-
PDF
- Split View
-
Views
-
Cite
Cite
Takashi Nobusawa, Misaki Kamei, Hiroaki Ueda, Naoya Matsushima, Hiroshi Yamatani, Makoto Kusaba, Highly pleiotropic functions of CYP78As and AMP1 are regulated in non-cell-autonomous/organ-specific manners, Plant Physiology, Volume 186, Issue 1, May 2021, Pages 767–781, https://doi.org/10.1093/plphys/kiab067
- Share Icon Share
Abstract
The cytochrome P450 CYP78A5/KLUH in Arabidopsis thaliana is predicted to be involved in the synthesis of a mobile signal molecule that has a pleiotropic function that is distinct from classical phytohormones. CYP78A5 has five close relatives in Arabidopsis. We first investigated their functions, focusing on the plastochron, leaf size, and leaf senescence. Our analyses revealed that CYP78A5 and CYP78A7 are involved in the plastochron and leaf size, and CYP78A6 and CYP78A9 are involved in leaf senescence. Complementation analyses using heterologous promoters and expression analyses suggested that CYP78A isoforms have a common biochemical function and are functionally differentiated via organ-specific expression. The altered meristem program1 (amp1) carboxypeptidase mutant shows a phenotype very similar to that of the cyp78a5 mutant. Complementation analyses using boundary and organizing center-specific promoters suggested that both CYP78A5 and AMP1 act in a non-cell-autonomous manner. Analyses of multiple cyp78a mutants and crosses between cyp78a and amp1 mutants revealed that AMP1/LIKE AMP1 (LAMP1) and CYP78A isoforms regulate plastochron length and leaf senescence in the same genetic pathway, whereas leaf size is independently regulated. Furthermore, we detected feedback regulation between CYP78A6/CYP78A9 and AMP1 at the gene expression level. These observations raise the possibility that AMP1 and CYP78A isoforms are involved in the synthesis of the same mobile signal molecule, and suggest that AMP1 and CYP78A signaling pathways have a very close, albeit complex, functional relationship.
Introduction
Development and homeostasis in plants are regulated by a diverse range of signaling molecules, as represented by phytohormones. In addition to classical phytohormones, previously unidentified phytohormones and signal molecules such as strigolactone and sulfated peptides have recently been reported, thereby hinting at the probable existence of several unidentified signal molecules in plants (Kaufmann and Sauter, 2019; Bürger and Chory, 2020). For example, N-hydroxypipecolic acid was recently identified as an essential molecule for systemic acquired resistance (Hartmann and Zeier, 2018). Given that phytohormones have highly pleiotropic functions, mutants in their biosynthesis and signal transduction similarly show pleiotropic phenotypes, among which, mutants for the genes encoding enzymes are of particular interest, as these may be involved in the synthesis of signal molecules. One such gene is Arabidopsis thaliana KLUH, which encodes the endoplasmic reticulum (ER)-localized cytochrome P450 CYP78A5 (Zondlo and Irish, 1999; Anastasiou et al., 2007).
In plants, cytochrome P450 monooxygenases constitute a large enzyme family, whose members are involved in the biosynthesis of several signaling molecules (Bak et al., 2011). For example, CYP701A1 (ent-kaurene oxidase) and CYP88A3 (ent-kaurenoic acid oxidase) are involved in gibberellin (GA) biosynthesis, CYP85A1/2 and CYP90B1/C1/D1 in brassinosteroid biosynthesis, CYP735A1/2 in cytokinin biosynthesis, CYP74A (allene oxide synthase) in jasmonic acid biosynthesis, and CYP711A1 (MAX1) in strigolactone biosynthesis (Bishop and Konc, 2002; Turner et al., 2002; Sakakibara, 2006; Yamaguchi, 2008; Seto and Yamaguchi, 2014).
The loss-of-function cyp78a5 mutant produces smaller floral organs, owing to lower cell proliferation activity; conversely, CYP78A5 overexpression leads to enlargement of floral organs (Anastasiou et al., 2007). Given that cyp78a5 mutations affect the size of organs in which CYP78A5 is not expressed, CYP78A5 is believed to generate a mobile growth-stimulating signal molecule (Anastasiou et al., 2007). This hypothesis is further supported by elegant experiments using the Cre/loxP recombination system (Adamski et al., 2009; Eriksson et al., 2010). As the activities of CYP78A5-responsive genes do not overlap with those of known phytohormone-responsive genes and the provision of exogenous phytohormones does not rescue the cyp78a5 phenotype, it is considered that CYP78A5 acts independently in the synthesis and degradation of known phytohormones (Anastasiou et al., 2007). Thus, although neither the substrate nor product of CYP78A5 has been identified, the hypothesis that CYP78A5 is involved in the synthesis of a mobile signal molecule is well accepted.
The function of CYP78A5 is pleiotropic. For example, cyp78a5 mutants are characterized by a short plastochron (i.e. temporal rate of leaf initiation), early flowering, and reduced apical dominance in addition to reduced organ size phenotypes (Wang et al., 2008; Poretska et al., 2020). Furthermore, supernumerary egg cells are often observed during female gametophyte development (Zhao et al., 2018). In Arabidopsis, the CYP78A subfamily comprises six members, CYP78A5, A6, A7, A8, A9, and A10. Among the mutants of which, cyp78a7 was found to markedly enhance the phenotype of cyp78a5 with respect to the plastochron and apical dominance (Wang et al., 2008; Poretska et al., 2020). Furthermore, the cyp78a5 cyp78a7 double mutant is characterized by abnormal embryos with supernumerary cotyledons, notably small leaves, and sterility. Moreover, this mutant has a large shoot apical meristem (SAM) and ectopic shoot stem cell pools (Poretska et al., 2020). Although the functions of other CYP78A isoforms are yet to be investigated in detail, CYP78A6 (EOD3) and CYP78A9 have been found to redundantly regulate seed size, whereas CYP78A6 and CYP78A8 are involved in seed color development (Fang et al., 2012; Sotelo-Silveira et al., 2013).
In other species, PLASTOCHRON1 (PLA1), a probable rice (Oryza sativa) ortholog of CYP78A5, is characterized by a short plastochron and flower-to-shoot conversion (Itoh et al., 1998; Miyoshi et al., 2004). Another CYP78A family gene in rice, GIANT EMBRYO, is involved in the regulation of the embryo/endosperm size balance; that is, cell size in the embryo and cell death in the endosperm (Nagasawa et al., 2013). In tomato (Solanum lycopersicum), a mutation in the promoter region of SlKLUH, a member of the CYP78A subfamily, increases its expression, and has thereby contributed to increased fruit size during domestication (Chakrabarti et al., 2013). Thus, the CYP78A subfamily appears to have manifold functions in a diverse range of plant species.
A short plastochron is one of the characteristic cyp78a5 phenotypes. Several pleiotropic mutations involving the regulation of plastochron length have been described, including squamosa promoter binding protein-like9 (spl9), spl15, and altered meristem program1 (amp1; Chaudhury et al., 1993; Wang et al., 2008). While SPL9 and SPL15 encode transcription factors, AMP1 encodes an enzyme with homology to M28 family metalloproteases (Helliwell et al., 2001). Although AMP1 reportedly regulates microRNA (miRNA)-mediated translational repression and, similar to CYP78A5, localizes to the ER, the role of its carboxypeptidase activity remains to be elucidated (Li et al., 2013).
The phenotype of the amp1 mutant is also notably pleiotropic; for example, in addition to a short plastochron, amp1 plants show early flowering, reduced organ size, reduced apical dominance, supernumerary egg cell formation, abnormal embryos with supernumerary cotyledons, enlarged SAM, and ectopic shoot stem cell pools (Chaudhury et al., 1993; Kong et al., 2015; Poretska et al., 2020). AMP1 has a closely related paralog, namely, LIKE AMP1 (LAMP1; Li et al., 2013; Huang et al., 2015). The amp1 lamp1 double mutant shows an enhanced phenotype with respect to plastochron and organ size, has particularly small leaves, and is sterile. Interestingly, the phenotypes observed in amp1 and amp1 lamp1 mutants have also been reported in cyp78a5 and cyp78a5 cyp78a7 mutants. In addition, amp1 plants exhibit further pleiotropic phenotypes related to the juvenile–adult phase transition, hypocotyl elongation, photomorphogenesis, and stress response/seed dormancy via abscisic acid responsiveness (Chaudhury et al., 1993; Conway and Poethig, 1997; Saibo et al., 2007; Griffiths et al., 2011; Shi et al., 2013a, 2013b; Fouracre et al., 2020).
AMP1 orthologs in other species have also been demonstrated to have pleiotropic functions. For example, the mutant AMP1 ortholog plastochron3 (pla3) in rice is characterized by a short plastochron and reduced seed dormancy, which is similar to the traits of viviparous8, a mutant of the AMP1 ortholog in maize (Zea mays; Suzuki et al., 2008; Kawakatsu et al., 2009). The pla3 mutant also shows impaired flower development, as observed in the pla1 mutant, thereby indicating that the AMP1 ortholog has functional similarity to the CYP78A5 ortholog in rice. Additionally, the AMP1 ortholog in Lotus japonicus (TRICOT) regulates plastochron and root nodule development, thereby confirming that AMP1 orthologs have pleiotropic functions in a wide range of species (Suzaki et al., 2013).
Given that CYP78A5 and AMP1 appear to have very similar functions, it might be predicted that CYP78A5 and AMP1 act in the same signaling pathway. To examine the probability of this supposition, we initially investigated the functions of different CYP78A isoforms in Arabidopsis using double mutants and complementation analyses, focusing on vegetative-stage traits, including the plastochron, leaf size, and dark-induced leaf senescence. The results indicated that CYP78A isoforms have a common biochemical function and are functionally diverged via organ-specific expression. We performed genetic analyses for the ubiquitously expressed AMP1/LAMP1 and CYP78A isoform genes, revealing that they (at least partly) use the same signaling pathway in the regulation of plastochron and leaf senescence, but regulate leaf size independently. These results indicate a close but complex relationship between the CYP78A and AMP1 pathways. Furthermore, our observations indicate that both CYP78A5 and AMP1 can act in a non-cell-autonomous manner in plastochron regulation, suggesting the possibility that CYP78A and AMP1 are involved in the synthesis of a common mobile signal molecule.
Results
Plastochron and leaf size in CYP78A isoform mutants
Cytochrome P450 CYP78A isoforms are involved in the regulation of various phenomena throughout plant development. Their functions in post-flowering events, such as flower and seed development, have previously been analyzed in detail in Arabidopsis (Anastasiou et al., 2007; Adamski et al., 2009; Fang et al., 2012; Sotelo-Silveira et al., 2013). Therefore, in this study, we focused on the role of CYP78A isoforms in leaf-related traits at the vegetative stage, including plastochron, leaf size, and leaf senescence.
CYP78A5 or KLUH, which is the most well-characterized of the six CYP78A isoforms in Arabidopsis, is known to be involved in the regulation of leaf size, plastochron, and tooth formation at the leaf margin (Wang et al., 2008; Maugarny-Calès et al., 2019). Although cyp78a7 does not result in a prominent phenotype, it does markedly enhance the cyp78a5 phenotype with respect to plastochron and leaf size, thereby indicating that CYP78A5 and CYP78A7 are functionally redundant (Wang et al., 2008). Three other CYP78A isoforms, CYP78A6, CYP78A8, and CYP78A9, have been shown to be involved in post-flowering traits such as floral organ size, seed size, and seed color (Fang et al., 2012; Sotelo-Silveira et al., 2013). Among the six CYP78A isoforms, only CYP78A10 was not found to be expressed in shoots (data not shown); thus, we characterized the functions of the remaining five isoforms (CYP78A5, CYP78A6, CYP78A7, CYP78A8, and CYP78A9) during vegetative growth.
As a shortened plastochron is one of the major characteristics of the cyp78a5 mutant at the vegetative stage, we examined the leaf number of single mutants of the five CYP78A isoforms. Counting leaf number over time in the cyp78a single mutants until 28 d after germination revealed that only the cyp78a5-11 mutant showed a short plastochron phenotype (Supplemental Figure S1A). Although the cyp78a7 mutant does not result in the short plastochron phenotype, it is known to markedly enhance the plastochron of the cyp78a5 mutant. Therefore, we generated cyp78a5 cyp78a6, cyp78a5 cyp78a8, and cyp78a5 cyp78a9 double mutants and examined their plastochron phenotype (Figure 1, A and B). A cyp78a5-11 mutant created by a transfer (T)-DNA insertion in the proximal region of the CYP78A5 promoter was used to generate the cyp78a5 cyp78a6 and cyp78a5 cyp78a9 mutants, whereas a cyp78a5-12 mutant created by clustered regularly interspaced short palindromic repeats (CRISPRs)/CRISPR-associated protein 9 (Cas9)-based genome editing was used to generate the cyp78a5 cyp78a8 mutant (Supplemental Figure S1B). The cyp78a5-12 allele is considered a null allele, as it is characterized by a single-base deletion in the first exon that results in a frameshift. We detected no statistically significant difference in leaf number between cyp78a5-11 and cyp78a5-12 plants, indicating that cyp78a5-11 (cyp78a5 hereafter) is equivalent to a null allele (Supplemental Figure S1, C and D). The cyp78a5 cyp78a6, cyp78a5 cyp78a9, and cyp78a5-12 cyp78a8 mutants showed the same plastochron length as cyp78a5 and cyp78a5-12 plants (Figure 1B; Supplemental Figure S1D). Furthermore, although the cyp78a5 cyp78a7 mutant was shown to produce notably small leaves, the leaf sizes of cyp78a5 cyp78a6, cyp78a5-12 cyp78a8, and cyp78a5 cyp78a9 plants were similar to those of cyp78a5 and cyp78a5-12 plants (Figure 1A; Supplemental Figure S1C). Thus, CY78A6, CYP78A8, and CYP78A9 are not involved in plastochron and leaf size regulation.
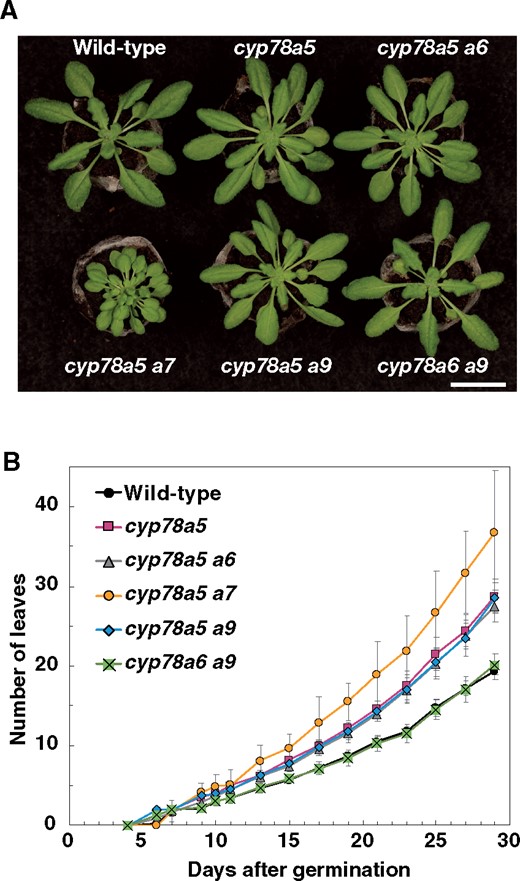
Plastochron length of the cyp78a double mutants. A, cyp78a mutants grown for 22 d under short-day conditions. B, Changes over time in the total number of visible true rosette leaves. Values are the means ± SD (n ≥ 9). Bar in (A) = 2 cm.
Leaf senescence in cyp78a isoform mutants
Leaf senescence is the final step in leaf development; whereas leaf senescence can be induced by different stimuli, dark-induced senescence is the best characterized (Ueda et al., 2020). Therefore, we investigated the phenotype of cyp78a isoform mutants during dark-induced leaf senescence. Rosette leaves that emerged on the same day (23 d after germination) were detached and incubated under dark conditions at 22°C. We observed that chlorophyll content in the leaves of wild-type plants declined substantially after 4 d of dark incubation (Figure 2A). Among the single mutants of CYP78A isoforms, the chlorophyll content of cyp78a6 and cyp78a9 declined earlier than that in the wild-type (Figure 2A). Similarly, we found that reductions in Fv/Fm values, which reflect the activity of photosystem II, were also greater in the leaves of cyp78a6 and cyp78a9 than in the wild-type leaves, thereby indicating that cyp78a6 and cyp78a9 are early senescence mutants rather than early yellowing mutants (Figure 2B). To examine functional redundancy, we generated a cyp78a6 cyp78a9 double mutant and measured the chlorophyll content and Fv/Fm values under the aforementioned conditions (Figure 2, A and B). We found that both chlorophyll content and Fv/Fm values declined earlier in the cyp78a6 cyp78a9 double mutant than in either of the cyp78a6 or cyp78a9 single mutants, indicating that CYP78A6 and CYP78A9 redundantly regulate leaf senescence. Consistent with this assumption, the expression of the senescence-marker gene STAY GREEN (SGR1) was upregulated earlier in the cyp78a6 cyp78a9 double mutant than in the wild-type (Supplemental Figure S2, A–C). Furthermore, we generated a cyp78a6 cyp78a8 cyp78a9 triple mutant and found that there was no difference in the progression of dark-induced senescence between the cyp78a6 cyp78a9 double mutant and the triple mutant, thereby providing evidence that CYP78A8 is not involved in leaf senescence (Figure 2, A and B). Collectively, these observations indicate that CYP78A6 and CYP78A9 redundantly suppress leaf senescence.
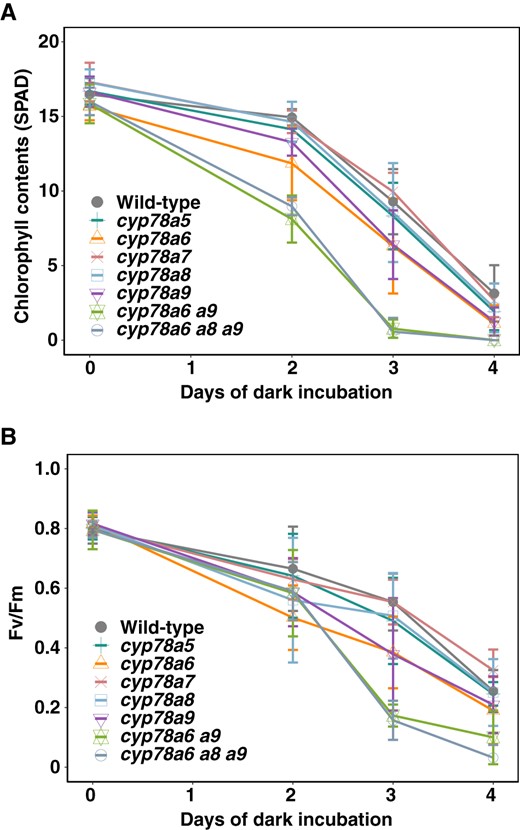
Leaf senescence of the CYP78A isoform mutants. A, Change in the chlorophyll content over time in the cyp78a mutants during dark incubation. B, Change in Fv/Fm value over time in the cyp78a mutants during dark incubation. Values are the means ± SD (n = 6).
Expression of CYP78A isoform genes
We examined the organ- and stage-specific expression of CYP78A isoform genes by reverse transcription-quantitative PCR (RT-qPCR) analysis (Figure 3A). Although CYP78A5 is reportedly expressed in the proximal region of very young leaves (YLs), we detected no expression in young, mature, or senescing/senescent leaves (Figure 3A; Kazama et al., 2010; Maugarny-Calès et al., 2019). Similarly, we were unable to detect any CYP78A7 expression in leaves at the developmental stages examined, indicating that CYP78A5 and CYP78A7 are exclusively expressed in the shoot apices (SAs). Contrastingly, CYP78A6 was expressed in leaves from the young to senescent stage, whereas CYP78A8 was expressed in young and mature leaves, although little expression was observed in senescing/senescent leaves. CYP78A9 was found to be expressed predominantly in senescing/senescent leaves. We also detected certain levels of CYP78A6, CYP78A8, and CYP78A9 expression in the shoot apex (Figure 3A). In addition, the expression of CYP78A6, CYP78A8, and CYP78A9 gradually decreased in the dark, but only CYP78A8 increased after 6 d of dark incubation (Supplemental Figure S2D).
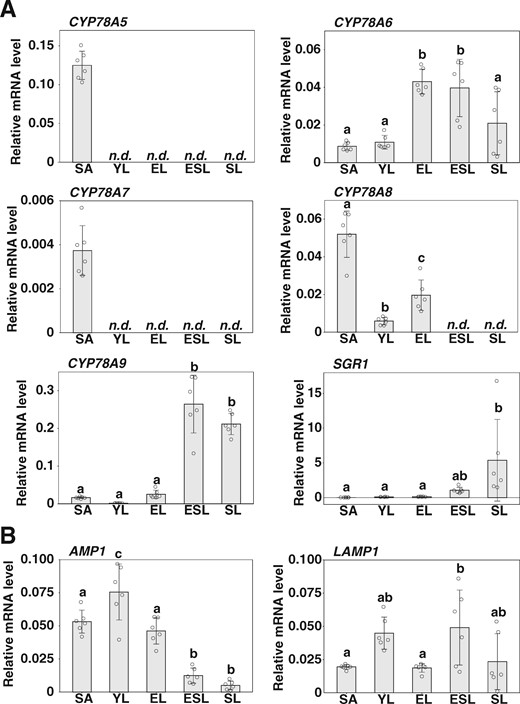
Expression of CYP78A isoform genes and AMP1/LAMP1. A, RT-qPCR analysis of CYP78A isoform gene expression. B, RT-qPCR analysis of the expression of AMP1 and LAMP1. SAs, YLs, fully expanded leaves (EL), leaves in the early stages of natural senescence (ESL), and leaves in the late stages of natural senescence (SL) from plants grown under long-day conditions were analyzed. ACT8 was used as a reference. Values are the means ± SD (n = 6, biological replicates). Statistical differences were analyzed by one-way ANOVA followed by a Tukey–Kramer multiple comparison tests (P < 0.05). n.d., not detected.
To characterize the expression patterns of CYP78A isoform genes in SAs, we referred to the Translating ribosome affinity purification followed by sequencing (TRAP-seq) database (Supplemental Figure S3; Tian et al., 2019). Interestingly, we found that CYP78A5 and CYP78A7 are highly expressed in the shoot apex, particularly in the boundary domain (the LATERAL SUPPRESSOR (LAS) domain). In contrast, CYP78A6, CYP78A8, and CYP78A9 expression levels are low in the boundary domain and SAM (the WUSCHEL (WUS), CLAVATA3, and UNUSUAL FLORAL ORGANS domains). Although RT-qPCR analysis showed that relative expression of CYP78A8 was higher in the shoot apex compared with other tissues, the absolute level of expression may be low, even in the shoot apex, according to the TRAP-seq analysis results (Figure 3A; Supplemental Figure S3). The difference might be partly due to the fact that the shoot apex samples included some other tissues surrounding the shoot apex. These observations thus indicate a close correlation between the organ specificity of expression and the function of CYP78A isoform genes; that is, CYP78A5 and CYP78A7, which are strongly expressed in the shoot apex, are involved in plastochron and leaf size regulation, whereas CYP78A6 and CYP78A9, which are expressed in mature leaves, are involved in leaf senescence progression.
Functional commonality of CYP78A5 and CYP78A6
To examine whether CYP78A isoforms have a common biochemical function, we attempted complementation of cyp78a5 by CYP78A6. To this end, we introduced a CYP78A6–Venus construct driven by the CYP78A5 promoter into the cyp78a5 mutant using Agrobacterium tumefaciens-mediated transformation. We observed that CYP78A6–Venus was strongly expressed in the boundary domain between the SAM and leaf primordium or between leaf primordia, and weakly expressed in the SAM (Figure 4A; Supplemental Figure S4). Accordingly, we observed that the number of leaves developed by 26-d-old plants of three independent transgenic lines was similar to that in wild-type plants, thereby indicating that the function of CYP78A5 can be replaced by that of CYP78A6 when expressed in the peripheral domain of the SAM (Figure 4, B and C).
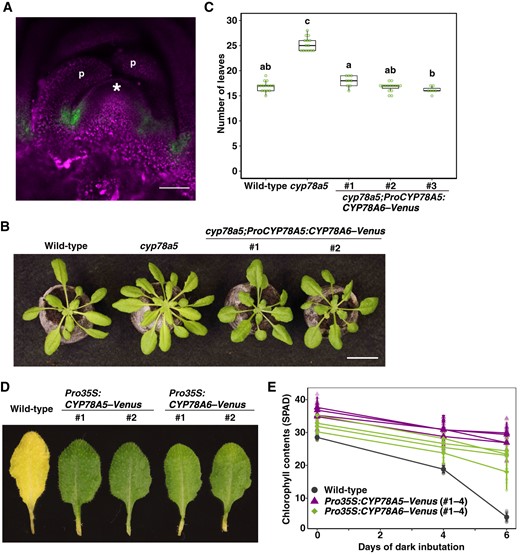
CYP78A5 and CYP78A6 are characterized by a common biochemical property. A, Confocal microscopic image of the shoot apex of a cyp78a5 plant harboring a CYP78A5 promoter-driven CYP78A6–Venus transgene. Fluorescence signals of Venus (green) were merged with the autofluorescence (magenta). Bar, 50 µm. * and ‘p’ indicate the SAM and leaf primordium, respectively. B, Phenotypes of cyp78a5 plants harboring a CYP78A5 promoter-driven CYP78A6–Venus transgene. Bar, 2 cm. C, The number of visible true rosette leaves of the transgenic plants described in (B). Plants were grown for 26 d under short-day conditions. Statistical differences were analyzed by one-way ANOVA followed by a Tukey–Kramer multiple comparison tests (P < 0.01). n = 7–15. D, Dark-incubated detached leaves carrying a CaMV35SRNA promoter-driven CYP78A5–Venus or CYP78A6–Venus transgene. Leaves were incubated for 6 d in the dark. E, Change over time in the chlorophyll content of transgenic plants. Four independent transgenic lines were used in each construct. Values are the means ± SD (n = 8).
We went on to generate transgenic plants carrying CaMV35SRNA promoter-driven CYP78A5–Venus or CYP78A6–Venus transgenes and found that plants overexpressing CYP78A6 showed delayed leaf senescence during dark incubation (Figure 4, D and E), which is consistent with the assumption that CYP78A6 is involved in the repression of leaf senescence. Interestingly, high-level ectopic expression of CYP78A5 in leaves, the mutant of which does not show a leaf senescence phenotype, induced a delayed senescence phenotype during dark incubation (Figure 4, D and E). These observations thus indicate that CYP78A5 and CYP78A6 share a common biochemical function, although they are functionally diverged as a consequence of organ-specific expression.
Meanwhile, CYP78A5 promoter-driven CYP78A6–Venus was expressed mainly in the boundary domain, but CYP78A5 promoter-driven β-glucuronidase was expressed more broadly in the shoot apex (Poretska et al., 2020). It is possible that an element in the CYP78A coding sequence modifies its own expression.
The CYP78A5 signal as an intercellular signal within the shoot apex
Reportedly, CYP78A9 is localized in the plasma membrane, whereas CYP78A5 is located in the ER (Anastasiou et al., 2007; Sotelo-Silveriea et al., 2013). It can be reasoned that if CYP78A isoforms have a common function, they are likely to have a similar subcellular localization. Therefore, we re-examined the subcellular localization of CYP78A isoforms based on transient co-expression of the ER marker ECFP-HDEL and CYP78A–Venus constructs in the Arabidopsis T87 cultured cell line (Supplemental Figure S5). Accordingly, we detected the co-localization of the signals of ECFP-HDEL and CYP78A9–Venus florescent proteins, indicating that similar to CYP78A5, CYP78A9 is localized in the ER. Additionally, we examined the subcellular localization of CYP78A6 using the same strategy and found that CYP78A6 is also localized in the ER. These results suggest that CYP78A isoforms are generally localized in the ER.
CYP78A5 is strongly expressed in the peripheral domains of the SAM, particularly in the boundary domain of the shoot apex (Zondlo and Irish, 1999). Thus, to examine the importance of CYP78A5 expression in the boundary domain of the shoot apex with regard to plastochron regulation, we performed complementation analysis of cyp78a5 using the CYP78A5 transgene driven by the LAS promoter, which is active in the boundary domains, and the WUS promoter, which is active in the organizing center (OC). In line with expectations, the LAS promoter-driven CYP78A5, which induces expression in the domain where CYP78A5 is strongly expressed, was observed to revert the increase in leaf number in the cyp78a5 mutant to the wild-type level in both short-day and long-day conditions (Figure 5; Supplemental Figure S6A). Similarly, we found that the leaf number of transgenic plants carrying the WUS promoter-driven CYP78A5 was also reverted to the wild-type level (Figure 5; Supplemental Figure S6A). As with plastochron length, the small leaf size of cyp78a5 was reversed by both LAS promoter-driven and WUS promoter-driven CYP78A5 (Supplemental Figure S6, B and C). These observations are consistent with the prediction that CYP78A5 (KLUH) synthesizes a mobile signal molecule (Anastasiou et al., 2007; Eriksson et al., 2010).
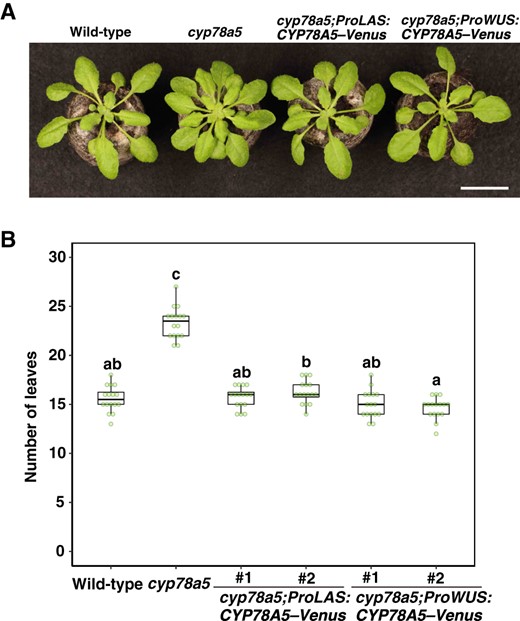
CYP78A5 regulates the plastochron non-cell-autonomously in the shoot apex. A, Phenotypes of cyp78a5 plants harboring LAS promoter- or WUS promoter-driven CYP78A5–Venus transgenes. Bar, 2 cm. B, The number of visible true rosette leaves of the transgenic plants described in (A). Plants were grown under short-day conditions for 23 d. Statistical differences were analyzed by one-way ANOVA followed by a Tukey–Kramer multiple comparison tests (P < 0.001). n = 16.
Regulation of plastochron and leaf size by AMP1/LAMP1
The amp1 mutant is characterized by a short plastochron phenotype. LAMP1 is a close paralog of AMP1, characterized by similar biochemical functions. We found that the amp1 lamp1 double mutant showed a considerably shorter plastochron phenotype (Figure 6A; Huang et al., 2015; Poretska et al., 2020). In addition to an extremely short plastochron and small leaf size, the cyp78a5 cyp78a7 mutant has other notable pleiotropic phenotypes, including abnormal embryo genesis, an occasional increase in the number of cotyledons, early flowering, small flower size, and sterility (Wang et al., 2008). Furthermore, we also observed reduced apical dominance and occasional fasciation (Supplemental Figure S7). Interestingly, the amp1 lamp1 mutant showed a phenotype similar to that of the cyp78a5 cyp78a7 mutant; all the aforementioned cyp78a5 cyp78a7 phenotypes were also observed in the amp1 lamp1 mutant. However, compared with the cyp78a5 cyp78a7 mutant, we found that organ size was relatively smaller and the plastochron was shorter in the amp1 lamp1 double mutant (Supplemental Figure S7, A and E).

Domain-specific expression of AMP1 in SAs is sufficient for the control of plastochron length. A, Seedlings of cyp78a5, amp1, cyp78a5 cyp78a7, and amp1 lamp1 mutants. Plants were grown for 21 d under short-day conditions. Bar, 2 cm. B, Confocal microscopic images of the SAs of the amp1 plants harboring LAS promoter-driven AMP1–Venus (left) and WUS promoter-driven AMP1-Venus (right) transgenes. Fluorescence signals of Venus (green) were merged with the chlorophyll autofluorescence (magenta). * and “p” indicate the SAM and leaf primordium, respectively. Bars, 50 µm. C, amp1 plants harboring the LAS promoter- or WUS promoter-driven AMP1-Venus transgenes. D, The number of visible true rosette leaves of the transgenic plants described in (C). Plants were grown under short-day conditions for 23 d. Statistical differences were analyzed by one-way ANOVA followed by a Tukey–Kramer multiple comparison tests (P < 0.001). n = 16. Bar, 2 cm.
We further observed that both AMP1 and LAMP1 were expressed in SAs and in young, mature, and senescing/senescent leaves (Figure 3B). Our search of the TRAP-seq database revealed that AMP1 is expressed ubiquitously in the shoot apex, including the SAM, boundary, and leaf primordia, whereas the expression of LAMP1 is relatively lower in the SAM and boundary than in leaf primordia (Supplemental Figure S3). To determine the important domain for plastochron regulation in the shoot apex, we attempted complementation of amp1 using AMP1–Venus driven by the LAS or WUS promoter (Figure 6, B–D). As conjectured, we observed that AMP1–Venus proteins driven by the LAS and WUS promoters were specifically localized in the boundary domain and the OC of the shoot apex, respectively (Figure 6B). The transgenic plant lines harboring the LAS-promoter and WUS-promoter transgenes produced numbers of rosette leaves similar to those produced by wild-type plants under both short-day and long-day conditions, thereby indicating that both transgenes can complement the plastochron phenotypes of the amp1 mutant (Figure 6, C and D; Supplemental Figure S8A). Similarly, both the LAS promoter-driven and WUS promoter-driven AMP1 reversed the leaf size phenotype of the amp1 mutant (Supplemental Figure S8, B and C). Thus, although AMP1 is expressed in both the boundary and OC domains of the shoot apex, these observations indicate that AMP1 can regulate the plastochron in a non-cell-autonomous manner.
Regulation of leaf senescence by AMP1
Here, we reasoned that CYP78A isoforms would have the same biochemical function. Given that cyp78a5 cyp78a7 and amp1 lamp1 double mutants are characterized by similar phenotypes, we examined whether the leaf senescence phenotype observed in the cyp78a6 cyp78a9 mutant is also seen in the amp1 mutant (Figure 7, A and B). Leaves that emerged on the same day were incubated in the dark and changes in the chlorophyll content and Fv/Fm values over time were measured. Interestingly, as in the cyp78a6 cyp78a9 mutant, we found that the chlorophyll content and Fv/Fm value in the amp1 mutant declined earlier than in the wild-type (Figure 7, A and B). These observations indicate that AMP1 has a functional profile that encompasses the activities of all CYP78A isoforms; that is, AMP1 performs functions corresponding to CYP78A5 and CYP78A7 in the shoot apex, such as plastochron regulation, and functions corresponding to CYP78A6 and CYP78A9 in leaves, such as regulation of leaf senescence.
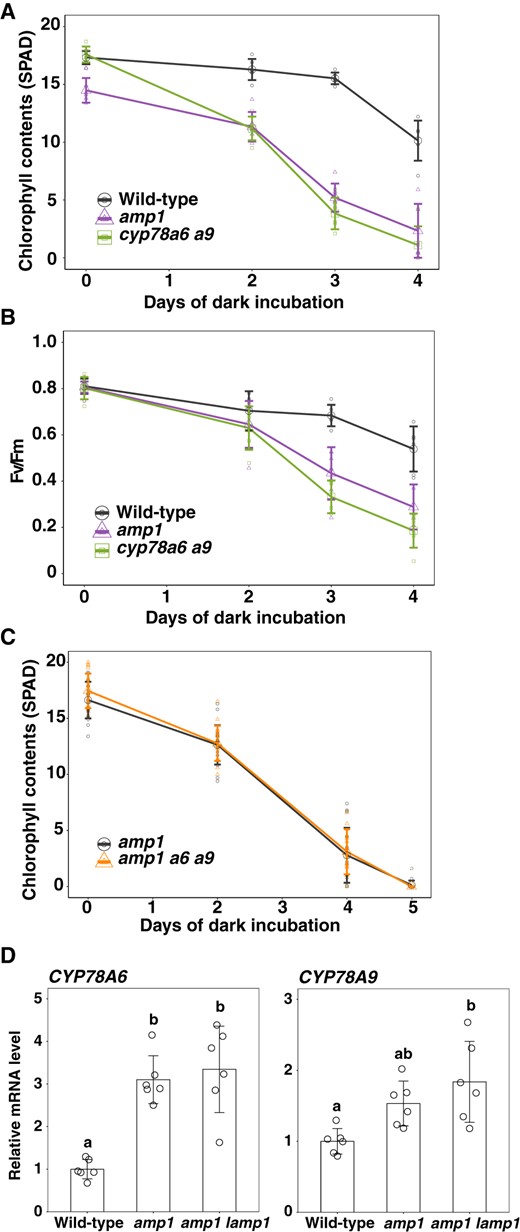
Genetic interaction between CYP78A isoform genes and AMP1/LAMP1 during leaf senescence. A, Change in the chlorophyll content over time in amp1 plants during dark incubation. Values are the mean ± SD (n = 7). B, Change in Fv/Fm values over time in amp1 plants during dark incubation. Values are the means ± SD (n = 7). C, Change in the chlorophyll content over time in amp1 cyp78a6 cyp78a9 plants during dark incubation. Values are the means ± SD (n = 18). D, Expression of CYP78A6 and A9 in mature leaves of amp1 and amp1 lamp1 plants. Plants were grown for 21 d under short-day conditions. Transcript levels were relative to those in wild-type leaves. ACT8 was used as a reference. Values are the mean ± SD (n = 6, biological replicates). Statistical differences were analyzed by one-way ANOVA followed a Tukey–Kramer multiple comparison test (P < 0.05).
Genetic interactions between AMP1/LAMP1 and CYP78A isoform genes
To examine the genetic interaction between AMP1 and CYP78A6/CYP78A9 in leaf senescence, we generated an amp1 cyp78a6 cyp78a9 triple mutant and compared its characteristics with those of the amp1 mutant (Figure 7C). Accordingly, we observed that amp1 and amp1 cyp78a6 cyp78a9 plants showed similar progression of dark-induced leaf senescence, thereby indicating that AMP1 and CYP78A6/CYP78A9 function in the same genetic pathway involved in leaf senescence.
As CYP78A5 was reported to be upregulated in the amp1 mutant, we examined the expression of CYP78A6 and CYP78A9 in the leaves of amp1 and amp1 lamp1 plants (Figure 7D; Helliwell et al., 2001). Compared with the wild-type, we found that CYP78A6 expression was upregulated by 3.2- and 3.3-fold in amp1 and amp1 lamp1 plants, respectively, and that CYP78A9 expression was correspondingly upregulated by 1.5- and 1.8-fold, respectively. Assuming that AMP1 and CYP78A6/CYP78A9 function in the same signaling pathway, it is conceivable that CYP78A6 and CYP78A9 are upregulated as a consequence of feedback regulation involving AMP1. However, we found that neither AMP1 nor LAMP1 was upregulated in the cyp78a6 cyp78a9 mutant in either presenescent or senescing leaves, indicating that AMP1 and LAMP1 are not under the control of feedback regulation involving CYP78A6/CYP78A9 (Supplemental Figure S2E).
To examine the genetic interaction of AMP1/LAMP1 and CYP78A5 with regard to plastochron regulation and leaf/plant size, we subsequently generated an amp1 lamp1 cyp78a5 triple mutant and compared the characteristics of this mutant with those of amp1 lamp1 plants (Figure 8). We did not detect significant differences in the leaf numbers of 16-d-old amp1 lamp1 and cyp78a5 amp1 lamp1 plants, indicating that CYP78A5 and AMP1/LAMP1 act in the same genetic pathway involved in plastochron regulation (Figure 8B). However, the size of leaves produced by the amp1 lamp1 cyp78a5 mutant, as well as the overall plant size, appeared to be smaller than those of the amp1 lamp1 mutant, thereby indicating an independent genetic interaction between CYP78A5 and AMP1/LAMP1 in the regulation of leaf/plant size (Figure 8C). These observations indicate that both the plastochron and leaf/plant size are regulated by AMP1/LAMP1 and CYP78A5, although the manner of regulation differs at least partially.
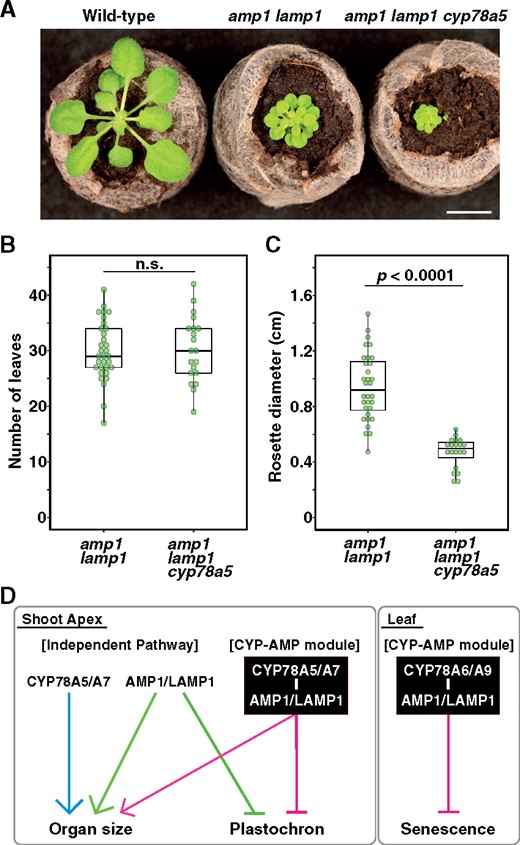
Genetic interaction between CYP78A5 and AMP1/LAMP1 in plastochron and leaf size regulation. A, Phenotypes of amp1 lamp1 and amp1 lamp1 cyp78a5 mutants grown for 16 d under short-day conditions. Bar, 2 cm. B, Number of visible true rosette leaves of amp1 lamp1 and amp1 lamp1 cyp78a5 mutants. C, Rosette diameter of amp1 lamp1 and amp1 lamp1 cyp78a5 mutants. Statistical differences were analyzed using Welch’s t test. n = 32 and 20 for amp1 lamp1 and amp1 lamp1 cyp78a5, respectively. D, Model of functions of CYP78A isoforms and AMP1/LAMP1. CYP78A5/CYP78A7 and AMP1/LAMP1 regulate plastochron and organ size in a non-cell-autonomous manner in the shoot apex. They function as a “CYP-AMP module” as well as in independent pathways. CYP78A6/CYP78A9 and AMP/LAMP regulate leaf senescence.
Discussion
Functional overlap between CYP78A isoforms and AMP1/LAMP1
Among the pleiotropic functions of CYP78A isoform genes, those that are performed at the reproductive stage, such as flower and seed size regulation, have attracted considerable attention. In this study, however, we focused on the functions of CYP78A isoforms at the vegetative stage, specifically those associated with plastochron regulation, leaf size, and dark-induced leaf senescence. Our double mutant analyses revealed that CYP78A5 and CYP78A7 play prominent roles in the regulation of plastochron and leaf size, while CYP78A6 and CYP78A9 are important in regulating leaf senescence.
We found that ectopic expression of CYP78A6 reversed the plastochron and leaf size phenotype of the cyp78a5 mutant and that overexpression of CYP78A6 and high-level ectopic expression of CYP78A5 resulted in a delayed-senescence phenotype, thereby indicating that CYP78A isoforms have similar biochemical functions. The absence of expression of CYP78A5 and CYP78A7 in leaves and the low expression of CYP78A6 and CYP78A9 in SAs indicate that the functional differences between CYP78A5/CYP78A7 and CYP78A6/CYP78A9 can be attributed to their organ-specific expression, rather than to differences in biochemical functions. Accordingly, CYP78A6 and CYP78A9, which are expressed in leaves, are involved in the regulation of leaf senescence, whereas CYP78A5 and CYP78A7, which are expressed in SAs, are involved in the regulation of the plastochron and leaf size.
The amp1 lamp1 double mutant showed highly pleiotropic phenotypes, including a particularly short plastochron, reduced organ size, early flowering, reduced apical dominance, sterility, occasional fasciation, and hypertrophy in the embryo and SAM, which coincide with the phenotypes observed in the cyp78a5 cyp78a7 double mutant. Furthermore, the amp1 mutant shows an early-senescence phenotype, which is also observed in the cyp78a6 cyp78a9 mutant (Figure 2). Very recently, Poretska et al. (2020) examined the functions of CYP78A5/CYP78A7 and AMP1/LAMP1 in meristem patterning; their detailed analysis indicated that cyp78a5 cyp78a7 and amp1 lamp1 double mutants show nearly identical defects in meristem patterning in embryos and seedlings. However, whereas CYP78A isoforms have become functionally differentiated via organ-specific expression, AMP1 is expressed almost ubiquitously (Helliwell et al., 2001; Vidaurre et al., 2007). The similarity in their functions and the differences in their expression suggest that the ubiquitous expression of AMP1, at least in part, integrates the functions of the organ-specific CYP78A isoforms.
We found that the expression of CYP78A6 and CYP78A9 was upregulated in the leaves of amp1 plants (Figure 3), which could be attributed to feedback regulation. Similarly, the expression of CYP78A5 is also reportedly upregulated in amp1 plants, although the enhanced expression is thought to be due to enlargement of the SAM in amp1 plants (Anastasiou et al., 2007). However, considering the functional overlap between CYP78A isoforms and AMP1/LAMP1 described above, we suspect that the upregulation of CYP78A5 in the amp1 mutant might also, at least in part, be a consequence of feedback regulation. Thus, it appears that CYP78A isoforms and AMP1/LAMP1 have closely related functions in a range of phenomena associated with plant development.
CYP78A isoforms and AMP1 act in a non-cell-autonomous manner
The fact that expression of CYP78A5 in the boundary domain can complement the cyp78a5 phenotype confirms that expression in a confined domain in the shoot apex is sufficient to regulate plastochron length. Furthermore, we found that the expression of CYP78A5 in the OC can also complement the cyp78a5 phenotype (Figure 5), indicating that CYP78A5 can act in a noncell-autonomous manner. This assumption is congruent with the well-accepted hypothesis that CYP78A5 is involved in the synthesis of a previously unidentified mobile signal molecule (Anastasiou et al., 2007; Eriksson et al., 2010).
The CYP78A5 signal is transmitted between flowers in an inflorescence, but not between inflorescences and rosette leaves (Eriksson et al., 2010). Furthermore, Maugarny-Calès et al. (2019) suggested that in leaf margin tooth formation, the CYP78A5 signal functions within a range of a few hundred micrometers. Our complementation analysis based on the domain-specific expression of CYP78A5 indicated that the CYP78A5 signal in the shoot apex diffuses within a range of 100 µm (Figure 5). Furthermore, the functional differentiation of CYP78A isoforms via organ specificity provides evidence that the CYP78A5 signal is not transmitted beyond organs, for instance between the SAM and leaves. Additionally, our observation that CYP78A6 mainly expressed in the boundary domain in the shoot apex complemented the phenotype of the cyp78a5 mutant is consistent with the assumption that CYP78A isoforms generally act non-cell-autonomously (Figure 4).
Similarly, we found that the short plastochron phenotype of the amp1 mutant was complemented by the boundary- or OC-specific expression of AMP1 (Figure 6, C and D), indicating that AMP1 can also act in a non-cell-autonomous manner, although AMP1 is expressed ubiquitously, including in both boundary and OC domains (Vidaurre et al., 2007). Consistent with these results, Kong et al. (2015) also claimed that AMP1-dependent signals are mobile in developing ovules during synergid speciation. As cytokinin levels are reportedly elevated in the amp1 mutant, it is conceivable that the non-cell-autonomously acting factor could be cytokinin (Chaudhury et al., 1993). However, the phenotype observed in amp1 plants is unlikely to be due to elevated cytokinin levels (Huang et al., 2015).
Previous studies indicated that AMP1 is involved in the regulation of miRNA-mediated translational repression, but also may function independently of miRNA-mediated regulation (Li et al., 2013; Fouracre et al., 2020). Interestingly, AMP1 belongs to the M28 family of metalloproteases, the human homologs of which hydrolyze the neuropeptide N-acetyl-l-aspartyl-l-glutamate (Pangalos et al., 1999). Considering the non-cell-autonomous actions of AMP1 and CYP78A isoforms and the close relationship between these, it can be speculated that AMP1 might function in conjunction with CYP78A isoforms in the synthesis of mobile signal molecules.
Genes encoding enzymes involved in phytohormone synthesis are often targets of negative regulation via master transcription factors of the associated signaling pathway. For example, transcription of GA synthesis genes is repressed by the GAI-ASSOCIATED FACTOR1-TOPLESS RELATED complex (Fukazawa et al., 2014) and brassinosteroid synthesis genes are repressed by BRASSINAZOLE RESISTANT1 (Wang et al., 2002). RAP2.6L has been identified as a downstream transcription factor of AMP1, and possibly of CYP78A5 (Yang et al., 2018; Poretska et al. 2020). Although the commitment of RAP2.6L to the transcriptional feedback regulation of CYP78A6/CYP78A9 observed in the amp1 mutant has not been investigated, it might be controlled by levels of the mobile signal molecule. Furthermore, we established that the transcription of AMP1/LAMP1 is not controlled by negative feedback regulation (Supplemental Figure S2E). Although overexpression of AMP1 does not alter shoot meristem activity (Poretska et al., 2020), we found that overexpression of CYP78A5 and CYP78A6 was associated with several phenotypes, including delayed senescence (Figure 4, D and E), suggesting that the step catalyzed by CYP78A isoforms is rate-limiting, whereas that catalyzed by AMP1 is not.
Genetic relationship between CYP78A and AMP1 signaling pathways
The amp1 lamp1 double mutant appears to show more pleiotropic phenotypes than the cyp78a isoform mutants. For example, the amp1 mutant shows defects in photomorphogenesis, an impaired adult-juvenile transition, and altered abscisic acid responsiveness, which have not been reported in any cyp78a isoform mutants to date. Although validation of this view may require the generation of a sextuple mutant carrying mutations of all CYP78A isoform genes, it is difficult to explain all the functions of AMP1/LAMP1 in terms of a single signaling pathway common to CYP78A isoforms. Consistent with this view, despite the substantial functional overlap between CYP78A isoforms and AMP1/LAMP1, we revealed that their genetic interactions are probably complex. The cyp78a6 cyp78a9 double mutation did not enhance the early senescence phenotype of the amp1 mutant (Figure 7C), indicating that AMP1 and CYP78A6/CYP78A9 act in the same genetic pathway in the regulation of leaf senescence. Similarly, cyp78a5 did not enhance the short plastochron phenotype of the amp1 lamp1 mutant (Figure 8B), suggesting that CYP78A5 and AMP1/LAMP1 play roles in a common genetic pathway involved in the regulation of the plastochron length. However, we observed that amp1 lamp1 plants produced a larger number of leaves than did those of the cyp78a5 cyp78a7 mutant (Supplemental Figure S8E), indicating that the effects of impairments of the CYP78A5/CYP78A7 and AMP1/LAMP1 pathways on plastochron regulation are not equal. Consequently, it is conceivable that in addition to the pathway common to CYP78A5/CYP78A7, AMP1/LAMP1 uses an alternative genetic pathway in regulation of the plastochron. Furthermore, we found that cyp78a5 enhanced the small leaf/plant size phenotype of the amp1 lamp1 mutant, implying that the plastochron and leaf size are differentially regulated and that the small leaf size phenotype is not compensation for an increase in leaf number.
Poretska et al. (2020) proposed that whereas AMP1 and CYP78A5/CYP78A7 regulate meristem patterning independently, they are associated with common downstream processes. The findings of this study indicate that, depending on the trait, CYP78A isoforms and AMP1/LAMP1 function in parallel or through a common signaling pathway (Figure 8D). These observations could be explained by the bifunctionality of the AMP1/LAMP1 and CYP78A isoforms. AMP1 not only regulates the repression of miRNA-mediated translation but also could function as a carboxypeptidase, whereas CYP78A isoforms may be involved in the synthesis of more than one signaling molecule. Accordingly, shared traits may, for example, be regulated by a common signal molecule, whereas nonshared or shared but partly independently controlled traits may be regulated by miRNA-mediated translational repression via AMP1 or an alternative signal molecule synthesized by CYP78A isoforms.
Although more than a decade has passed since Anastasiou et al. (2007) first proposed the existence of a mobile signal molecule produced by CYP78A5, this molecule has yet to be identified. In part, this may be because CYP78A5 is expressed only in the boundary domains around the meristems of shoots and inflorescences. Our results indicate that the same molecule is produced in leaves by CYP78A6 and CYP78A9, which may facilitate biochemical analysis of the molecule. In this regard, the fatty acid hydroxylation activity of CYP78A isoforms has been reported (Imaishi et al., 2000; Kai et al., 2009). Although it remains unclear whether AMP1/LAMP1 is involved in the synthesis of the signal molecule as carboxypeptidases, it is expected that identification of the substrate or product of CYP78A isoforms will provide important insights into the growth-regulating mechanisms involving the CYP78A–AMP1 module.
Materials and methods
Plant materials and growth conditions
Seeds of A. thaliana cyp78a5-11 (SALK_024697), cyp78a6 (CS833552), cyp78a7 (SALK_140985), cyp78a8 (SAIL_98_C06/CS804699), cyp78a9 (SALK_066588), amp1-1 (CS8324), and lamp1 (SALK_117012) mutants were obtained from the Arabidopsis Biological Resource Center. All Arabidopsis plants used were in the wild-type Columbia-0 background, except for the cyp78a8 mutant, which was in the Col-3 background. Genotypes were identified by PCR amplification using the primers listed in Supplemental Table S1. Imbibed seeds were vernalized at 4°C for 2 d in the dark. Plants were grown in Jiffy-7 peat pellets under long-day (16L/8D) or short-day (10L/14D) conditions (∼100 μmol photons s−1·m−2) at 22°C. The Arabidopsis cell line T87 was suspension cultured in Jouanneau and Péaud-Lenoël medium under continuous light conditions (∼100 μmol photons s−1·m−2) at 23°C with shaking at 120 r.p.m. (Axelos et al., 1992).
Plasmid construction and transformation
Venus containing a 72-bp linker sequence (Nobusawa et al., 2017) was cloned into the multi-cloning site (MCS) of the pMOE vector (Ito et al., 2010), which is a pUC18-based vector carrying the CaMV 35S promoter (Pro35S), translation enhancer (Ω), MCS, NOS terminator, and attL1/L2 sequences. The resulting Pro35S:Ω:NotI:linker:Venus:terNOS:pUC18 construct was named pPYO_C. The CYP78A5, CYP78A6, CYP78A9, and AMP1 coding sequences were cloned into the NotI site of pPYO_C. For misexpression analysis, 4.7 kb of the CYP78A5 promoter, 4.0 kb of the LAS promoter, and 1.7 kb of the WUS promoter were PCR-amplified and cloned into a modified pENTR vector, which contains an MCS and the NOS terminator (Goldshmidt et al., 2008; Fouracre and Poethig, 2019). Then, Ω:CYP78A5–Venus, Ω:CYP78A6–Venus, and Ω:AMP1–Venus sequences were cloned into the resulting ProCYP78A5:PstI:terNOS:pENTR, ProLAS:PstI:terNOS:pENTR, and ProWUS:PstI:terNOS:pENTR vectors. An ER-marker (SP–ECFP–HDEL) was constructed in the pMOE vector, as described by Mitsuhashi et al. (2000). The primers used for plasmid construction are listed in Supplemental Table S1. For transient gene expression, protoplasts were prepared by treating Arabidopsis T87 suspension-cultured cells with cellulase (Yakult) and macerozyme (Yakult). Plasmid DNA was introduced into the protoplasts via the polyethylene glycol method. To generate transgenic plant lines, the aforementioned vector constructs were introduced into the pGWB601 binary vector using Gateway LR-Clonase (Invitrogen ; Nakamura et al., 2010). Agrobacterium tumefaciens strain EHA-105 was used for transformation of Arabidopsis using the floral-dip method.
Generation of the cyp78a5-12 mutation
We initially constructed Gateway-based vectors by modifying gene cassettes of the pKIR1.0 vector (Tsutsui and Higashiyama, 2017). As an entry vector, an Arabidopsis U6 snRNA 26 promoter:2xBbsI sites:sgRNA:polyT cassette was subcloned from pEN-Chimera into the pENTR vector (Invitrogen; pENTR_AtU6gRNA2; Supplemental Figure S9A; Fauser et al., 2014; Nakajima et al., 2017), whereas as a destination vector, a RPS5A promoter:Cas9:HSP terminator cassette was subcloned into the pGWB601 vector (pGWB601_AtRPS5A-Cas9; Supplemental Figure S9B). To introduce the cyp78a5-12 mutation, a CYP78A5-specific gRNA sequence (5ʹ-ACGGUGCGAAUCCCAUGGCA-3ʹ) was introduced into the BbsI site of the entry vector. The transgene was segregated after induction of the mutation.
Leaf senescence assays
Chlorophyll content was measured using a SPAD-502Plus chlorophyll meter (Konica Minolta); Fv/Fm values were measured using a Junior PAM chlorophyll fluorometer (Walz; Kohzuma et al., 2017). For the dark-induced senescence measurements, leaves emerging on the same day were used for comparison. Detached leaves were placed in 12-well plates and incubated in the dark at 23°C under high humidity conditions.
RNA extraction and quantification analysis
Total RNA was extracted using TRI reagent (Molecular Research Center) as described in the manufacturer’s instructions. Following DNase I (TaKaRa) treatment at 37°C for 30 min, total RNA was further purified using the acidic phenol/chloroform method. First-strand cDNA was synthesized from 500 ng total RNA using ReverTra Ace qPCR RT Master Mix (TOYOBO) according to the manufacturer’s instructions. Quantification analysis was performed using a KAPA SYBR FAST qPCR Kit (KAPA Biosystems) and a Rotor-Gene Q 2PLEX thermocycler (Qiagen). The primers used for the quantification analyses are listed in Supplemental Table S1.
Microscope observations
For the detection of Venus fluorescence in SAs, plants were sliced manually using a razor. Sections were immediately observed under a confocal laser scanning microscope (LSM710; Carl Zeiss) equipped with a Plan-Apochromat ×20/0.8 lens (Carl Zeiss). Emissions of Venus and autofluorescence were detected using SP640 and LP640 filters (Carl Zeiss), respectively. A 488-nm argon laser was used for excitation at 2% power output. Pinhole was set to 1 Airy Unit. Gain settings to detect emissions from fluorescent proteins were ˂750. To observe subcellular localization, we used an LSM710 microscope fitted with a Plan-Apochromat ×63/1.40 lens (Carl Zeiss). Emissions of ECFP, Venus, and chlorophyll autofluorescence were detected using BP490–555, SP490, and LP615 filters, respectively. Acquired images were processed using ZEN software (Carl Zeiss). Rosette diameters were measured from photographic images using the NIH ImageJ software.
Statistical analyses
Statistical analyses were carried out using R software (https://www.r-project.org). Statistical methods used for comparison and significance criteria were described in the figure legends.
Accession numbers
The sequence data used in this study were as follows: CYP78A5 (AT1G13710), CYP78A6 (AT2G46660), CYP78A7 (AT5G09970), CYP78A8 (AT1G01190), CYP78A9 (AT3G61880), CYP78A10 (AT1G74110), AMP1 (AT3G54720), LAMP1 (AT5G19740), SGR1 (AT4G22920), ACT8 (AT1G49240), LAS (AT1G55580), and WUS (AT2G17950).
Supplemental data
The following materials are available in the online version of this article.
Supplemental Figure S1 Plastochron length of the CYP78A isoform mutants.
Supplemental Figure S2 Expression of CYP78A isoform genes during dark-induced senescence.
Supplemental Figure S3 Expression levels of CYP78A isoforms, AMP1, and LAMP1 in the shoot apex domains.
Supplemental Figure S4 Confocal microscopic images of the shoot apex of two independent cyp78a5 plants harboring a CYP78A5 promoter-driven CYP78A6–Venus transgene.
Supplemental Figure S5 Subcellular localization of CYP78A isoforms.
Supplemental Figure S6 CYP78A5 can regulate the plastochron and leaf size in a non-cell-autonomous manner.
Supplemental Figure S7 Phenotypic comparison of the cyp78a5 a7 and amp1 lamp1 double mutants.
Supplemental Figure S8 AMP1 can regulate the plastochron and leaf size in a non-cell-autonomous manner.
Supplemental Figure S9 Construction of Gateway-based CRISPR/Cas9 vectors.
Supplemental Table S1 Primers used in this study.
Acknowledgments
We thank T. Nakagawa for providing the pGWB601 vector, T. Higashiyama for the pKIR1.0 vector, M. Endo for the pEN-Chimera, Y. Takahashi for the pMOE vector, and Y. Nagashima, Y. Honbo, and Y. Kobuke for technical assistance. The Arabidopsis T87 cell line was provided by RIKEN BRC through the National Bio-Resource Project of MEXT, Japan.
Funding
This research was supported by Research Fellowships from the Graduate School of Integrated Sciences for Life, Hiroshima University, to TN, and grants from the Project of the NARO Bio-oriented Technology Research Advancement Institution (Research Program on Development of Innovative Technology) to M.K.
Conflict of interest statement. None declared.
T.N. and M.K. conceived and designed the research; T.N., M.K., H.U., and H.Y. performed the experiments; and T.N. and M.K. wrote the manuscript.
The author responsible for distribution of materials integral to the findings presented in this article in accordance with the policy described in the Instructions for Authors (https://dbpia.nl.go.kr/plphys/pages/general-instructions) is: Makoto Kusaba ([email protected]).
References
Author notes
Senior author.