-
PDF
- Split View
-
Views
-
Cite
Cite
Yamei Li, Ruyu Li, Zhiguo Han, Haitang Wang, Sixian Zhou, Yongqing Li, Yumei Wang, Junsheng Qi, David W Ow, Recombinase-mediated gene stacking in cotton, Plant Physiology, Volume 188, Issue 4, April 2022, Pages 1852–1865, https://doi.org/10.1093/plphys/kiac005
- Share Icon Share
Abstract
Site-specific gene stacking could reduce the number of segregating loci and expedite the introgression of transgenes from experimental lines to field lines. Recombinase-mediated site-specific gene stacking provides a flexible and efficient solution, but this approach requires a recombinase recognition site in the genome. Here, we describe several cotton (Gossypium hirsutum cv. Coker 312) target lines suitable for Mycobacteriophage Bxb1 recombinase-mediated gene stacking. Obtained through the empirical screening of random insertion events, each of these target lines contains a single intact copy of the target construct with precise sequences of RS2, lox, and attP sites that is not inserted within or close to a known gene or near a centromere and shows good expression of the reporter gene gfp. Gene stacking was tested with insertion of different combinations of three candidate genes for resistance to verticillium wilt into three cotton target lines: CTS1, CTS3, and CTS4. Nine site-specific integration events were recovered from 95 independently transformed embryogenic calluses. Southern and DNA sequence analyses of regenerated plants confirmed precise site-specific integration, and resistance to verticillium wilt was observed for plant CTS1i3, which has a single precise copy of site-specifically integrated DNA. These cotton target lines can serve as foundation lines for recombinase-mediated gene stacking to facilitate precise DNA integration and introgression to field cultivars.
Introduction
Traditional breeding has been used to introgress transgenes into commercial cultivars, but crossing to a different cultivar can lead to heterozygosity in many genes for agronomic traits. A seed-producing line must be homozygous for not only the transgene, but also for all other elite traits associated with each field cultivar. In cotton (Gossypium hirsutism), a tetraploid that segregates like a diploid, if there is no linkage drag the probability for assorting the “n” number of independent linkage units into a homozygous breeding line is (1/4)n. This probability could easily become 1 in a million plants, as for instance by adding 3 transgenic loci to a lab cultivar that differs from elite cultivars in 7 other segregating traits. Breeders could only select for homozygosity of a few traits in every generation, so increasing the number of unlinked loci would necessitate more backcrosses that extend the time, labor, and cost for introducing new cultivars.
A single insertion of the transgenic cluster would segregate as a single locus, and one way to achieve this would be to redo transformation with a larger DNA construct incorporating new and previously introduced transgenes. But to redo many previously introduced transgenes could be challenging in having all of the genes appropriately expressed in a single integration event. Moreover, it would likely require de-regulation of not only the newly introduced transgenes, but also the previously introduced DNA since those would be part of a new integration event.
Direct transformation of elite cultivars can bypass the introgression process but most commercial cultivars are more difficult to transform, and would require developing efficient transformation protocols for many different elite lines, especially when numerous independent transformants are typically needed for field efficacy testing. Unlike the deregulation of a single integration event that is bred out to numerous field cultivars, the same DNA introduced into every commercial cultivar would be independent events that would trigger individual de-regulation.
In planta gene stacking could offer a solution. Zinc finger nuclease-induced site-specific recombination has inserted new DNA next to preexisting transgenes in maize (Zea mays; Ainley et al., 2013) or next to a host gene in soybean (Glycine max) (Bonawitz et al., 2019), while meganuclease-mediated targeting has inserted herbicide resistance genes into a transgenic locus in cotton (D’Halluin et al., 2013). We previously described an in planta gene stacking method based on the use of recombinases (Hou et al., 2014). A circular DNA molecule recombines into a genomic recombination target using the Mycobacteriophage Bxb1-att site-specific integration system, which consists of the Bxb1 integrase (recombinase) to catalyze, without other proteins or high-energy cofactors, the recombination between a 48-bp attachment phage (attP) and a 38 bp attachment bacteria (attB) site to generate attachment left (attL), and attachment right (attR) sites (Ghosh et al., 2003). Each integrating molecule brings in an additional recombination target (attB or attP) for the next round of integration, and the gene stacking process can continue by switching between uses of attP and attB genomic targets. Transgenic DNA not needed after Bxb1 integrase-mediated gene targeting can be removed subsequently by the Coliphage P1 Cre-lox recombination system, in which the Cre recombinase deletes DNA between directly oriented 34-bp lox sites.
For this gene stacking method to operate, a target plant line must have an attP or an attB site to permit the integration of an incoming molecule through Bxb1 integrase catalyzed site-specific recombination. Although site-specific nucleases could direct the insertion of target sites, the cotton genome sequence cannot currently predict suitable chromosome locations for transgene expression. Moreover, to ensure that commercial use of the target lines would not be restricted by site-specific nuclease patents, we chose instead to use Agrobacterium tumefaciens-mediated gene transfer. A collection of 152 random-insertion lines were screened for the precise insertion of a target construct with the following properties: (1) single intact copy; (2) not close to a centromere; (3) not inserted into repetitive DNA; (4) not inserted within or close to a known gene; (5) precise sequence of recombination sites RS2, lox, and attP near the right and left T-DNA borders; and (6) good expression of the reporter gene gfp.
Moreover, to test the utility of the target lines, we stacked different combinations of three candidate genes for resistance to Verticillium dahliae that causes the destructive disease verticillium wilt. One or more of these genes, V. dahliae-secreted Asp f2-like protein gene (VdAL), Gossypium barbadense cv. AXMN lipid transfer protein precursor gene (GbLTP) and G. barbadense cv. AXMN toxin-induced protein-11 gene (GbAt11), were site-specifically integrated into lines CTS1, CTS3, and CTS4, and site-specific integration events were recovered from 9.5% of the independently transformed embryogenic calluses. Plants were then regenerated from these calluses and site-specific integration events were confirmed by Southern analysis. Plant CTS1i3, which has a single precise copy of site-specifically integrated DNA, showed higher resistance to verticillium wilt.
Results and discussion
Target constructs
We used two different strategies to generate target sites in the cotton genome. As in the previous strategy (Hou et al., 2014) the target construct pZH83 contains the selectable marker npt for resistance to kanamycin and the green fluorescence protein gene gfp for monitoring gene expression (Figure 1A). This segment of DNA is flanked by a set of directly oriented lox sites from the Coliphage Cre-lox site-specific recombination system (Hoess et al., 1982) to permit subsequent excision of the npt-gfp DNA after finding integration events with stable gfp expression. Downstream of the lox-npt-gfp-lox fragment is an attP site from the Mycobacteriophage Bxb1-att site-specific recombination system (Ghosh et al., 2003), which serves as the target for recombination by a circular DNA containing an attB site from this recombination system. On the other side of attP is a third lox site situated in opposite orientation with respect to the lox sites that flank npt-gfp. This third lox site permits subsequent Cre-lox site-specific excision of unneeded DNA introduced by the incoming molecule. Flanking the entire DNA segment is a set of directly oriented 119-bp RS2 recombination sites from the Acetinetobacter plasmid CinH-RS2 site-specific deletion system (Kholodii, 2001). This DNA segment with the flanking RS2 sites was then placed between the T-DNA left (LB) and right (RB) borders of an Agrobacterium binary vector for plant transformation. The RS2 sites were incorporated into this construct to permit the optional excision of the RS2-flanked DNA by the CinH recombinase, although this study had not yet tested this optional feature.
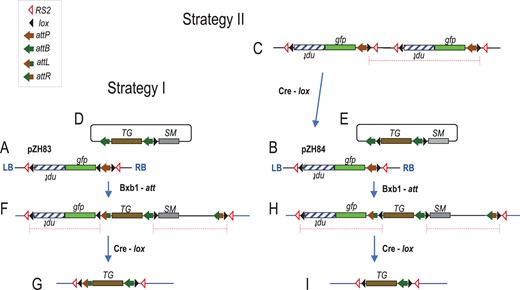
Gene stacking scheme in cotton. A, Schematic representation of strategy I target construct pZH83 with gfp and npt inserted into cotton chromosome from Agrobacterium-mediated random integration; D, Strategy I integration construct with SM (selection marker gene) and TG (trait gene) co-bombarded along with Bxb1 integrase-expressing construct pYQ78 (not shown, see Figure 3B); F, Expected structure from recombination with SM-distal attB and chromosomal attP; G, Structure after Cre recombinase-catalyzed deletion of DNA flanked by directly oriented lox sites. B, Strategy II target construct pZH84 has only two lox sites in opposite orientation. Co-transformation with a cre-expressing construct can delete tandem multicopy insertions, example shows only two copies (C), leading to a single copy structure in (B). E, Strategy II integration construct with an additional lox site distal to SM co-bombarded along with Bxb1 integrase-expressing construct pYQ78 to integrate into expected genome structure (H) from recombination with SM-distal attB and chromosomal attP, and (I) after Cre recombinase-catalyzed deletion of DNA flanked by directly oriented lox sites. Recombinase site symbols as shown in legend, Bxb1 recombinase-mediated attP × attB to yield attL and attR; LB and RB, T-DNA left and right borders, respectively. Promoters and terminators not shown, see “Materials and methods.” Gene transcription from left to right, except for npt indicated by upside down lettering.
We also used a second strategy in case Agrobacterium-mediated gene transfer did not yield a sufficient number of single-copy transformants. Based on prior experience in wheat (Triticum aestivum) and maize transformation, where Cre recombinase was used to convert multiple copy insertions into single-copy events (Srivastava et al., 1999; Srivastava and Ow, 2001), this second strategy used target construct pZH84 that lacks a lox site between gfp and attP (Figures 1, B and 2, A). Tandem multimers of pZH84 (Figure 1C) would have lox sites in the same orientation that could recombine to form a monomer (Figure 1B). Co-transformed along with a cre-expressing construct (pZH95, data not shown), the expectation was that Cre would resolve multiple tandem insertions into single-copy targets as long as the two outermost copies were in the same orientation (Figure 1C), and hence yield a higher rate of single-copy lines.
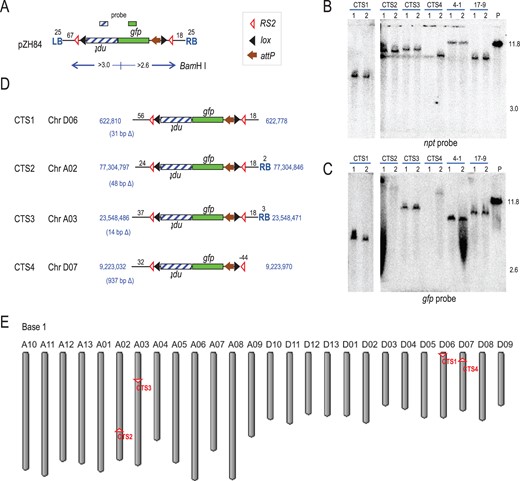
Genome location and structure of cotton target lines. A, pZH84 structure; D, pZH84 derived insertions. Blue lettering indicates chromosome positions at left and right ends and chromosome deletions in parentheses. Black lettering shows the number of base pairs from RS2, except for CTS4 that lacks a right end RS2. B and C, Southern blots detected a single T-DNA left or right end fragment in BamHI cleaved genomic DNA when probed with npt for a single band of expected size >3 kb (B), or with gfp for a single band >2.6 kb (C). Hybridization probes shown above pZH84. P: plasmid pZH84 added to Coker 312 genomic DNA at 1 copy per cotton genome. Fragments and size markers in kb. E, Cotton genome map from https://www.ncbi.nlm.nih.gov/genome/gdv/?org=gossypium-hirsutum&group=malvids; genome coordinates start at the top for each chromosome. Target sites derived from pZH84 shown as RS2 site, with site orientation indicated. Genes and symbols as in Figure 1. Two different T0 plants from the same callus labeled 1 and 2 above gels; 4-1 and 17-9 are other single-copy lines that did not meet the criteria for target lines.
Integration constructs
For plants with a single copy of the pZH83-derived T-DNA (Figure 1A), the integrating plasmid (Figure 1D) contains a selection marker gene (SM) and a trait gene (TG). Depending on the construct, the SM was either hygromycin resistance gene (hpt), bialaphos resistance gene (bar), or a gene fusion between bar and DsRed. A trait gene (or more than a single trait gene) is situated between a set of attB sites, and between SM and TG lies a single lox site. Bxb1 integrase, produced by co-introduced construct pYQ78 (Figures 3B, 4B, and 5B) would recombine the integrating plasmid into the genomic attP. Either attB sites on the integrating plasmid could recombine with chromosomal attP. The preferred structure is from recombination between the SM-distal attB with the genomic attP (Figure 1F). This configuration places directly oriented lox sites flanking the npt-gfp fragment as well the SM-plasmid backbone fragment. Cre recombinase subsequently introduced into this genome would be expected to generate the structure shown in Figure 1G, and comprises of the trait gene fragment surrounded by several recombination sites, but devoid of no-longer-needed selection markers and plasmid backbones.
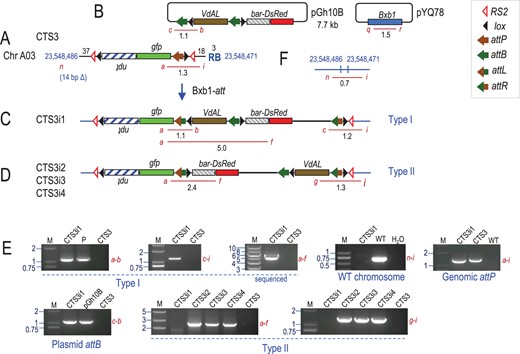
CTS3 derived site-specific integrant plants. A, Recombination between the genomic attP site in CTS3 with attB site in pGh10B (B) to produce (C) type I or (D) type II integration structure, catalyzed by Bxb1 integrase from co-transformed pYQ78. E, Representative PCR detection of site-specific recombination junctions, WT site, genome attP and plasmid attB. F, WT chromosome site corresponding CTS3 insertion. Red lines represent PCR products. M: marker lane, fragment sizes in kb; P: positive control from in vitro recombination of pZH84 and pGh10B.
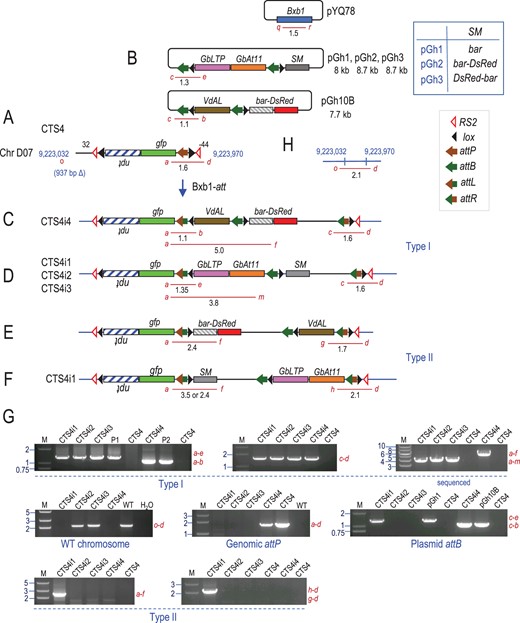
CTS4 derived site-specific integrant plants. A, Recombination between the genomic attP site in CTS4 with attB site in (B) pGh10B, pGh1, pGh2, or pGh3 to produce (C and D) type I or (E and F) type II integration catalyzed by Bxb1 integrase from co-transformed pYQ78. G, Representative PCR detection of site-specific recombination junctions, WT site, genome attP and plasmid attB. H, WT chromosome site corresponding to CTS4 insertion. Red lines represent PCR products. M: marker lane, fragment sizes in kb; P1 or P2 indicates positive control from in vitro recombination of pZH84 with pGh1 or pGh10B, respectively; WT: Coker 312 DNA.
For plants harboring target construct pZH84 (Figure 1B), the integrating construct (Figure 1E) differs from that shown in Figure 1D is having a second lox site in the opposite orientation distal to the SM. As before, Bxb1 integrase would recombine either attB site of the integration construct with the genomic attP, and the preferred structure (Figure 1H) is produced from recombination between the SM-distal attB with the genomic attP. This configuration also places directly-oriented lox sites flanking the npt-gfp fragment and the SM-plasmid backbone to permit subsequent Cre-lox mediated excision of DNA no longer needed (Figure 1I).
Generating target sites in the cotton genome
From the Agrobacterium-mediated transformation of cotton hypocotyls, plantlets regenerated on kanamycin-selection medium were screened for GFP activity. From the transformation with pZH83, 27 GFP-positive plants were regenerated from 10 kanamycin-resistant calluses, and with pZH84, 125 plants from 36 calluses (Table 1). These 152 plants were screened by polymerase chain reaction (PCR) for the presence of DNA adjacent to the T-DNA on the left and right borders (Supplemental Figure S1A). Out of 152 plants, 86 were PCR positive for having the left and right end DNA, and were derived from 4 pZH83-transformed and 31 pZH84 transformed calluses. Since plants regenerated from the same callus could be clonal, we therefore could be certain of only 4 pZH83-derived and 31 pZH84-derived independent events.
Steps achieved . | pZH83 . | pZH84 . |
---|---|---|
Number of Plants (Calluses/Independent Events) . | Number of Plants (Calluses/Independent Events) . | |
GFP expression detected | 27 (10) | 125 (36) |
Left and right ends detected by PCR | 9 (4) | 77 (31) |
Number of plants Southern hybridized to npt probe | 9 (4) | 55 (31) |
Single copy based on hybridization with npt probe | 0 | 33 (17) |
Single copy based on hybridization with gpf probe | 0 | 20 (12) |
Inverse PCR of independent events | a | 16 (10) |
Inserted into repetitive DNA | a | 3 |
Inserted into intron | a | 2 |
Too close to coding region stop codon | a | 1 |
Inserted into coding region (CTS4) | a | 1 |
Single copy target lines (CTS1, CTS2, and CTS3) | a | 3 |
Steps achieved . | pZH83 . | pZH84 . |
---|---|---|
Number of Plants (Calluses/Independent Events) . | Number of Plants (Calluses/Independent Events) . | |
GFP expression detected | 27 (10) | 125 (36) |
Left and right ends detected by PCR | 9 (4) | 77 (31) |
Number of plants Southern hybridized to npt probe | 9 (4) | 55 (31) |
Single copy based on hybridization with npt probe | 0 | 33 (17) |
Single copy based on hybridization with gpf probe | 0 | 20 (12) |
Inverse PCR of independent events | a | 16 (10) |
Inserted into repetitive DNA | a | 3 |
Inserted into intron | a | 2 |
Too close to coding region stop codon | a | 1 |
Inserted into coding region (CTS4) | a | 1 |
Single copy target lines (CTS1, CTS2, and CTS3) | a | 3 |
Not applicable.
Steps achieved . | pZH83 . | pZH84 . |
---|---|---|
Number of Plants (Calluses/Independent Events) . | Number of Plants (Calluses/Independent Events) . | |
GFP expression detected | 27 (10) | 125 (36) |
Left and right ends detected by PCR | 9 (4) | 77 (31) |
Number of plants Southern hybridized to npt probe | 9 (4) | 55 (31) |
Single copy based on hybridization with npt probe | 0 | 33 (17) |
Single copy based on hybridization with gpf probe | 0 | 20 (12) |
Inverse PCR of independent events | a | 16 (10) |
Inserted into repetitive DNA | a | 3 |
Inserted into intron | a | 2 |
Too close to coding region stop codon | a | 1 |
Inserted into coding region (CTS4) | a | 1 |
Single copy target lines (CTS1, CTS2, and CTS3) | a | 3 |
Steps achieved . | pZH83 . | pZH84 . |
---|---|---|
Number of Plants (Calluses/Independent Events) . | Number of Plants (Calluses/Independent Events) . | |
GFP expression detected | 27 (10) | 125 (36) |
Left and right ends detected by PCR | 9 (4) | 77 (31) |
Number of plants Southern hybridized to npt probe | 9 (4) | 55 (31) |
Single copy based on hybridization with npt probe | 0 | 33 (17) |
Single copy based on hybridization with gpf probe | 0 | 20 (12) |
Inverse PCR of independent events | a | 16 (10) |
Inserted into repetitive DNA | a | 3 |
Inserted into intron | a | 2 |
Too close to coding region stop codon | a | 1 |
Inserted into coding region (CTS4) | a | 1 |
Single copy target lines (CTS1, CTS2, and CTS3) | a | 3 |
Not applicable.
To detect copy number, plants were subjected to Southern blot analysis of BamHI treated DNA, which cuts the transgene construct once to split it into right-end and left-end fragments (Figure 2A). Using npt as a probe, the expectation would be a hybridizing band >3.0 kb. Of the 9 pZH83-derived plants from four events, none met this expectation (Table 1), but of the 55 pZH84-derived plants from 31 events, 33 of them from 17 events showed a single >3.0 kb hybridizing band (Figure 2B shows 12 representative plants from six events). A gfp probe would be expected to hybridize to a right-end band >2.6 kb; and 20 of the 33 plants, from 12 events, met this expectation (Figure 2C shows the same representative plants as Figure 2B). It is noteworthy that 39% of the pZH84-derived events (12/31) had the expected left and right end fragments for single-copy integration, consistent with prior research in wheat and maize that Cre-mediated resolution of multimeric insertions can generate a high rate of single-copy insertions (Srivastava et al., 1999; Srivastava and Ow, 2001).
Inverse-PCR was used to retrieve the flanking DNA to define the site location as well as the DNA sequence between the RS2 sites and the genome borders (Supplemental Figure S1B). Inverse-PCR products were amplified successfully from 16 plants that represented 10 of the 12 single copy events. A BLAST search against the cotton genome database using sequences flanking the insertion showed that seven insertion events were not suitable target lines because one was in a coding region, two were into introns, one after a TAG stop codon, and three were into repetitive DNA (Table 1).
This left only three insertion events (CTS1, CTS2, and CTS3) that met the criteria of being single intact copy, not close to a centromere or a coding region, not within repetitive DNA, has precise sequences for RS2, lox, and attP on the ends, and good expression of the reporter gene gfp. However, we noticed that CTS4, which had the T-DNA inserted into a coding region showed early boll set, with nearly double the number of bolls by 5 months compared to the wild-type (WT) control (Supplemental Figure S2, A and B). However, by 6.5 months, both WT and CTS4 boll yields were comparable (Supplemental Figure S2C). Since the ability to set bolls within a shorter time cycle might be beneficial for early harvesting, we kept this potentially useful line as a possible target line.
Map locations
The left end of pZH84 in Figure 2A depicts a 25-bp T-DNA left border along with 67 bp of spacer sequence between T-DNA LB and RS2, and at its right end 18-bp between RS2 and the 25 bp T-DNA RB. Figure 2D depicts the locations of the four lines based on matching the LB and RB flanking DNA with the cotton genome database (https://www.ncbi.nlm.nih.gov/genome/gdv/?org=gossypium-hirsutum). Descriptions at the DNA sequence level are provided in Supplemental Figures S3 and S4. Salient features of each target site are listed below. All insertions are described from T-DNA LB to RB as drawn in Figure 2A.
Target site CTS1: chromosome D06 between position 622,778 and 622,810 (Figure 2, D and E). The DNA between 622,779 and 622,809 is missing, representing a 31-bp deletion at the site of the insertion (Supplemental Figure S3). On the left end, it lacks the entire 25-bp LB plus 11 bp of the adjacent sequence. On the right end, it lacks the entire 25-bp RB (Supplemental Figure S4). The nearest coding region from the LB or RB is 1.3 kb (stop codon) or 11.2 kb (start codon), respectively.
Target site CTS2: chromosome A02 between position 77,304,797 and 77,304,846 (Figure 2, D and E). The DNA between 77,304,798 and 77,304,845 is not found, representing a 48-bp deletion at the insertion site (Supplemental Figure S3). On the left end, it lacks the entire 25-bp T-DNA LB, plus 43 bp of the adjacent vector sequence. On the right end, it lacks 23 bp of the T-DNA RB (Supplemental Figure S4). The nearest coding region from the LB or RB is 1.9 kb (start codon) or 119 kb (stop codon), respectively.
Target site CTS3: chromosome A03 between position 23,548,471 and 23,548,486 (Figure 2, D and E). Line CTS3 has a 14-bp deletion at the site of the insertion as the DNA between 23,548,472 and 23,548,485 is not found (Supplemental Figure S3). On the left end, it lacks the entire 25-bp T-DNA LB plus 30 bp of adjacent vector sequence. On the right end, it lacks 22 bp of the T-DNA RB (Supplemental Figure S4). The nearest coding region from the LB or RB is 1.4 kb (stop codon) or 39.8 kb (start codon), respectively.
Target site CTS4: chromosome D07 between 9,223,032 and 9,223,970 (Figure 2, D and E). The DNA between 9,223,033 and 9,223,969 is missing, representing a 937-bp deletion at the insertion site (Supplemental Figure S3). The deletion includes the loss of 769 bp at the 3′-end of the coding sequence of GhAMT1.3 (NCBI Accession number: XM_016889412), an ammonium transporter 1 member 3-like gene, and adjacent 168-bp genome sequence. The left end lacks the entire 25 bp T-DNA LB plus 35 bp of the adjacent vector sequence, while the right end lacks the entire 25 bp T-DNA RB plus 18 bp of adjacent vector sequence and 44 bp of RS2 (Supplemental Figure S4). The 44 bp deletion of RS2 would likely render CinH recombinase-mediated excision of DNA between the RS2 sites ineffective. As CTS4 is inserted into GhAMT1.3, the nearest start codon from the LB is that of GhAMT1.3 at 0.6 kb. The nearest coding region beyond GhAMT1.3 from the LB or RB is 28.3 kb (start codon) or 1.2 kb (stop codon), respectively.
Bxb1-mediated site-specific integration into the target lines
To test Bxb1-mediated site-specific integration into the target lines, embryogenic calluses were induced from T1 hypocotyls of CTS1, CTS3, and CTS4 that could be homozygous or hemizygous for the target site. CTS2 was not tested since it did not set seed. Instead of using reporter genes to exemplify trait genes, as was done to test site-specific integration in rice (Oryza sativa) and soybean (Li et al., submitted for publication; Jiang et al., in press), genes that encode resistance to a major disease were used in this study. Hence, integrating plasmids used were pGh10B (Figure 3B), pGh1, pGh2, pGh3 (Figure 4B), and pGh6 (Figure 5B) that range from 7.7 to 11 kb. They harbor different combinations of VdAL, GbLTP, and GbAt11 that putatively confer resistance to V. dahliae, the causative agent of verticillium wilt (Wang et al., 2016). Each integrating construct was co-bombarded into target line embryogenic calluses along with pYQ78 that produced Bxb1 integrase (Figures 3, B, 4, B, and 5, B). Transformation events were detected from resistance conferred by bar or hpt, PCR detection of the selection genes, as well as DsRed expression for those constructs with DsRed fused to bar. The positive calluses were subsequently subjected to PCR detection for site-specific integration junctions expected from type I (Figures 3, C, 4, C,4, D, and 5, C) or type II (Figures 3, D, 4, E F, and 5, D) integration depending on which attB of the integrating plasmid recombined with the genomic attP.
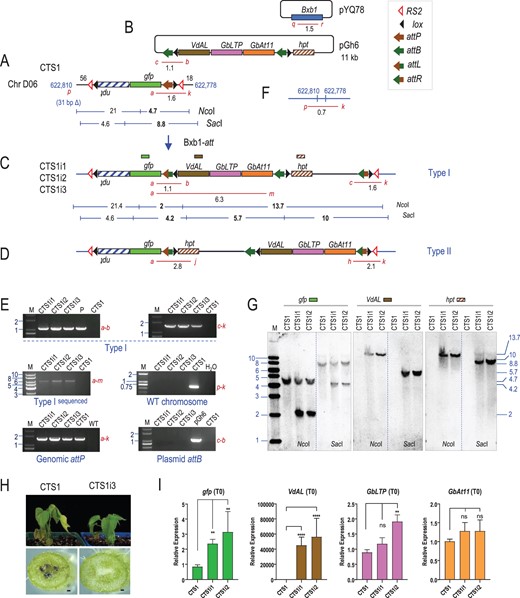
CTS1 derived site-specific integrant plants. A, Recombination between the genomic attP site in CTS1 with attB site in pGh6 (B) to produce (C) type I or (D) type II integration structure, catalyzed by Bxb1 integrase from co-transformed pYQ78. E, Representative PCR detection of site-specific recombination junctions, WT site, genome attP, and plasmid attB. F, WT chromosome site of corresponding CTS1 insertion. Red lines represent PCR products. G, Southern blots of plant CTS1 and integrant plants CTS1i1 and CTS1i2 cleaved with NcoI or SacI and hybridized with probe gfp, VdAL, or hpt. Restriction sites and expected fragments shown in (A) and (C). H, Integrated plant CTS1i3 showed resistance to Verticillium dahlia 16 d after inoculation with the “Vd991” conidial, scale bar: 0.01 mm. I, Expression of site-specifically integrated trait genes in CTS1i1 and CTS1i2 compared to CTS1. Transcript level of each gene normalized to G. hirsutum histone 3 gene. Data presented as means ± sd of three sample repeats. Significant differences according to unpaired t test (∗∗P < 0.01, ∗∗∗∗P < 0.0001, ns, not significant). M: marker lane, fragment sizes in kb; P: positive control from pZH84 and pGh6 in vitro recombination; WT: Coker 312 DNA.
From a total of 850 embryogenic calluses from three target lines bombarded with five different integration constructs, a total of 95 transformed calluses were obtained, an overall transformation efficiency of 11% (Table 2). For the 7.7 kb pGh10B (Figure 3B), bialaphos resistant calluses that expressed DsRed and confirmed as PCR-positive for bar were 18% (41/230) for CTS3 and 43% (13/30) for CTS4. However, based on PCR detection of integration junctions, only 4 of 41 CTS3-derived calluses and 1 of 13 CTS4-derived calluses were due to site-specific integration. Three were of the undesired type II integration detected by primers a + f and g + j (Figure 3, D and E), and only two were of the desired type I integration detected by primers a + b and c + i (Figure 3, C and E) or a + b and c + d (Figure 4, C and G). Hence, site-specific (versus random-only) integration efficiency for pGh10B was 9.3% (5/54), and the preferred type I integration frequency was 3.7% (2/54).
Target Line . | Integration Plasmid (size in kb) . | Number of Calluses Bombarded . | Calluses Positive for bar, bar+DsRed or hpt (Efficiency (%)) . | Number of PCR Positive Lines . | Recombination Junction Detected by PCR . | Site Specific Integration Efficiency . | ||||
---|---|---|---|---|---|---|---|---|---|---|
. | Type I . | Type II . | ||||||||
Primers used | a + b | c + i | a + f | g + i | ||||||
CTS3 | pGh10B (7.7) | 230 | 41 (18) | 1 | + | + | − | − | 9.8% (4/41) | |
3 | − | − | + | + | ||||||
Primers used | a + b | c + d | a + f | g + d | ||||||
CTS4 | pGh10B (7.7) | 30 | 13 (43) | 1 | + | + | − | − | 7.7% (1/13) | |
Primers used | a + e | c + d | a + f | h + d | ||||||
pGh1 (8) | 50 | 1 (2) | 1 | + | + | + | + | 100% (1/1) | ||
pGh2 (8.7) | 250 | 13 (5.2) | 1 | + | + | − | − | 7.7% (1/13) | ||
pGh3 (8.7) | 90 | 12 (13) | 1 | + | + | − | − | 8.3% (1/12) | ||
Primers used | a + b | c + k | a + j | h + k | ||||||
CTS1 | pGh6(11) | 200 | 15 (7.5) | 1 | + | + | − | − | 6.7% (1/15) | |
Total | 850 | 95 (11) | 9.5% (9/95) |
Target Line . | Integration Plasmid (size in kb) . | Number of Calluses Bombarded . | Calluses Positive for bar, bar+DsRed or hpt (Efficiency (%)) . | Number of PCR Positive Lines . | Recombination Junction Detected by PCR . | Site Specific Integration Efficiency . | ||||
---|---|---|---|---|---|---|---|---|---|---|
. | Type I . | Type II . | ||||||||
Primers used | a + b | c + i | a + f | g + i | ||||||
CTS3 | pGh10B (7.7) | 230 | 41 (18) | 1 | + | + | − | − | 9.8% (4/41) | |
3 | − | − | + | + | ||||||
Primers used | a + b | c + d | a + f | g + d | ||||||
CTS4 | pGh10B (7.7) | 30 | 13 (43) | 1 | + | + | − | − | 7.7% (1/13) | |
Primers used | a + e | c + d | a + f | h + d | ||||||
pGh1 (8) | 50 | 1 (2) | 1 | + | + | + | + | 100% (1/1) | ||
pGh2 (8.7) | 250 | 13 (5.2) | 1 | + | + | − | − | 7.7% (1/13) | ||
pGh3 (8.7) | 90 | 12 (13) | 1 | + | + | − | − | 8.3% (1/12) | ||
Primers used | a + b | c + k | a + j | h + k | ||||||
CTS1 | pGh6(11) | 200 | 15 (7.5) | 1 | + | + | − | − | 6.7% (1/15) | |
Total | 850 | 95 (11) | 9.5% (9/95) |
“+” or “−” indicates the presence and absence of the specific recombination junctions.
Target Line . | Integration Plasmid (size in kb) . | Number of Calluses Bombarded . | Calluses Positive for bar, bar+DsRed or hpt (Efficiency (%)) . | Number of PCR Positive Lines . | Recombination Junction Detected by PCR . | Site Specific Integration Efficiency . | ||||
---|---|---|---|---|---|---|---|---|---|---|
. | Type I . | Type II . | ||||||||
Primers used | a + b | c + i | a + f | g + i | ||||||
CTS3 | pGh10B (7.7) | 230 | 41 (18) | 1 | + | + | − | − | 9.8% (4/41) | |
3 | − | − | + | + | ||||||
Primers used | a + b | c + d | a + f | g + d | ||||||
CTS4 | pGh10B (7.7) | 30 | 13 (43) | 1 | + | + | − | − | 7.7% (1/13) | |
Primers used | a + e | c + d | a + f | h + d | ||||||
pGh1 (8) | 50 | 1 (2) | 1 | + | + | + | + | 100% (1/1) | ||
pGh2 (8.7) | 250 | 13 (5.2) | 1 | + | + | − | − | 7.7% (1/13) | ||
pGh3 (8.7) | 90 | 12 (13) | 1 | + | + | − | − | 8.3% (1/12) | ||
Primers used | a + b | c + k | a + j | h + k | ||||||
CTS1 | pGh6(11) | 200 | 15 (7.5) | 1 | + | + | − | − | 6.7% (1/15) | |
Total | 850 | 95 (11) | 9.5% (9/95) |
Target Line . | Integration Plasmid (size in kb) . | Number of Calluses Bombarded . | Calluses Positive for bar, bar+DsRed or hpt (Efficiency (%)) . | Number of PCR Positive Lines . | Recombination Junction Detected by PCR . | Site Specific Integration Efficiency . | ||||
---|---|---|---|---|---|---|---|---|---|---|
. | Type I . | Type II . | ||||||||
Primers used | a + b | c + i | a + f | g + i | ||||||
CTS3 | pGh10B (7.7) | 230 | 41 (18) | 1 | + | + | − | − | 9.8% (4/41) | |
3 | − | − | + | + | ||||||
Primers used | a + b | c + d | a + f | g + d | ||||||
CTS4 | pGh10B (7.7) | 30 | 13 (43) | 1 | + | + | − | − | 7.7% (1/13) | |
Primers used | a + e | c + d | a + f | h + d | ||||||
pGh1 (8) | 50 | 1 (2) | 1 | + | + | + | + | 100% (1/1) | ||
pGh2 (8.7) | 250 | 13 (5.2) | 1 | + | + | − | − | 7.7% (1/13) | ||
pGh3 (8.7) | 90 | 12 (13) | 1 | + | + | − | − | 8.3% (1/12) | ||
Primers used | a + b | c + k | a + j | h + k | ||||||
CTS1 | pGh6(11) | 200 | 15 (7.5) | 1 | + | + | − | − | 6.7% (1/15) | |
Total | 850 | 95 (11) | 9.5% (9/95) |
“+” or “−” indicates the presence and absence of the specific recombination junctions.
For pGh1, pGh2, and pGh3 into target line CTS4 (Figure 4, A and B), only a single callus from each integrating plasmid yielded site-specific integration detected by primers a + e and c + d (Figure 4, D and G), a total of 3 out of 26 or 11.5% (Table 2). For pGh2 and pGh3, the integration was type I, while for pGh1, both integration types were found from the one callus as primers a + f and h + d also detected type II junctions (Figure 4, F and G). Since the calluses from T1 plants might be homozygous for the target construct, finding both integration types could indicate integration into homologous chromosomes within the same genome. Alternatively, it could be due to integration of different cells in the same callus. For the 11-kb pGh6 containing 3 trait genes, PCR primers a + b and c + k (Figure 5, C and E) detected one type I event from 15 transformed CTS1 calluses (Table 2). Overall, site-specific integration frequency among transgenic calluses was 9.5% (9/95), while the preferred type I integration was 6.3% (6/95). However, these site-specific integration efficiencies could also include calluses with possible additional molecules integrated elsewhere in the genome.
Eight plants were regenerated from the calluses that showed type I integration and confirmed by detection of the integration junctions (Table 3), although they represented only six independent events, since three plants were from the same CTS1 callus. Integrant plants with the type I events were further tested by primers n + i, o + d, or p + k (Figures 3, F, 4, H, and 5, F) to check for DNA corresponding to the WT chromosome without a target construct. A PCR product would be too long to amplify across the 5.5-kb target construct. Since the WT locus-specific PCR product was detected from CTS4i2 and CTS4i3 with primers o + d (Figure 4, G and H), these two plants were hemizygous for the target locus (Table 3). For the others that are homozygous for the target locus, they could be either homozygous or hemizygous for site-specific integration. This was tested using primers a + i, a + d, or a + k to detect an intact genomic attP (Figures 3A, 4A, and 5A). As an intact attP was found in CTS3i1, CTS4i4, CTS1i1, CTS1i2, and CTS1i3, this means that only one of two target sites was occupied by an integrated plasmid. In plant CTS4i1, the plant PCR data confirmed the callus data of both types of integration patterns; and an intact attP was not found. Since this data was from a plant, it most likely means a type I event on one chromosome and type II event in the other homologous chromosome.
Plant Name . | Integration Plasmid . | Trait Gene(s) . | Marker Gene . | Type of Integration . | WT Locus . | attP (Empty Target) . | Genotype . | attB (Random Insertion) . | Bxb1 Integrase Gene . | Status of T0 Plants . |
---|---|---|---|---|---|---|---|---|---|---|
Primers n + i | Primers a + i | Primers c + b | Primers q + r | |||||||
CTS3i1 | pGh10B | VdAL | bar-DsRed | I | – | + | Type I/attP | + | + | Plantlet |
Primers o + d | Primers a + d | Primers c + e | Primers q + r | |||||||
CTS4i4 | pGh10B | VdAL | bar-DsRed | I | − | + | Type I/attP | + | + | Plantlet |
CTS4i1 | pGh1 | GbLTP, GbAt11 | bar | I + II | − | − | Type I/type II | + | + | Abnormal |
CTS4i2 | pGh2 | bar-DsRed | I | + | − | Type I/WT | − | − | No seed | |
CTS4i3 | pGh3 | DsRed-bar | I | + | − | Type I/WT | − | − | Abnormal | |
pGh6 | VdAL, GbLTP, GbAt11 | hpt | Primers p + k | Primers a + k | Primers c + b | Primers q + r | ||||
CTS1i1 | I | − | + | Type I/attP | − | − | Set bolls | |||
CTS1i2 | I | − | + | Type I/attP | − | − | Set bolls | |||
CTS1i3 | I | − | + | Type I/attP | − | − | Sacrificed for testing against verticillium wilt |
Plant Name . | Integration Plasmid . | Trait Gene(s) . | Marker Gene . | Type of Integration . | WT Locus . | attP (Empty Target) . | Genotype . | attB (Random Insertion) . | Bxb1 Integrase Gene . | Status of T0 Plants . |
---|---|---|---|---|---|---|---|---|---|---|
Primers n + i | Primers a + i | Primers c + b | Primers q + r | |||||||
CTS3i1 | pGh10B | VdAL | bar-DsRed | I | – | + | Type I/attP | + | + | Plantlet |
Primers o + d | Primers a + d | Primers c + e | Primers q + r | |||||||
CTS4i4 | pGh10B | VdAL | bar-DsRed | I | − | + | Type I/attP | + | + | Plantlet |
CTS4i1 | pGh1 | GbLTP, GbAt11 | bar | I + II | − | − | Type I/type II | + | + | Abnormal |
CTS4i2 | pGh2 | bar-DsRed | I | + | − | Type I/WT | − | − | No seed | |
CTS4i3 | pGh3 | DsRed-bar | I | + | − | Type I/WT | − | − | Abnormal | |
pGh6 | VdAL, GbLTP, GbAt11 | hpt | Primers p + k | Primers a + k | Primers c + b | Primers q + r | ||||
CTS1i1 | I | − | + | Type I/attP | − | − | Set bolls | |||
CTS1i2 | I | − | + | Type I/attP | − | − | Set bolls | |||
CTS1i3 | I | − | + | Type I/attP | − | − | Sacrificed for testing against verticillium wilt |
“+” or “−” indicates the presence and absence of the specific recombination junctions.
Plant Name . | Integration Plasmid . | Trait Gene(s) . | Marker Gene . | Type of Integration . | WT Locus . | attP (Empty Target) . | Genotype . | attB (Random Insertion) . | Bxb1 Integrase Gene . | Status of T0 Plants . |
---|---|---|---|---|---|---|---|---|---|---|
Primers n + i | Primers a + i | Primers c + b | Primers q + r | |||||||
CTS3i1 | pGh10B | VdAL | bar-DsRed | I | – | + | Type I/attP | + | + | Plantlet |
Primers o + d | Primers a + d | Primers c + e | Primers q + r | |||||||
CTS4i4 | pGh10B | VdAL | bar-DsRed | I | − | + | Type I/attP | + | + | Plantlet |
CTS4i1 | pGh1 | GbLTP, GbAt11 | bar | I + II | − | − | Type I/type II | + | + | Abnormal |
CTS4i2 | pGh2 | bar-DsRed | I | + | − | Type I/WT | − | − | No seed | |
CTS4i3 | pGh3 | DsRed-bar | I | + | − | Type I/WT | − | − | Abnormal | |
pGh6 | VdAL, GbLTP, GbAt11 | hpt | Primers p + k | Primers a + k | Primers c + b | Primers q + r | ||||
CTS1i1 | I | − | + | Type I/attP | − | − | Set bolls | |||
CTS1i2 | I | − | + | Type I/attP | − | − | Set bolls | |||
CTS1i3 | I | − | + | Type I/attP | − | − | Sacrificed for testing against verticillium wilt |
Plant Name . | Integration Plasmid . | Trait Gene(s) . | Marker Gene . | Type of Integration . | WT Locus . | attP (Empty Target) . | Genotype . | attB (Random Insertion) . | Bxb1 Integrase Gene . | Status of T0 Plants . |
---|---|---|---|---|---|---|---|---|---|---|
Primers n + i | Primers a + i | Primers c + b | Primers q + r | |||||||
CTS3i1 | pGh10B | VdAL | bar-DsRed | I | – | + | Type I/attP | + | + | Plantlet |
Primers o + d | Primers a + d | Primers c + e | Primers q + r | |||||||
CTS4i4 | pGh10B | VdAL | bar-DsRed | I | − | + | Type I/attP | + | + | Plantlet |
CTS4i1 | pGh1 | GbLTP, GbAt11 | bar | I + II | − | − | Type I/type II | + | + | Abnormal |
CTS4i2 | pGh2 | bar-DsRed | I | + | − | Type I/WT | − | − | No seed | |
CTS4i3 | pGh3 | DsRed-bar | I | + | − | Type I/WT | − | − | Abnormal | |
pGh6 | VdAL, GbLTP, GbAt11 | hpt | Primers p + k | Primers a + k | Primers c + b | Primers q + r | ||||
CTS1i1 | I | − | + | Type I/attP | − | − | Set bolls | |||
CTS1i2 | I | − | + | Type I/attP | − | − | Set bolls | |||
CTS1i3 | I | − | + | Type I/attP | − | − | Sacrificed for testing against verticillium wilt |
“+” or “−” indicates the presence and absence of the specific recombination junctions.
Additionally, primers c + b or c + e (Figures 3, B, 4, B, and 5, B) were used to detect an intact attB site of the integrating plasmid, with the assumption that its detection would indicate another copy of the construct randomly inserted in the genome. Finally, to see whether there was integration by the Bxb1 integrase construct pYQ78, primers q + r (Figures 3, B, 4, B, and 5, B) were used to detect the integrase gene (Supplemental Figure S5). As shown in Table 3, CTS3i1, CTS4i4, and CS4i1 contain random integrations of pYQ78 as well as pGh10B or pGh1. For CTS4i2 and CTS4i3, neither genome contained a copy pYQ78 or another copy of pGh2 or pGh3. For the pGh6 integration, the three plants regenerated from the same callus with identical PCR data would likely be clonal siblings. Each showed a type I integration pattern and without additional copies of pGh6 (Figure 5E) or pYQ78 (Supplemental Figure S5). In total, half of the six independent events also have random integration of pYQ78 and the integrating plasmid, although these unwanted transgenes might segregate away in future generations.
Representative DNA segments were PCR amplified and sequenced. Primers a + f amplified a 5.0-kb fragment from CTS3i1 and CTS4i4 (Figures 3, C and 4, C) covering attL, lox, attB, and VdAL. Primers a + m amplified a 3.8-kb fragment from CTS4i1, CTS4i2, and CTS4i3 containing attL, lox, GbLTP, and GbAt11 (Figure 4D), and a 6.3-kb fragment from CTS1i1, CTS1i2, and CTS1i3 covering attL, lox, VdAL, GbLTP, and GbAt11 (Figure 5C). In all cases, the DNA sequences were as predicted without a single mutation found (Supplemental Figures S6–S9).
Structural fidelity of representative plants was further analyzed by Southern hybridization. With NcoI-cleaved genomic DNA, a gfp probe is expected to hybridize to a 4.7 kb size band in CTS1 and a 2 kb band in CTS1i1 and CTS1i2 (Figure 5, A and C), while a VdAL or a hpt probe is expected to hybridize to a 13.7-kb band in CTS1i1 and CTS1i2 (Figure 5C). With SacI-treated DNA, a gfp probe is expected to hybridize to an 8.8-kb band in CTS1 and a 4.2-kb band in CTS1i1 and CTS1i2, a VdAL probe is expected to hybridize to a 5.7-kb band in CTS1i1 and CTS1i2, and the hpt probe is expected to hybridize to a 10-kb band in CTS1i1 and CTS1i2 (Figure 5, A and C). As shown in Figure 5G, each expectation was met. Along with the sequence data, this shows that Bxb1 integrase-mediated site-specific integration into the cotton is a precise process.
Expression of trait genes
CTS1i1, CTS1i2, or CTS1i3 each contains three genes for resistance to verticillium wilt, a destructive disease that reduces yield and fiber quality in cotton-growing areas worldwide (Wang et al., 2016). VdAL from the V. dahliae strain Vd991 encodes a secreted Aspf2-like protein that can confer resistance to verticillium wilt in Arabidopsis thaliana and in cotton (Ma et al., 2021). GbLTP from G. barbadense cultivar AXMN encodes a putative lipid transfer protein precursor predicated in NCBI to have anti-fungal activity; and disruption of Arabidopsis glycosylphosphatidylinositol-anchored LTP (LTPG1) enhanced susceptibility to infection by the necrotrophic fungal pathogen Alternaria brassicicola (Lee et al., 2009). GbAt11 encodes toxin-induced protein-11 from G. barbadense cultivar AXMN that is specifically induced by V. dahliae. Overexpression of GbAt11 enhanced verticillium wilt resistance and increased the number of bolls per plant in G.hirsutum (Qiu et al., 2020).
Since all three plants regenerated from the same callus, they could be clonal derivatives. To check the effect of the integrated genes, we sacrificed the CTS1i3 T0 plant for a test against the pathogen V. dahliae. Resistance was observed 16 d after inoculation with the Vd991 conidial suspensions via the root dipping method (Figure 5H). While CTS1i3 showed healthy growth, the leaf of the control (CTS1 target line) plant had wilted. In addition, the vascular tissue of the CTS1 control was severely blackened indicative of infection. Unfortunately, despite showing higher resistance to V. dahliae, CTS1i3 succumbed to the inoculation and failed to survive after 30 d, 12 d longer than the CTS1 control that died after 18 d.
For CTS1i1 and CTS1i2, RT-qPCR was performed on leaf tissue of 4-month-old T0 plants. Controlled by the commelina yellow mottle virus promoter (CoYMVpro), VdAL mRNA was not detected from CTS1 as expected, but showed expression in CTS1i1 and CTS1i2 (Figure 5I). Compared with CTS1, GbLTP controlled by the nopaline synthase promoter (nospro) showed about a two-fold higher expression in CTS1i2, but not in CTS1i1. It is possible that somaclonal variation might have caused this difference between the two regenerated plants. With GbAt11, controlled by the mannopine synthase promoter derivative Superpro, expression over CTS1 was not statistically significant. For the latter two genes, the PCR assays were detecting both the G. barbadense as well as the G. hirsutum GbLTP and GbAt11 genes, and we do not have an explanation why total expression was not raised substantially higher by the additional G. barbadense genes in CTS1i1 and CTS1i2. It remains to be tested whether this pattern of gene expression would remain after Cre-mediated excision of the transgenic DNA that is no longer needed. Interestingly, integration of the three trait genes next to gfp also increased gfp expression in CTS1i1 and CTS1i2 by two- to three-fold, as though the newly inserted DNA might have brought in a cis-acting enhancer element. This enhancement effect on preexisting transgenes was also found with gene integration studies in rice (Li, R. et al., submitted for publication).
Future prospects
Despite the precision of the recombinase technology, obtaining plants from site-specific integration still requires tissue culture-induced regeneration that can be difficult in cotton. CTS4i1 and CTS4i3 survived as plantlets long enough for molecular analysis, but eventually died as they appeared abnormal and made few leaves. CTS4i2 survived but has yet to set seed. On the other hand, CTS3i1, CTS4i4, CTS1i1, and CTS1i2 have set bolls but the seeds were very delicate and have yet to bud. We are currently attempting to rescue these plants through grafting and outcrosses.
Problems in maintaining regenerated plants could be caused by a combination of suboptimal tissue culture care and tissue culture-induced somaclonal variation, both of which are likely related to the length of time in culture, as it took 6–8 months to obtain the site-specific integrant plantlets after particle bombardment; and we admit that this team was previously not experienced with cotton tissue culture. Although hygromycin and bialaphos were effective for hpt and bar, respectively, the selection was longer and less effective compared with using kanamycin for npt. The auxiliary use of DsRed greatly aided the selection since transformation events could be visualized earlier. Although we shall continue to test various means to obtain seed from CTS2, it remains possible that CTS2 will not serve as a viable target line, leaving only CTS1, CTS3 as true target lines, along with CTS4 as a target line with the insertion within a coding region of GhAMT 1.3, an ammonium transporter 1 member 3-like gene (Sun et al., 2019).
As our main focus was to developing target lines, the genes for resistance to verticillium wilt just happened to serve as example trait genes. The in vitro stacking of three trait genes in pGh6 was also a test to see whether we could obtain precise integration of a larger 11-kb molecule. Although 15 transformed calluses were recovered, only one was due to precise site-specific integration, but a resistance phenotype was observed in the regenerated plant, which lends to future attempts to scale up a repeat of this integration of pGh6 into CST1 for the goal of generating verticillium wilt plants
Materials and methods
DNA constructs
Target constructs and integration constructs (Supplemental Figures S10 and S11) as diagramed in the experiment were made using standard recombinant DNA methods. All PCR reactions were conducted using KOD FX High success-rate DNA polymerase (TOYOBO, Japan). For target constructs: neomycin phosphotransferase II (npt) gene was controlled by the cotton (Gossypium hirsutum) ubiquitin promoter and the cauliflower mosaic virus (CaMV) 35S RNA terminator. The green fluorescent protein (gfp) gene (enhanced version) was controlled by the sugarcane bacilliform virus promoter and octopine synthase (ocs) terminator. The cre gene was controlled by the CaMV 35S promoter and the nopaline synthase (nos) terminator. For integration constructs: marker gene bar, bar-DsRed, or DsRed-bar were all controlled by CaMV 35S RNA promoter and terminator. GbLTP (NCBI Accession number: AY507141.1) was controlled by the nos promoter and terminator. GbAt11 was controlled by the mannopine synthase promoter derivative Superpro (Lee et al., 2007) and ocs terminator. VdAL was controlled by the CoYMVpro and the rice ubiquitin (OsUbi) terminator. Hygromycin phosphotransferase gene (hpt) was controlled by the CaMV 35S RNA promoter and terminator. Bxb1 recombinase gene was controlled by the CaMV 35S RNA promoter and nos terminator.
Agrobacterium-mediated transformation
A total of 1,471 segments (∼1.5 cm) of hypocotyl of G. hirsutum (cv. Coker 312) were used for transformation. Infection was conducted with A. tumefaciens EHA105 harboring vector pZH83 or co-transformation of Agrobacterium EHA105 with pZH84 and Agrobacterium EHA105 with the cre-expressing construct pZH95. Transformation procedure was as described (Wu et al., 2008).
PCR and RT-qPCR
Genomic DNA of plants or calluses was extracted with Plant Genome Extraction Kit (Magen, Guangzhou, China). DNA quality was checked by gel electrophoresis. The PCR was conducted under standard conditions using 2x Mix (GenStar, Shanghai, China) using the primers listed in Supplemental Table S1. Total RNA of the second leaf from the top of the cotton plant was extracted by HiPure Plant RNA Mini Kit (Magen, China). RNA was reverse-transcribed using PrimeScript RT Reagent Kit with gDNA Eraser (TakaRa, Shiga, Japan). RT-qPCR was performed following manufacturer’s protocols for the SYBR Premix Ex Taq (TakaRa, Japan) on an ABI 7900 Real-Time PCR System (Applied Biosystems, Waltham, MA, USA). The Ghhistone3 gene (Accession number in Gene bank: Gh_A02G1234) was used as an internal control. PCR conditions were one cycle of 95°C for 30 s, 40 cycles of 95°C for 5 s, and 60°C for 30 s. The dissociation curves for each reaction were analyzed, and the 2−ΔΔCT method was used to calculate the relative expression levels of each target gene (Pathak and Srivastava, 2020). Primers listed in Supplemental Table S1, and values are means ± sd of three sample repeats. Significant differences were analyzed by unpaired t test.
Target site identification and mapping
Inverse-PCR was conducted as described (Boulin and Bessereau, 2007) on DNA from single-copy transgenic lines. BamHI was used to cleave DNA from single-copy lines, followed by circularization with T4 ligase (TaKaRa, Japan), and then two rounds of PCR with primers listed in Supplemental Table S1. PCR products were purified from 1% w/v agarose gel prior to DNA sequencing. Using flanking DNA sequence, bioinformatics analysis was performed using the database: https://www.ncbi.nlm.nih.gov/genome/gdv/?org=gossypium-hirsutum&group=malvids). Inverse-PCR product sequences were blasted against the cotton genome database from NCBI. Vector sequences from Inverse-PCR products were aligned with the plasmid sequences using online software ClustalW2 (http://www.ebi.ac.uk/Tools/msa/clustalw2/). Transgene insertions were mapped to the cotton chromosome by online software genome data viewer (https://www.ncbi.nlm.nih.gov/genome/gdv/?org=gossypium-hirsutum&group=malvids).
Yield evaluation
CTS4 T1 seeds were germinated in the mix of Jiffy substrate (Netherlands), vermiculite and perlite in a ratio of 3:1:1. They were grown under light cycle of 16-h d/8-h night and light intensity of 100 μmol/m2 at 26–28°C. Zygosity was determined by PCR with primer set a + d and o + d (Supplemental Table S1). Cotton yield including number of bolls, lint yield, unginned cotton were evaluated at 5 and/or 6.5 months after planting and the data are presented in Supplemental Figure S2. Significant differences were analysis by unpaired t test (n = 4).
Southern blot analysis
Genomic DNA (30 μg) cleaved with BamHI was transferred to Hybond-N+ membrane (GE Healthcare, Chicago, IL, USA) by 10 × SSC using Model 785 Vacuum Blotter (Bio-Rad, Hercules, CA, USA). [α-32P] dCTP labeled npt or gfp fragments (Amersham Rediprime II Random Prime Labelling System, GE Healthcare, Buckinghamshire, UK) were used as hybridization probes. Hybridization and washing methods were as described (Sambrook and Russell, 2001). After washing, membranes were exposed to a phosphor screen for 5–12 h and scanned on Typhoon FLA 9500 (IP: 635nm, PMT: 500V, Pixel size 200 μm). For site-specific integrant plants, genomic DNA (40 μg) was cleaved with NcoI or SacI, respectively, and hybridized with hpt, gfp and VdAL probes using DIG High Prime DNA Labelling and Detection Starter Kit II (Roche, Basel, Switzerland). The highly sensitive probes were PCR labeled with DIG-dUTP (alkali-labile) using PCR DIG Probe Synthesis Kit. Primers for labeling in Supplemental Table S1.
GFP and DsRed activity
GFP or DsRed fluorescence of calluses or leaf was visualized using a DMI6000B microscope (Leica, Wetzlar, Germany). The wavelength for excitation filter for GFP ranged from 440 to 520 nm, and 510 LP was chosen for the barrier filter. For DsRed, wavelength for excitation filter ranged from 525 to 565 nm, and the wavelength of the barrier filter ranged from 572 to 648 nm.
Particle bombardment for site-specific integrant plants
Growth media and detailed components are listed in Supplemental Table S2, and have been derived from MS salts (Murashige and Skoog, 1962) and B5 vitamins (Gamborg et al., 1968). Calluses were induced from sections of hypocotyls of 8-d-old seedlings of target lines on medium CIM1 under light intensity of 60–70 μmol/m2/s and photoperiod of 16-h light/8-h dark at 26–28°C (Supplemental Figure S12, A and B). After 3 weeks, they were transferred into MSVc medium and cultured in the dark until embryogenic calluses formed (Supplemental Figure S12C). MSBIK medium was used to subculture calluses (Supplemental Figure S12D). Before bombardment, the embryogenic calluses were precultured 1 week and put in osmotic medium MSOs for 4 h (Supplemental Figure S12E) before co-bombardment with the integration construct and the Bxb1 integrase-expression construct coated with 1.0-µm gold particles in the presence of 2.5 M MgCl2 and 3-mg/mL protamine. A biolistic PDS-1000/He particle delivery system (Bio-Rad, Hercules, CA, USA) was used for bombardment at a distance of 6.0 cm from 1100-psi rupture disk. Thereafter, calluses were kept on osmotic medium for another 18-h before detection of DsRed transient expression (Supplemental Figure S12F). Selection was on FSH medium (for hpt selection) or FSB medium (for bar selection) with subculture every month. For a resistant callus that expressed DsRed (Supplemental Figure S12G), parts of it were subjected to PCR analysis to identify the site-specific integration junctions, other parts were plated on a sterile filter paper on top of F medium to initiate formation of individual somatic embryos (Supplemental Figure S12H) under light intensity 25–35 μmol/m2/s, photoperiod of 16-h light/8-h dark. Somatic embryos about 0.5–1 cm were transferred on F medium until plantlets developed for at least 1 month before hardening off the plantlets for transplantation to soil (Supplemental Figure S12I).
Pathogen inoculation for evaluation of verticillium wilt resistance
Verticillium dahliae strains (Vd991) were grown on Potato Dextrose Agar medium (Coolaber, Beijing, China) at 25°C until enough spores formed. The spore suspensions were prepared at 2 × 105 conidia per milliliter before inoculation. CTS1.3 and CTS1 were cultured in water for 7 d before dipping their roots into the spore suspension for 5 min. Thereafter, they were transferred to soil in a growth chamber under light intensity of ∼100 μmol/m2/s, photoperiod 16-h light/8-h dark at 26–28°C.
Accession numbers
GenBank accession numbers for pZH83, pZH84, and pZH95 are OL870854, OL870855, and OL870856, respectively.
Supplemental data
The following materials are available in the online version of this article.
Supplemental Figure S1. PCR detection of insertions ends and flanking host DNA.
Supplemental Figure S2. Yield evaluation of CTS4 T1 plants.
Supplemental Figure S3. Flanking DNA of the target line insertion sites.
Supplemental Figure S4. pZH84-derived target sites.
Supplemental Figure S5. PCR detection of Bxb1 gene in integrant plants.
Supplemental Figure S6. Fragment a-f sequence amplified from CTS3-derived integrated plant CTS3i1.
Supplemental Figure S7. Fragment a-f sequence amplified from CTS4-derived integrant plant CTS4i4.
Supplemental Figure S8. Fragment a-m sequences amplified from CTS4-derived integrant plants CTS4i1, CTS4i2, and CTS4i3.
Supplemental Figure S9. Fragment a-m sequences amplified from CTS1 derived integrant plants CTS1i1, CTS1i2, and CTS1i3.
Supplemental Figure S10. Maps of target construct pZH83, pZH84, and Cre expression construct pZH95.
Supplemental Figure S11. Maps of integration construct pGh10B, pGh1, pGh2, pGh3, and pGh6.
Supplemental Figure S12. Biolistic transformation of cotton.
Supplemental Table S1. PCR primers used in this study.
Supplemental Table S2. Growth media used in this study.
These authors contributed equally (Y.L. and R.L.).
D.W.O., R.L., and Y.L. designed the research; Y.L. performed the research; Z.H., H.W., S.Z., Y.L., and Y.W. contributed to target line construction, J.Q. provided trait genes VdAL, GbLTP, and GbAt11. D.W.O., Y.L., and R.L. wrote the manuscript.
The author responsible for distribution of materials integral to the findings presented in this article in accordance with the policy described in the Instructions for Authors (https://dbpia.nl.go.kr/plphys/pages/general-instructions) is: Ruyu Li ([email protected]).
Acknowledgments
We thank Shenjie Wu of Shanxi Agricultural University for Coker 312 seeds.
Funding
This study was supported by the National Key R&D Project (Grant Nos. 2016YFD0101904) from the Ministry of Science and Technology of China and the Key Research Program of Frontier Sciences CAS, China (Grant No QYZDY-SSW-SMC010).
Conflict of interest statement: All authors delcared no conflict of interest.