms4978 |
Klebsormidium flaccidum | |
Charophyceae | Nishiyama et al. (2018), doi: 10.1016/j.cell.2018.06.033 |
Chara braunii | |
Zygnematophyceae | Cheng et al. (2019), doi: 10.1016/j.cell.2019.10.019 |
Spirogloea muscicola | |
Zygnematophyceae | Cheng et al. (2019), doi: 10.1016/j.cell.2019.10.019 |
Mesotaenium endlicherianum | |
Zygnematophyceae | Jiao et al. (2020), doi: 10.1016/j.cell.2020.04.019 |
Peniun maragaritaceum |
Major transcriptome studies: Van de Poel et al., Spirogyra pratensis transcriptome 10.1104/pp.16.00299; One thousand transcriptomes 10.1038/s41586-019-1693-2. For information on Spirotaenia’s position in the charophytes, see Irissarri et al., 2021.
Group and specific taxon . | Source . |
---|---|
Mesostigmatophyceae | Wang et al. (2020), doi: 10.1038/s41477-019-0560-3; |
Mesostigma viride | Liang et al. (2019), doi:_ 10.1002/advs.201901850 |
Chlorokybophyceae | Irisarri et al. (2021), doi: 10.1098/rspb.2021.2168;Wang et al. (2020), doi: 10.1038/s41477-019-0560-3 |
Chlorokybus atmophyrticus and other Chlorokybus isolates | |
Klebsormidiophyceae | Hori et al. (2014), doi: 10.1038/ncomms4978 |
Klebsormidium flaccidum | |
Charophyceae | Nishiyama et al. (2018), doi: 10.1016/j.cell.2018.06.033 |
Chara braunii | |
Zygnematophyceae | Cheng et al. (2019), doi: 10.1016/j.cell.2019.10.019 |
Spirogloea muscicola | |
Zygnematophyceae | Cheng et al. (2019), doi: 10.1016/j.cell.2019.10.019 |
Mesotaenium endlicherianum | |
Zygnematophyceae | Jiao et al. (2020), doi: 10.1016/j.cell.2020.04.019 |
Peniun maragaritaceum |
Group and specific taxon . | Source . |
---|---|
Mesostigmatophyceae | Wang et al. (2020), doi: 10.1038/s41477-019-0560-3; |
Mesostigma viride | Liang et al. (2019), doi:_ 10.1002/advs.201901850 |
Chlorokybophyceae | Irisarri et al. (2021), doi: 10.1098/rspb.2021.2168;Wang et al. (2020), doi: 10.1038/s41477-019-0560-3 |
Chlorokybus atmophyrticus and other Chlorokybus isolates | |
Klebsormidiophyceae | Hori et al. (2014), doi: 10.1038/ncomms4978 |
Klebsormidium flaccidum | |
Charophyceae | Nishiyama et al. (2018), doi: 10.1016/j.cell.2018.06.033 |
Chara braunii | |
Zygnematophyceae | Cheng et al. (2019), doi: 10.1016/j.cell.2019.10.019 |
Spirogloea muscicola | |
Zygnematophyceae | Cheng et al. (2019), doi: 10.1016/j.cell.2019.10.019 |
Mesotaenium endlicherianum | |
Zygnematophyceae | Jiao et al. (2020), doi: 10.1016/j.cell.2020.04.019 |
Peniun maragaritaceum |
Major transcriptome studies: Van de Poel et al., Spirogyra pratensis transcriptome 10.1104/pp.16.00299; One thousand transcriptomes 10.1038/s41586-019-1693-2. For information on Spirotaenia’s position in the charophytes, see Irissarri et al., 2021.
The charophytes groups
The charophytes are part of the Streptophyta, a monophyletic clade within the Viridiplantae that includes both the charophytes and land plants (Mattox and Stewart, 1984; Bremer, 1985; Delwiche and Cooper, 2015; Rensing, 2018). Extant charophytes are currently placed in two grades (de Vries and Archibald, 2018), the KCM grade, consisting of the Klebsormidiophyceae, Chlorokybophyceae, and Mesostigmatophyceae, the “early divergent” or lower branching charophytes. The ZCC grade consists of the Zygnematophycaneae, Coleochaetophyceae, and Charophyceae or the “later divergent” or higher branching charophytes. Figure 1 provides an introduction to each group, and detailed information about charophyte body plans, development, reproduction, and ecology may be obtained in Graham et al. (2016). It is now thought that all land plants evolved from a single streptophyte algal progenitor 500–600+ million years ago (Fürst-Jansen et al., 2021), and phylogenomic analyses have now clearly confirmed that the Zygnematophyceae are sister to embryophytes (Delwiche and Cooper, 2015; Harholt et al., 2016; de Vries and Archibald, 2018; Puttick et al., 2018; Zhou and von Schwartzenberg, 2020; Hess et al., 2022).
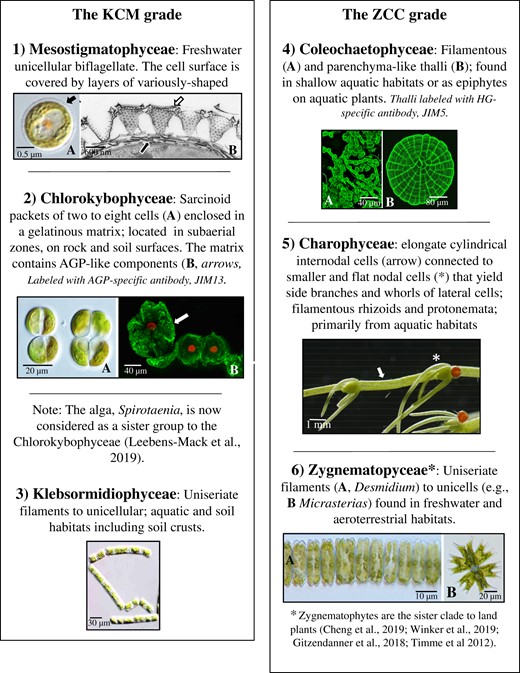
Overview of the charophyte grades in current classification. LM, light microscopy; HG, homogalacturonan; JIM5: John Innes monoclonal antibody 5-specific for HG.
The cell biology of charophytes
The architectural design and functional interactions of the subcellular components of individual cells of different charophytes taxa are very diverse. Highlights of the cell biology of charophytes are presented below.
Nucleus
Much of what is known about the structure and dynamics of the nucleus in charophytes comes from early cytological and ultrastructural studies, especially those focused upon mitosis (Pickett-Heaps, 1975; Brook, 1981). More details of the nuclei of specific taxa will be found in studies dealing with cell division (see “Cell division”) and for a comparative study of charophyte nuclei in light of their life cycles one should read Haig (2010).
Two of the more distinct types of nuclei-based features in charophytes are found in Chara (Charophyceae) and in many zygnematophytes (e.g. Micrasterias). In Chara, each mature internodal cell may possess several thousand nuclei that are located in the streaming endoplasm (Foissner and Wasteneys, 2014). These nuclei and those in other cell types are typically products of “amitosis,” a process where rapid structural changes to nuclei occur during the cell cycle (Vouilloud et al., 2007). In some zygnematophyte taxa, a distinct migration of postmitotic nuclei takes place during cell division (Meindl et al., 1994; Holzinger and Lütz-Meindl, 2002; Oertel et al., 2003). The nucleus passively migrates into the growing semicell and then migrates via microtubule and myosin mediation into the isthmus of the daughter cell.
There have also been recent advances in methodology for in vitro studies using nuclei. Intact nuclei along with other organelles have been isolated from Chara internodal cells that can then be used for subsequent experimentation (Gylytė et al., 2021). Pure fractions of nuclei obtained from protoplasts of Penium margaritaceum (Raimundo et al., 2018; Domozych et al., 2020a, 2020b) allowed for the isolation of nuclear DNA that was subsequently employed for sequencing of its genome (Jiao et al., 2020). The isolation of nuclei and nuclear genome size estimation of Spirogyra and Zygnema have recently been enhanced via in vitro analyses (Feng et al., 2021; Čertnerová, 2021).
Mitochondria
Mitochondrial architecture in charophytes is similar to that observed in land plants and only a few detailed structural studies of these organelles in charophytes have been performed. In Chara, mitochondrial size, shape, and distribution vary depending on cell age and environmental conditions (Foissner and Wasteneys, 2014). The mitochondria of the large internodal cells are equipped with both myosin motors and kinesin that allow them to translocate in the cell. In Micrasterias that is exposed to ionic stress via application of KCl, NaCl, or CoCl2, mitochondria fuse to form distinct, localized 3D networks (Steiner et al., 2020; Darehshouri et al., 2020). These high salinity treatments induce the formation of pronounced protuberances of the outer mitochondrial membrane with concurrent mitochondrial elongation and fusion (Affenzeller et al., 2009a). It is also important to note that sequencing of mitochondrial genomes of various taxa (Turmel et al., 2013) has been critical to early phylogenetic studies of charophytes.
Chloroplasts
Chloroplasts have been focal points of study in many areas of charophyte research. Their structural and functional evolution are considered to be key adaptations for successful terrestrialization (de Vries et al., 2016; Fürst-Jansen et al., 2021). Comparative analyses of the charophyte chloroplast genomes have also been important for evolutionary studies and details may be obtained in the following papers (Civaň et al., 2014; Lemieux et al., 2016; Gitzendanner et al., 2018). Insight into rubulose 1–5 biphosphate carboxylase, carbon-concentrating mechanisms in the plastid, and the functional role of pyrenoids is also available in Goudet et al. (2020).
Mesostigma (Mesostigmatophyceae) possesses a plate-like chloroplast and a pigment profile that is notably different from that found in plants and other green algae (Yoshii et al., 2003). An eyespot made of rows of pigment globules is found in the chloroplast nearest the flagellar basal bodies and functions in phototaxis (Kreimer, 2009). In this process, the excitation of the sensory rhodopsins, specifically channel rhodopsins, generates transmembrane photoreceptor currents (i.e. they act like light-gated cation channels) that are part of the cell’s sensory movement in response to light (Govorunova et al., 2022, 2011).
In the Chlorokybophyceae, Klebsormidiophyceae, and Coleochaetophyceae, most cells contain simple chloroplasts, each with pyrenoids (Graham et al., 2016). Substantial structural changes to chloroplast structure and photosynthesis-based activity in Klebsormidium have been described under various abiotic stressors (Holzinger et al., 2011; Kaplan et al., 2012; Karsten and Holzinger, 2014, 2012; Holzinger and Pichrtová, 2016).
In the Zygnematophyceae, most cells contain one or two chloroplasts, each typically with one or more pyrenoids. The shape of the chloroplast is taxon-specific, ranging from flat to star-shaped to spiral, and in some desmids, the plastid is nearly the same size and shape of the cell (Hall and McCourt, 2005). In some zygnematophytes like Mougeotia, chloroplasts move in response to specific light-induced signals that include both phytochrome and blue light receptors (Gabryś, 1985; Kianianmomeni and Hallmann, 2014). Substantial changes to chloroplast structure and biochemistry have also been elucidated in cells grown under UV stress (Holzinger and Lütz, 2006). Heat stress in Spirogyra praetensis results in notable changes to the chloroplast including the loss of its characteristic spiral arrangement and changes in pigmentation (de Vries et al., 2020).
In the internodal cells of Chara, chloroplasts are arranged in helical files that are either oriented in right- or left-handed vectors (Foissner and Wasteneys, 2014). They are firmly attached to the cell periphery and do not change position in response to light. During cell development, multiple interactions between chloroplasts also occur (Bulychev and Rybina, 2018).
The endomembrane system, secretory dynamics, and membrane trafficking
Charophytes possess diverse and often highly complex endomembrane systems and membrane trafficking networks that “process” (i.e. synthesize, package, transport, and retrieve) a wide array of cargo. The unicellular Mesostigma viride secretes several types of highly ornate scales that cover both the cell and flagella membranes (Manton and Ettl, 1965). The main components of these scales are primarily neutral and acidic sugars including 2-keto sugars such as 3-deoxy-lyxo-2-heptulosaric acid and small amounts of protein (Domozych et al., 1991; Becker et al., 1994, 1995). These scales also complex calcium in the form of calcium phosphate. TEM imaging has demonstrated that scales are processed within the Golgi Apparatus, transported to a vacuole-like scale reservoir, and ultimately secreted onto the cell surface (Domozych et al., 1992). The precursors of the large and spectacular basket scales are first seen in the peripheries of the Golgi cisternae of the medial zone of the Golgi cisternal stack. Fully assembled basket scales appear in the trans face cisternae and in large vesicles that emerge from these cisternae. Vacuoles situated near the Golgi body contain stores of Ca2+ that very likely are used in scale processing. Scale development in this and other scale-bearing algae has been critical in resolving previous “competing” theories of the movement of cargo through the Golgi cisternal stack. Scales develop via the “cisternal progression” model (Glick and Luini, 2011) as anterograde transport of the scales does not entail peripheral vesicles surrounding the Golgi stack (Melkonian et al., 1991; Becker et al., 1995).
Zygnematophytes also possess some of the most distinct membrane trafficking networks within the charophytes. This is very likely a manifestation of the large amounts and diverse types of extracellular matrix (ECM) materials that they synthesize and secrete. Ultrastructural studies of unicellular desmids like Micrasterias denticulata (Lütz-Meindl, 2016), Peniummargaritaceum (Domozych et al., 2020a, 2020b), Netrium digitus (Eder and Lütz-Meindl, 2010), and others have revealed complex endomembrane architecture and secretory dynamics, some of which are very different from that observed in land plants. These studies have been supplemented with data from experimental interrogation of particular endomembrane system components in cells treated with targeted chemical agents and/or grown under particular stress agents (Url et al., 1993; Höftberger et al., 1995; Lütz-Meindl, 2016; Domozych et al., 2020a, 2020b).
The most well-studied endomembrane component of zygnematophytes is the Golgi Apparatus that consists of several to more than one hundred Golgi bodies per cell. The arrangement of the Golgi bodies is highly organized and precisely positioned in the cell in relation to the cytoskeletal network, endoplasmic reticulum (ER), chloroplast, mitochondria, and nucleus. In P. margaritaceum, 100–150 Golgi bodies form linear arrays positioned deep in the cytoplasmic valleys created by the multilobed chloroplast (Domozych et al., 2020a, 2020b). Each Golgi body contains 12–15 flattened cisternae and with clearly defined cis- and trans faces. Large vesicles containing the mucilage-like extracellular polymeric substance or extracellular polysaccharides (EPS) emerge from the peripheries of trans face cisternae. Smaller vesicles carrying cell wall materials emerge from the trans face and the trans-Golgi network (TGN). During periods of high secretory activity, adjacent Golgi bodies often fuse with each other at the cisternal peripheries of the medial and trans regions. Unlike what has been reported in many land plants, Golgi bodies of Penium are not mobile. Rather, EPS and wall vesicles are carried from the Golgi body to the peripheral cytoplasm where they enter into thin cytoplasmic streaming “highways” located just under the plasma membrane. This cortical cytoplasmic streaming is actin-mediated and transports vesicles around the cell periphery. Cell wall cargo is secreted to the expanding central isthmus, while EPS-carrying vesicles fuse at various zones around the cell depending on where EPS secretion is needed. At present, little data are available describing the signaling mechanisms or the molecular architecture that define the changing secretion zones. Similar secretory machinery has also been observed in the zygnematophytes, Closterium acerosum, Pleurotaenium trabecula, Cosmarium reniforme, and M. denticulata (Domozych and Domozych, 1993; Oertel et al., 2004; Domozych et al., 2007, 2009a, 2009b). Here though, EPS vesicles traveling on the cortical cytoplasmic streaming channels appear to be directed to the inner part of the wall pore apparatuses that are found throughout the wall and penetrate the thick secondary cell wall.
Micrasterias denticulata has served as an important organism in resolving details of the structure and function of the Golgi Apparatus in zygnematophytes. Many important details of the alga’s subcellular architecture have been obtained employing high pressure freezing and freeze substitution technologies (Lütz-Meindl and Brosch-Salomon, 2000; Aichinger and Lütz-Meindl, 2005; Lütz -Meindl et al., 2015). Each Golgi body is 2–3 µm in size, consists of 11 cisternae and has clearly defined cis- and trans faces. Fifty- to seventy-nm vesicles derived from transitional regions (i.e. part rough/smooth) of adjacent ER transport membrane and inclusive cargo to the cis face Golgi body (Noguchi and Morré, 1991). Dark vesicles carrying wall cargo are found near the Golgi cisternal peripheries or at the TGN. The dark vesicles accumulate inside the plasma membrane at the growing lobes of the cell at specific fusion sites that correspond to sites of Ca2+ accumulation. Wall vesicle fusion with the plasma membrane and release of cargo contribute to cell morphogenesis including broadening of the cell body and the “multipolar” mechanism that forms the high symmetric lobing (Holzinger, 2000). Immunogold labeling studies have shown that pectic polysaccharides are packaged in the Golgi Apparatus and transported in high-esterified form to the cell surface (Eder and Lütz-Meindl, 2008). Arabinogalactan protein (AGP) and xyloglucan synthesis and secretion occur in the Golgi body and Golgi-derived vesicles, respectively (Eder et al., 2008). Pectins, AGPs, and nonAGP epitopes are transported together in the dark vesicles, while EPS is carried in large 400-nm vesicles in compact de-esterified form and with high Ca2+-binding capacity. Focused ion beam-Scanning Electron Microscopy (SEM) imaging has identified a large trans-Golgi sheath of ER that makes contact with the TGN and membrane of both the Golgi body and associated multivesicular bodies (MVBs; Wanner et al., 2013). The intracellular movement of secretory vesicles is fueled by actin and is critical for cell morphogenesis. EPS vesicles fuse with the plasma membrane at the cell wall pores but also appear to be able to release their cargo at any surface site through the cell wall layer (Oertel et al., 2004). Alteration of vesicle transport by targeted perturbation using optical tweezers causes irreversible malformations of the cell shape (Holzinger et al., 2002).
Micrasterias has also been used for elucidating the effects of stress on cell structure, development, and physiology including the endomembrane system. When incubated in medium with high levels of metals such as manganese or lead or placed in media that disturbs ionic homeostasis, Golgi bodies undergo a unique form of autophagy (Affenzeller et al., 2009a, 2009b; Lütz-Meindl, 2016). The cisternae transform into single membrane “balls” that appear to be absorbed by the ER. Incubation of cells in H2O2 results in programmed cell death (PCD) that then alters multiple subcellular systems including an increase in the number of ER compartments, abnormal dilation of cisternal rims of the Golgi bodies and the increased occurrence of MVBs (Darehshouri et al., 2008; Darehshouri and Lütz-Meindl, 2010). H2O2 levels are found to increase in cells kept under ionic and osmotic stress conditions and long term exposure to H2O2 causes an uptake of the multiple subcellular components by autophagy. Micrasterias, C. acerosum, and P. margaritaceum have also been used to study the specific effects of targeted inhibitors (e.g. brefeldin A, tunicamycin, and cyclopiazonic acid) on Golgi structure and dynamics (Höftberger et al., 1995; Salomon and Meindl, 1996; Domozych 1999a, 1999b; Domozych et al., 2020b).
Recent studies with transient transformed lines of zygnematophytes have revealed information about endomembrane components and secretion dynamics. Cellulose synthase (CesA) has been identified in particles concentrated at the plasma membrane of developing lobes during cell morphogenesis and Golgi bodies using transformed Micrasterias expressing MdCesA1-GFP fusion protein (M. denticulata cellulose synthase!; Vannerum et al., 2010). Rab GTPase, a key protein in vesicular trafficking in ER-Golgi transfer, secretion, endosomal activity, cytokinesis, and cell morphogenesis has been localized in the ER, Golgi bodies, exocytotic vesicles and in the isthmus zone in Micrasterias expressing using GFP-MdRABE1 (M. denticulata RABE1 GTPase; Vannerum et al., 2012). Sørensen et al. (2014) located the fluorescent reporter protein, red fluorescent protein (RFP), using a combination of an N–terminal SP from polygalacturonase 2, a tomato cell wall protein, and a C-terminal ER retention sequence in the ER network of P. margaritaceum. RNAi-suppressed expression of a pectin methylesterase homolog was also employed by Sørensen et al. (2014) to analyze pectin secretion and assembly.
One of the more notably distinct membrane trafficking systems in charophytes is found in the Charophyceae, more specifically in Chara australis or Chara corallina and several Nitella species. These organisms are macroscopic and consist of a series of long cylindrical internodal cells of up to 10 cm in length and small nodal cells from which side branches and whorls of lateral cells emerge (Foissner and Wasteneys, 2014). Two types of ER are found in the internodal cells, mobile endoplasmic ER that uses the endoplasmic actin/myosin network and cortical or ectoplasmic ER that uses microtubule-based mechanisms for organization and motility during early stages of cell elongation (Foissner et al., 2009; Sparkes et al., 2009). Mature internodal cells contain Golgi bodies that lie parallel to the ER and glide along the endoplasmic actin bundles (Foissner and Wasteneys, 2014). A TGN is found near the Golgi body and functions both as an endocytic and secretory organelle (Pesacreta and Lucas, 1984; Viotti et al., 2010). When internodal cells of C. australis are treated with BFA, Golgi bodies and TGN cluster together into “brefeldin compartments.”
A highly distinct membranous component of the internodal cells, nodal cells, protonemata, and rhizoids of Chara is the charasome (Foissner and Wasteneys, 2014; Foissner et al., 2015). This structure, a convoluted plasma membrane domain, comprised anastomosing membranous tubules that are continuous with the plasma membrane and measure ∼50 nm in length and 30 nm in diameter. Charasomes form in response to light via fusion of TGN-derived vesicles and local inhibition of endocytosis (Hoepflinger et al., 2017; Absolonova et al., 2019). Charasome formation is dependent on photosynthesis but does not require close contact with chloroplasts (Foissner et al., 2015). Charasomes contain H+-ATPases that are involved in the acidification of their aqueous environment and subsequent facilitation of photosynthesis-dependent carbon uptake (Sommer et al., 2015). The actin cytoskeleton, but not microtubules, is necessary for charasome growth as well as facilitating charasome degradation most likely by controlling trafficking of secretory and endocytic vesicles.
In aquatic habitats that harbor Chara, carbon is mainly found in the form which is poorly membrane permeable. The internodal cells acidify their surface either directly or via carbonic anhydrase to help facilitate the conversion of to membrane-permeable CO2 for increasing photosynthetic efficiency (Eremin et al., 2019). In order to maintain pH homeostasis of the cytoplasm, the proton efflux is balanced by either influx of H+ or efflux of OH− (Quade et al., 2022). The resulting pattern of alternating acid and alkaline regions or “bands” helps maintain metabolism (Beilby and Bisson, 2012). At the cell surface, areas with high charasome abundance stabilize and restrict the position of the acidic bands, whereas regions with low charasome abundance allow the formation of either stable or unstable alkaline bands (Pertl-Obermeyer et al., 2018; Absolonova et al., 2019). This acidification phenomenon has made Chara and other Charophycean taxa outstanding organisms for electrophysiology studies at the cellular level (Beilby and Casanova, 2014; Bulychev and Komarova, 2014; Beilby, 2016, 2019).
Chara also has a network of tip-growing rhizoids that recognize and adjust to gravity. At the expanding rhizoid tip is a special agglomeration of ER membranes, called the “Spitzenkorper” (Braun and Limbach, 2006). This subcellular component functions in guiding secretory vesicles that are needed for adjusting growth direction in response to gravity-induced statolith sedimentation.
Internal membrane components and secretory dynamics also play a key role during various types of wounding in Chara (Foissner and Wasteneys, 2011). During mechanical wounding, crystals and solid inclusions deposited in vacuoles are expelled from the wound zone due to the turgor and quickly occlude the hole in the cell wall (Menzel, 1988). This is followed by the synthesis of a two-layered cell wall at the wound zone. When cells are treated with Ca2+ ionophores which cause large influxes of Ca2+ at specific cell surface sites “compound exocytosis” occurs during which the products of endomembrane component fusion (e.g. ER with vesicles) are incompletely recycled by endocytosis. These are sent to and become part of the wound site. Likewise, exocytosis of vesicles carrying wall polysaccharides and membrane-bound cellulose/callose synthase are secreted at the wound site. The new cell wall material consists of pectin and callose and the membrane residues that are loaded with Ca2+ fill the wound site (Foissner, 1998, 1992, 1991). This represents a way for the cell to “detoxify” incoming Ca2+ by transferring Ca2+ to Ca2+-binding organelles and releasing them to the extracytoplasmic space at the wound site (Foissner and Wasteneys, 2011). Microtubules are not thought to be required in the wound response but their disassembly could have a signaling function. The transient modulation of the actin cytoskeleton into a meshwork of randomly oriented filaments is required for the transport of wall-forming organelles to the wound site (Foissner and Wasteneys, 2014).
Endocytosis
Endocytosis encompasses several membrane trafficking pathways that function in membrane retrieval and vesicle transport. This helps regulate cell expansion and provide a major route for the uptake of membrane proteins, lipids, and other extracellular molecules (e.g. signaling molecules) into the cell (Fan et al., 2015). Endocytosis initiates at localized microdomains on the plasma membrane and entails different vesicle types, most notably those coated with clathrin (Yao et al., 2022). Molecules brought into cell can be recycled or degraded using the plant cell’s endosomal organelles, TGN, and vacuoles (Robinson, 2015). Endocytosis in charophytes has primarily been studied in the Charophyceae (Sommer et al., 2021). Both fluid-phase and membrane dyes are actively taken up into the C. australis internodal cells by clathrin-mediated endocytosis. These dyes also stain various classes of endosomes including BFA- and wortmannin (WM)-sensitive organelles (i.e. TGN and MVBs; Sommer et al., 2021), thereby illustrating the paths of materials taken up by endocytosis. BFA treatment inhibits endocytosis and substantially decreases the number of clathrin-coated pits and clathrin-coated vesicles at the plasma membrane (Foissner et al., 2020). Clathrin and clathrin-coated vesicles play a role not only in constitutive endocytosis but also in the degradation of charasomes (Hoepflinger et al., 2017).
RAB5 GTPases (e.g. Arabidopsis [Arabidopsis thaliana] ARA7/RABF2b) are important regulators of endosomal membrane trafficking (Tripathy et al., 2021) and an ARA7 homolog (CaARA7/CaRABF2) has been isolated from Chara (Hoepflinger et al., 2015). Immunolabeling of the internodal cells revealed CaARA-epitopes located at the multivesicular endosomes (MVEs) and at MVE-containing WM compartments.
Vacuoles
Vacuoles represent a diverse array of single membrane-bound organelles that provide a wide variety of functions including maintaining turgor and serving as a lytic compartment for recycling and storing pigments, ions, proteins, carbohydrates, and metabolites (Marty, 1999). Vacuoles are also common components of charophyte cells and often take up substantial amounts of cell volume like that seen in land plants. Charophyte vacuoles have diverse morphology, development, and functions amongst the various subgroups.
Mesostigma contains both a branched vacuolar network, small smooth vacuoles that are reservoirs for Ca2+ and a scale reservoir where Golgi-derived scales are deposited, organized and ultimately released upon the plasma membrane surface (Manton and Ettl, 1965; Domozych et al., 1991). Zygnematophytes contain distinct vacuolar compartments. For example, many desmids contain large polar vacuoles positioned at the cell poles and a variety of smaller vacuoles found throughout the cytoplasm. Crystals of barium sulfate, strontium citrate, and other salts are often found in these vacuoles (Krejci et al., 2011a, 2011b; Niedermeier et al., 2018). In Zygnema and Zygogonium, the vacuoles are stores for phenolic compounds (Newsome and van Breeman, 2012; Aigner et al., 2013; Newsome et al., 2013; Herburger et al., 2016b) and are most notable in cells experiencing substantial abiotic stress. These phenolics are glycosylated, highly branched and form complexes with iron (Fe3+) that manifest in a purple color. These pigments may mask chlorophyll and provide a distinctive purple color to the filaments. The production of these pigments is thought to be in response to iron-induced oxidative stress as well as for protection against exposure to damaging UV light (Pichrtová et al., 2013; Newsome et al., 2012).
The large internodal cells of Chara have a central vacuole that occupies up to 95% of the cell volume and is overlaid by rapidly streaming cytoplasm that mixes most of the vacuolar contents (Oikawa et al., 2011; Foissner and Wasteneys, 2014). This vacuole appears to be used for storage rather than as a lytic organelle. In Nitella, the central vacuole contains solid inclusions made of a protein coat surrounding a polysaccharide core (Silverberg and Sawa, 1974).
Lipid bodies
Lipid bodies are subcellular components that are integrally associated with cellular homeostasis and modulate during metabolic development of a plant cell and in response to stress (Huang et al., 2019). In Zygnema spp. that occur in unstable polar terrestrial habitats that experience nutrient depletion, the accumulation of lipids may likely afford an advantage for survival (Pichrtová et al., 2016; Arc et al., 2020). In these environments, Zygnema forms mature vegetative cells called pre-akinetes that accumulate lipids in lipid bodies. This accumulation of lipid becomes a source of carbon for rapid growth that supplements photosynthetic carbon assimilation.
Autophagy
Autophagy is one mechanism that plant cells use to dispose of proteins, membranes, and organelles that become dysfunctional or are no longer needed (Marshall and Vierstra, 2018; Yoshimoto and Ohsumi, 2018). This process contributes to the regulation of plant growth, responses to environmental stress, and senescence/PCD (Wang et al., 2021). The most well-studied type of autophagy in plant cells is macroautophagy (Ding et al., 2018). Macroautophagy employs a unique double membrane compartment called an autophagosome that encloses a portion of the cytoplasmic constituents. The autophagosome then fuses with the vacuole membrane and the remaining single membrane structure called an autophagic body is transferred into the vacuole and broken down (Bassham, 2009).
In M. denticulata, salt stress, but not osmotic stress, induces macroautophagy (Affenzeller et al., 2009a). The process initiates by a swelling of ER compartments that subsequently surrounds organelles and eventually leads to the formation of autophagosomes (Affenzeller et al., 2009b). Autophagy accompanies other cell degradation processes including DNA laddering and mitochondrial disruption, all part of PCD (Lütz-Meindl, 2016). When Micrasterias is exposed to the photosynthesis-inhibiting herbicide, DCMU, to the glycolysis inhibitor, 2-Deoxy-d-glucose, and to complete darkness, lipid bodies form within the chloroplast (Schwarz et al., 2017). The lipid bodies are then released from the chloroplast where they become engulfed by double membranes derived from the ER and form autophagosomes. The autophagosomes then fuse with vacuoles. Molecular analysis of these stressed cells also reveals a single isoform of ATG8, the main regulator of both bulk and selective autophagy in eukaryotes. The degradation pathway of Golgi bodies in stressed Micrasterias does not appear to entail “classical” autophagy but occurs via disintegration of cisternae into masses that are absorbed by the ER (Lütz-Meindl, 2016).
Cytoskeleton
The diversity in cell size, shape, and development exhibited in charophytes is supported by distinct cytoskeletal architecture and dynamics. The study of charophyte cytoskeleton, specifically in Charophycean and Zygnematophycean taxa has had a long history in plant cell biology (see Foissner and Wasteneys, 2014; Lütz-Meindl, 2016).
Mesostigma viride displays a unique “flattened lifeboat” shape that is highlighted by a depression or pit from which two flagella arise (Manton and Ettl, 1965). The cortical cytoplasm including the pit is underlain by a parallel array of microtubules that very likely maintain the cell’s unique shape. The flagella are anchored to the cell by flagellar roots that include a multilayered structure, that is, a diagnostic feature of other charophytes (Rogers et al.,1981) including zoospores of Chlorokybus and Chaetosphaeridium and spermatozooids of Coleochaete (Moestrup, 1974; Graham and McBride, 1979; Rogers et al., 1980). The role of microtubules in the secretion and/or adhesion of the layers of body scales to the cell surface is not yet known.
Biological soil crusts are complex communities of surface microorganisms that create water-stable aggregates that have critical ecological roles in primary production, nitrogen cycling, mineralization, water retention, and stabilization of soils (Karsten and Holzinger, 2014). The charophyte, Klebsormidium, is a common resident of many of these habitats and must often deal with multiple and often severe abiotic stressors by modifying subcellular components like the cytoskeleton. When Klebsormidium grows under desiccation conditions, substantial changes to its microtubule and microfilament networks along with associated proteins have been observed. For example, F-actin disintegrates within 20 min of exposure to dehydration stress (Holzinger et al., 2014; Blaas and Holzinger, 2017). Transcriptomic studies of K. flaccidum and K. dissectum under cold and desiccation stress (Rippin et al., 2019) reveal that the expression of the Arp2/3 complex that is needed for actin filament formation, and kinesin, that is, a key motor protein of intracellular transport, both notably decrease. The Ca2+-binding actin-bundling protein required for the formation of actin bundles is also downregulated while the expression of the microtubule-severing enzyme, katanin, is enhanced. These major changes in cytoskeleton gene expression are thought to be part of the way that the organism prepares for rehydration during desiccation stress by protecting most of its cellular structures from damage and denaturation.
Taxa of the Coleochaetophyceae have been important organisms in analyzing the evolution of multicellularity in plants. Doty et al. (2014) revealed unordered microtubule patterns associated with diffuse growth during early cell expansion while in subsequent stages of elongating cells, tubulin cytoskeleton arrays similar to growth patterns associated with tip growth in other plants were noted.
In the multi-lobed Micrasterias, the cytoskeletal network has been shown to function in several phenomena (Lütz-Meindl, 2016; Felhofer et al., 2021). Two actin filament systems are present in an expanding cell (Meindl, 1985; Meindl et al., 1994; Pflügl-Haill et al., 2000). First, there is a cortical actin network that covers the inner surface of the cell and extends deep into the tips of the lobes in both the expanding and the nonexpanding semicell. This network also associates with the chloroplast surface. The second actin system ensheaths the nucleus at the isthmus-facing side during nuclear migration. Actin microfilaments and microtubules are key agents in moving wall and EPS vesicles from the Golgi body to the cell surface (Lütz-Meindl and Menzel, 2000; Oertel et al., 2004) most notably in the complex networks of F-actin-based cortical and transvacuolar cytoplasmic streaming. During cell division, microfilaments and microtubules form basket-like networks that attach to daughter nuclei and translocate them to the new semi-cells. The actin-binding protein, profilin, the actin-crosslinker, spectrin, kinesin-like proteins, and myosins are also involved in regulation and/or achievement of this nuclear and chloroplast movement in Micrasterias and other desmids (Holzinger et al., 2000; Holzinger and Lütz-Meindl, 2002; Oertel et al., 2003). The actin architecture is also critical for morphogenesis. Experimental alteration of this architecture by physical (e.g. laser) or chemical (actin-disrupting or -stabilizing drugs) or other stress agents result in notable alterations to cell shape (Meindl et al., 1994; Holzinger et al., 2002).
The microtubule and actin cytoskeleton are central to developmental phenomena in Spirogyra (Grolig, 1997; Schroder and Grolig, 1998). The cytoskeleton plays a central role in rhizoid formation in Spirogyra (Yoshida et al., 2003; Yoshida and Shimmen, 2009). Spirogyra filaments also glide and undulate when exposed to various wavelengths of light. Based on a comparison of filament cytoskeleton in cells exposed to red versus blue light, it was posited that this photomovement phenomenon is the result of a two-track control of microtubules and microfilaments signaled by the combination of phytochrome and phototropin-like receptors (Kim et al., 2005; Lee and Kim, 2017). Spirogyra has also served as a model for the development of the “cytoplast” theory of cytoplasm–cytoskeleton interactions (Pickett-Heaps et al., 1999). This theory posits that the “cell is a domain of cytoplasm (cytoplast) organized around the nucleus by a cytoskeleton consisting of a single ‘tensegral’ unit.” “Tensegrity” proposes that the cell is organized by an integrated cytoskeleton of tension elements, actin microfilaments, that extend over compression-resistant elements, the microtubules (Galli et al., 2005).
Charophycean taxa such as Chara and Nitella have been important organisms in elucidating cytoskeletal dynamics in plant cells due to their large size and ready ability to be perfused/microinjected with cytoskeleton-targeted agents. The presence of actin and myosin was reported in these algae before their discovery in land plants (Palevitz et al., 1974; Wasteneys et al., 1996; Foissner and Wasteneys, 2014) and in turn, has led to detailed studies of the cytoskeleton (Foissner et al., 2007, 2009; Woodhouse and Goldstein, 2013). The large internodal cells possess a meshwork of actin filaments and bundles that are arranged randomly and traverse the cell through transvacuolar strands (Wasteneys et al., 1996). Later in cell development, actin bundles become oriented in the subcortical cytoplasm parallel to the long axis. The cytoplasm constitutes of a 10-µm layer that lines the inner surface of the cell wall. The chloroplasts are organized in helical rows that spiral along the cell surface (Goldstein and van de Meent, 2015). Mitochondria are found in a stagnant layer of cytoplasm called the ectoplasm that surrounds the chloroplasts. At the inner part of the cytoplasm, the endoplasm, bundled actin filaments serve as tracts for myosins that propel subcellular structures around the cell in a streaming network. Myosin XI of Chara promotes streaming as fast as 100 m s−1 and is the fastest known in eukaryotes to date. Actin microfilaments display reversed polarity and the cytoplasm is, therefore, organized into two bands where cytoplasmic streaming occurs in opposite directions. In addition, Chara and Nitella, also have a distinct network of cortical microtubules. A notable association between the relative elemental elongation rates and transverse orientation of cortical microtubules matches patterns with cellulose microfibril orientation (Foissner and Wasteneys, 2014). The “branch form assembly” mechanism whereby new microtubules are initiated at discrete sites along the length of preexisting microtubules has been well-described in these algae (Murata et al., 2005).
Cell division
The recognition of charophytes as the progenitors of land plants, our understanding of phragmoplast-based cell division mechanisms and insight into the evolution of multicellularity in plants all stem in no small part from ultrastructural studies of cell division mechanisms that began over four decades ago (Pickett-Heaps, 1975; Mattox and Stewart, 1984). Taxa of the KCM grade possess relatively simple cell division mechanisms which may be a result of not having to construct new, large, and complex cell walls during the cell cycle. The scaly Mesostigma divides by simple furrowing (Manton and Ettl, 1965). Chlorokybus divides using centripetal plasma membrane invagination or cleavage furrow during cytokinesis and also uses centrosomes during mitotic spindle development (Lokhorst et al., 1988). The furrowing mechanism employs coated vesicles as well as transversely aligned cleavage microtubules. Klebsormidium and its relative, Interfilum, also divide by a centripetal cleavage furrow (Lokhorst and Star, 1985) that impinges on a persistent telophase spindle. In Klebsormidium, cell division features and their importance in taxonomic and evolutionary studies may be obtained in Mikhailyuk et al. (2014). Further details of cell division in Klebsormidium and their implications in taxonomic/phylogenetic studies are found in Mikhailyuk et al. (2014). In the related taxa, Entransia and Hormidella, the presence of periclinal centrioles has been described (Herburger et al., 2016a).
The cell division mechanisms of ZCC taxa have received much attention due to specific features that they share with land plants (Buschmann and Zachgo, 2016). An overview of the evolutionary trends observed in extant charophytes illustrates that the cytokinetic phragmoplast and preprophase band (PPB) of microtubules appeared, centrosomes disappeared, and in several groups, the cleavage furrow disappeared or was reduced.
The phragmoplast in most land plants is a microtubule-based structure that expands centrifugally (Smertenko et al., 2018) and contributes to the formation of the cell plate. Golgi-derived vesicles carrying cell wall materials collect at the phragmoplast, fuse and release their cargo which ultimately leads to the formation of a new cross wall between daughter cells (Drakakaki, 2015; Jawaid et al., 2022). In Chara, phragmoplast microtubules persist throughout cytokinesis and the cell plate forms across the whole cell at once (Cook et al., 1997). Cytokinesis initiates with an assemblage of smooth membrane tubules that are closely associated with ER and Golgi membranes. Plasmodesmata are formed during cytokinesis and are even observable in the incompletely formed reticulum of forming cell plates. Cell division has also been described in Coleochaete (Brown et al., 1994; Cook, 2004; Dupuy et al., 2010; Doty et al., 2014) and has been shown to entail a complex orientation of cytokinetic planes with polarized cytokinesis-based phragmoplasts. Phragmoplasts appear to develop among microtubules that emanate from the polar centrosomes rather than from nuclear envelopes and/or plastids.
Cell division in zygnematophytes was originally studied in Spirogyra (Fowke and Pickett-Heaps, 1969a, 1969b). This and subsequent studies have shown that there has been substantial reduction in the formation and use of the phragmoplast in zygnematophytes (Pickett-Heaps and Wetherbee, 1997). Phragmoplast formation in Spirogyra is initiated when the cleavage furrow contacts the microtubules of the telophase spindle. Cytokinesis begins with an ingrowing cleavage furrow and subsequently, actin-related determinants control cell-plate formation in the phragmoplast that arises from interzonal microtubules from the spindle. Recently, callose deposition and its putative role in the formation of the cell plate in Penium has been described (Davis et al., 2020). In many species of desmids (e.g. Micrasterias, Closterium, Netrium, and Cosmarium) and filamentous Zygnematalean taxa (Yoon et al., 2010; Regensdorff et al., 2018), the phragmoplast mechanism is totally eradicated and cleavage is re-established as the only device for dividing the cell.
The PPB is a ring-like structure that encircles the premitotic cell at the position where the new cross wall will be placed after mitosis in land plants (Schaefer et al., 2017). The PPB contains microtubules, F-actin, and ER and is also an active site of endocytosis. The PPB disassembles at the onset of mitosis, but leaves behind a “preprophase memory” that during cytokinesis attracts the phragmoplast. Similar cytoskeletal components have been found in many charophytes. In Micrasterias, a ring of microtubules at the cell isthmus is found encircling the cell at the site of future septum formation (Lütz-Meindl, 2016). In the filamentous Mougeotia, PPB-like microtubule rings in the cell cortex encircle the cell at the position of the premitotic nucleus (Galway and Hardham, 1991). These microtubules then disappear but mark the zone where cytokinesis will occur. The cortical cytoplasm of P. margaritaceum also contains a band of microtubules located at the cell isthmus (Ochs et al., 2014). This band is associated with a transient band of actin microfilaments and most likely helps to direct the deposition of new wall material as well as to mark the plane of the future cell division. Two additional satellite bands of microtubules arise from the original microtubular band and move to positions that mark of the future isthmus of daughter cells.
The ECM of the charophytes
One of the most intriguing features of charophytes is the diversity displayed in their extracellular matrix (ECM). Charophytes of the ZCC clade often possess cell wall constituents that are notably similar to those found in cell walls of many land plants (Popper et al., 2011; Sørensen et al., 2011, 2010; Domozych et al., 2012). These include cellulose, hemicelluloses, pectins, and glycoproteins like AGP (see Box 1). This has led to the supposition that charophytes evolved in terrestrial ecosystems (i.e. not in aquatic habitats), and their wall components were key preadaptations to successfully conquering land and the subsequent evolution of land plants (Harholt et al., 2016). Some charophytes though also produce very different if not spectacular ECMs whose chemistry, synthesis, and secretion from the cell are incompletely resolved. These include the layers of scales produced by Mesostigma and the recently described, Streptofilum capillatum (Mikhailyuk et al., 2018), as well as the mucilage- or slime-like extracellular polymeric substances (or EPS; see Box 2) produced by many taxa of the Zygnematophyceae. A detailed description of the ECM of charophytes is not possible here but a guide to components of the ECM and pertinent references are provided in Table 2.
Gravity, hormones, and stress responses in charophytes
Gravitropism
Gravitropism in plant cells entails coordinated interactions of heavy subcellular components, that is, statoliths, the cytoskeletal, and membrane trafficking (e.g. exocytosis). The tip-growing rhizoids and protonemata of Charophyceaen taxa have been exceptionally important specimens in elucidating gravitropism at the subcellular level in plants (Hodick et al., 1998; Braun and Limbach, 2006; Hader and Lebert, 2001). Studies have included the use of such technologies as zero gravity exposure (Limbach et al., 2005), magnetophoresis (Scherp and Hasenstein, 2007), and experimental perturbation via optical tweezers (Leitz et al., 1995). In Chara, “positively” gravitropic or downward growing rhizoids anchor the alga into the substrate. The “negatively” gravitropic protonemata grow against gravity or upward in darkness to light (Braun, 2001). The signal transduction and response pathways are limited to the apical region of a single cell in both rhizoids and protonemata. Gravitropism requires the sedimentation of statoliths, that is, single-membrane-bound vacuoles containing BaSO4 crystals and protein/carbohydrate structures (Hodick et al., 1998). Actin is integral to the sensing of gravity and the polarized growth mechanism of the gravitropic cell. Actin-based activities are regulated by several actin-binding proteins which control actin polymerization, actin filament remodeling, and the transport of vesicles and organelles. Actin and associated proteins work in coordination with steep gradients of cytoplasmic free Ca2+ for the coordination of statolith positioning. They direct statoliths to sediment at gravisensitive regions of the plasma membrane that will subsequently stimulate gravitropic growth responses (Braun 2001; Braun and Limbach 2006). The actin cytoskeleton also is involved in the structural and functional organization of the Spitzenkörper, a tip-based aggregation of endoplasmic reticulum and vesicles that deliver cell-wall material toward to apical plasma membrane for secretion and is responsible for controlling cell expansion, shape, and direction of growth (Braun et al., 1999).
Phytohormones
One of the major “headlines” arising from recent genomic and transcriptomic investigations of charophytes has been the identification of phytohormones as well as their biosynthetic and signaling pathways. This has “fueled the fires” for deciphering the roles of hormones in the evolution of multicellularity and morphogenesis and their functions in the integration of the signaling pathways in response to environmental stimuli. In a large multispecies genome-wide analysis, Wang et al. (2015) posited that auxin, cytokinin, ethylene, and strigolactone signaling pathways originated in charophyte lineages, while abscisic acid, jasmonate, and salicylic acid signaling pathways arose in the last common ancestor of land plants. Gibberellin and brassinosteroid dynamics arose later in land plant evolution. Further detailed analyses of hormones and hormone signaling pathways in charophytes may be found in these recent publications (Mahboubi and Delaux, 2021; Hori et al., 2014; Mutte et al., 2018; Martin-Arevalillo et al., 2019; Blázquez et al., 2020; Jiao et al., 2020; Wang et al., 2020; Baez and Nemhauser, 2021). Auxin and ethylene are the most well-studied hormones in charophytes and several important details have been recently revealed.
Auxin (i.e. indole-3-acetic acid [IAA]) and auxin transporters are commonly found in algae including the charophytes (Skokan et al., 2019; Vosolsobě et al., 2020). In Chara, auxin has been shown to hyperpolarize the membrane potential of the internodal cells (Zhang et al., 2016). Polar auxin transport in Chara shares the pathway for long-distance transport of nutrients through both the internodal cells and the parenchymatous nodal complexes (Raven, 2013). A strong circadian influence on metabolic pathways producing IAA has also been demonstrated in this alga (Beilby et al., 2015; Beilby, 2016). PIN-driven auxin transport has also been described in Klebsormidium nitens. The application of exogenous IAA and inhibitors of auxin biosynthesis and polar auxin transport stopped cell division and cell elongation in this alga (Ohtaka et al., 2017). In Spirogyra, ethylene has been shown to function in cell elongation, the modulation of the cell wall matrix by expansins and xyloglucan endotransglucosylases/hydrolases, downregulation of chlorophyll biosynthesis and photosynthesis, and activation of abiotic stress responses (Ju et al., 2015; Van de Poel et al., 2016).
Stress responses
Charophytes living at the interface of aquatic or terrestrial habitats or in subaerial zones are confronted with multiple stresses including low water, desiccation, high salt levels, substantial changes in CO2 and O2 levels, increased reactive oxygen species (ROS), and high levels of UV radiation (Delwiche and Cooper, 2015; de Vries and Archibald, 2018; Fürst-Jansen et al., 2021; Hereburger et al., 2014). Substantial adaptive strategies to these stresses have evolved in charophytes and several taxa have been selected as models for stress studies. For example, Klebsormidium species, including those living in soil crusts, exhibit distinct adaptations in response to fluctuations in temperature, water levels, and high irradiance (Holzinger et al., 2011; Mikhailyuk et al., 2015; Steiner et al., 2020). When K. crenulatum is subjected to freezing, new cell wall layers form and act as reinforcements to counteract physical pressures of the frozen surrounding medium. Mitochondria aggregate, ER levels substantially increase, the Golgi stacks are altered and photosynthetic oxygen production modulates. Under desiccation conditions, the levels of callose increase dramatically in cell walls (Herburger and Holzinger, 2015). Callose appears to be associated with the formation of fragmented filaments (Herburger and Holzinger, 2015) that most likely enhance dispersal. Klebsormidium may also produce spores to cope with low temperature and high light stress (Blaas and Holzinger 2017; Miguez et al., 2020). When Klebsormidium or its relative, Interfilum, are exposed to enhanced short UV wavelengths, two mycosporine-like amino acids, called klebsormidin A and klebsormidin B are produced. These compounds (Hartmann et al., 2020) are low molecular weight polar amino acid derivatives that act as passive shielding biomolecules by dissipating the energy of the previously absorbed UV light as harmless heat. UV stress also causes major changes to the F-actin architecture of the cell.
Transcriptomic analysis of Klebsormidium under desiccation stress (Holzinger et al., 2014) revealed an upregulation of transcripts for photosynthesis, energy production, ROS, chaperones associated with late embryogenesis (LEA) and early response to desiccation, as well as enzymes used for biosynthesis of the raffinose family of oligosaccharides that are known to act as osmolytes. Downregulation was observed for transcripts of integrative cellular functions including DNA replication, cell division, cofactor biosynthesis, and amino acid biosynthesis. Under cold-stress, there is an enhancement of the expression of heat-shock proteins, LEA, cryoprotectant carbohydrates and modified lipids, ROS scavenging components, and substantial changes to the cytoskeleton (Rippin et al., 2019).
The multicellular Coleochaete exhibits major changes in morphology when grown in desiccation versus aquatic conditions (Graham et al., 2012) and produces sporopollenin and lignin-like compounds (Delwiche et al., 1989). When wounded or placed under anoxic conditions or salt stress, Chara and other Charophyceaen taxa exhibit notable cell wall remodeling, changes to exo- and endocytosis, and notable salt tolerance mechanisms. This includes expression of the cation transporter (HKT), a Na+/H+ antiport (NHX), a H+-ATPase (AHA), and a Na+-ATPase (ENA) (Beilby, 2015; Foissner and Wasteneys, 2014; Phipps et al., 2021a, 2021b).
Distinct molecular and cellular changes occur in zygnematophytes placed under abiotic stress. Heat stress in Spirogyra and Mougeotia results in elevated transcript levels for molecular chaperones, thylakoid components, amino acid metabolism, and changes to phosphorelay-based signal transduction, Ca2+ signatures, and plastid biology (de Vries et al., 2020). de Vries and Archibald (2018) posited that responses to plastid stressors were critical to the colonization of land by an algal ancestor. In other Zygnematophyceae (Rippin et al., 2017, 2019), transcriptomic changes demonstrate that culture age has a severe influence on desiccation and in natural mats, the sun exposed upper layers have substantially more transcripts that are regulated when compared to protected lower layers. In Micrasterias, osmotic or salt stress leads to PCD that is induced by increased H2O2 levels produced in the chloroplast (Darehshouri and Lütz-Meindl, 2010; Steiner et al., 2021). Another abiotic stress-induced response of some zygnematophyceae is the accumulation of phenolic compounds including purple pigments (Newsome and van Breeman, 2012; Aigner et al., 2013; Newsome et al., 2013; Herburger et al., 2016a, 2016b). These pigments are glycosylated and highly branched phenolic compounds that form complexes with iron (Fe3+). This response may exhibit the requirement for iron metabolism to be adapted to the cellular demands in order to maintain a sufficient redox system but to avoid cellular damage because of iron-induced oxidative stress. A more complete overview on the genetics and biochemistry of metabolites in charophytes is provided in Rieseberg et al. (2022).
When P. margaritaceum is exposed to osmotic stress, Hechtian strands initially form (Domozych et al., 2020a, 2020b). This is followed by the formation of wall inclusions, altered HG lattice structure in the cell wall, and increased autophagy. Cell-wall modifications have also been noted in other zygnematophytes in both vegetative cells and zygospores (Fitzek et al., 2019; Herburger et al., 2019; Permann et al., 2021).
Conclusion
Charophytes continue to capture the interest of plant researchers. Yet we are only in the infancy stage in elucidating the details of their biology. Some of the more pressing questions that need to be addressed about charophytes are provided in “Outstanding Questions.” To answer these and other questions, it will require a synthesis of data derived from both classical and novel technologies. For example, carbohydrate and protein chemistry will be needed to decipher the complex glycomes and proteomes of the ECM. Mining of recently sequenced genomes and transcriptomes will allow for the mapping of biosynthetic pathways of these various ECM components and signaling networks that govern their secretion. This information can then be incorporated into cell biology-based studies such as those applying targeted agents that disrupt specific components or processes of the endomembrane system (e.g. chemical biology) and high-resolution microscopy- and spectroscopy-based imaging. One of the most pressing future challenges though will be generating stable transformed cell lines of model charophytes expressing proteins associated with the ECM synthesis, the endomembrane system, and cytoskeletal network. Stable transformed lines have been difficult to obtain with charophytes but recent technological advances (Kawai et al., 2021) provide promise for success in the near future. This will provide possibilities for 4D imaging of live cells that can then be used to create endomembrane and cell atlases of model charophytes.
Previous analyses of the cell wall biochemistry of charophytes have revealed notable similarities with cell walls of land plants (Popper et al., 2011; Sørensen et al., 2010, 2011; Harholt et al., 2016). Most of these studies have focused on polysaccharides including cellulose, xyloglucans, other hemicelluloses and pectins (e.g. Domozych et al., 2014; Mikkelsen et al., 2014, 2021; Frankova and Fry, 2021; see Table 2). Recently though, AGP-like glycoproteins have been identified in all charophyte groups except the Mesostigmatophyceae (Sørensen et al., 2011). AGPs represent a heterogeneous class of highly glycosylated, hydroxyproline-containing proteins typically found in the cell wall or apoplastic spaces (Silva et al., 2020). AGPs may act as Ca2+-capacitors, pectin plasticizers during cell expansion (Lamport and Varnai 2013; Lamport et al., 2014, 2018) and as conduits for communication between the cytoplasm and the ECM (Youl et al., 1998; Silva et al., 2020). Some AGPs act as adhesive agents (Huang et al., 2016). In charophytes, AGPs also appear to function in adhesion-based mechanisms (Palacio-Lopez et al., 2019). For example, in many desmids, short stiff fibers line the external surface of wall pores or fine fibrils cover the entire cell wall surface. These label with AGP-targeted monoclonal antibodies (e.g. JIM13) and serve as adhesion centers (Domozych et al., 2007, 2009a, 2009b; Eder et al., 2008). Adhesion efficacy is a critical trait for charophytes and other algae in that it stabilizes a cell’s position in a habitat and enhances capillary uptake of water during periods of water stress. Coordination of adhesion with secretion of EPS in gliding phenomena also allows for positioning the cell for maximizing light absorption for photosynthesis and/or the establishment of communication networks with local microbiota (Domozych and Domozych, 2008).
AGPs have also been studied in the cell walls of Spirogyra praetensis (Pfeifer et al., 2021). Interestingly, while there are biochemical similarities with typical AGPs, the lack of terminal Ara but notable amounts of terminal and 1,3-linked rhamnose indicated that these Spirogyra components should now be classified as “rhamnogalactan-proteins.”
These recent studies of AGPs or AGP-like components in charophytes demonstrate their fundamental importance in ECM-based dynamics. Future research is needed to elucidate their biosynthesis, interactions with cell wall polymers and plasma membrane (e.g. do they have GPI-anchors?) and their adhesive properties. Likewise, do these glycoproteins also act as signaling components, that is communicating information from the external environment to the cell or cell wall?
Many zygnematophytes secrete a mucilage-like EPS beyond the wall and often in very large amounts (Domozych et al., 2005). This material has many functions including water retention/anti-desiccation, creating cell buoyancy, fueling gliding motility, enhancing sexual reproduction efficacy, serving as the aggregate “glue” of a substrate (e.g. soil) particles, serving as a radiation trap and very likely, providing a structural conduit for interactions with local microbiota (Boney, 1981, 1980; Gerrath, 1993; Domozych and Domozych, 2008; Bellinger et al., 2010). The EPS may also serve as a high-quality carbon source for the heterotroph communities (Giroldo et al., 2005). The EPS consists primarily of complex anionic polysaccharides along with small amounts of protein (Paulsen and Veira, 1994; Domozych et al., 2005; Kiemle et al., 2007). EPS polysaccharides are highly branched, may be sulfated and chemically modulate in cells exposed to high temperature or desiccation conditions (Jiao et al., 2020). EPS is processed in the Golgi body and carried by large vesicles to the cell surface for secretion (Domozych and Domozych, 1993; Domozych et al., 2007, 2020a, 2020b; ,Eder and Lütz-Meindl, 2008, ,2010,). EPS biosynthetic pathways and the signaling cascades that turn on its production are not yet known.
The importance of land plant–microbiota interactions has been well detailed but the symbiotic ‘toolkit” or interplay between charophytes and local microbiota are only beginning to be understood (Delaux, 2017; de Vries and Archibald, 2018; Graham et al., 2018; Nishiyama et al., 2018). Furthermore, the role of EPS in these interactions remains a mystery. Does EPS serve as a structural platform for communication networks with specific microbiota? Why is the EPS glycome so complex and what enzymes are needed by local heterotrophs to metabolize it?
In a study of Adirondack wetland biofilms (Domozych and Domozych, 2008), 23.7% of all algae and cyanobacteria were zygnematophytes. These algae were observed to be initial colonizers of new substrates and subsequently residents of biofilms. Studies of the EPS sheaths of zygnematophytes from a peat bog revealed distinct populations of diverse bacteria (Fisher and Wilcox 1996; Fisher et al., 1998). Likewise, metagenomic analysis of microbiomes associated with other charophytes like Coleochaete pulvinata and Chaetosphaeridium globosum revealed diverse bacterial and fungal taxa (Knack et al., 2015). Clearly, a huge gap in our understanding of charophyte biology is their interactions with surrounding microbes and the role of EPS. This is an “elephant in the room” mystery that will need to be addressed in future research.
With the emergence of sequenced genomes of charophytes, substantial insight into the roles of hormones, stress signaling/responses and cell wall dynamics in plant evolution and physiology has emerged.
The synthesis of molecular and cellular data with field ecology studies has yielded a “whole biology” view of several charophytes, their role in aquatic and subaerial habitats and putative mechanisms that may have been key for successful transition onto land.
The presence of cell wall polymers similar to those of land plants and other ECM components positions zygnematophytes as potentially valuable model organisms in elucidating secretory mechanisms and membrane trafficking in plants.
What are the molecular tool boxes found in charophytes that allow them to survive at the aquatic/terrestrial interface, adapt to abiotic stressors, and form communication platforms with the surrounding microbiome?
How do hormones and other growth regulators function in charophytes?
How do the membrane trafficking networks and the cytoskeleton coordinate during development and environmental stress responses?
What is the interplay between the furrowing mechanism and the phragmoplast cell plate in the ZCC taxa and how does this relate to the evolution of multicellularity?
How do cell wall polymers interact in the architectural design of the wall and modulate during development and in response to stress?
What are the glycomes and proteomes of the EPS and other secreted materials, their synthetic pathways, and their modulations in response to environmental stimuli?
D.S.D. and K.B. were responsible for the composition of this review.
The author responsible for distribution of materials integral to the findings presented in this article in accordance with the policy described in the Instructions for Authors (https://dbpia.nl.go.kr/plphys/pages/general-instructions) is David S. Domozych ([email protected]).
Acknowledgments
Special thanks for assistance provided in the writing of this article to Lily Sun, Catherine Domozych, and Melaina Gilbert of Skidmore College.
Funding
This work was supported by National Science Foundation (USA) grants 2129443 and 1517345 (D.S.D.).
Conflict of interest statement. None declared.