-
PDF
- Split View
-
Views
-
Cite
Cite
Jelena Cvetkovic, Ilka Haferkamp, Regina Rode, Isabel Keller, Benjamin Pommerrenig, Oliver Trentmann, Jacqueline Altensell, Michaela Fischer-Stettler, Simona Eicke, Samuel C Zeeman, H Ekkehard Neuhaus, Ectopic maltase alleviates dwarf phenotype and improves plant frost tolerance of maltose transporter mutants, Plant Physiology, Volume 186, Issue 1, May 2021, Pages 315–329, https://doi.org/10.1093/plphys/kiab082
- Share Icon Share
Abstract
Maltose, the major product of starch breakdown in Arabidopsis (Arabidopsis thaliana) leaves, exits the chloroplast via the maltose exporter1 MEX1. Consequently, mex1 loss-of-function plants exhibit substantial maltose accumulation, a starch-excess phenotype and a specific chlorotic phenotype during leaf development. Here, we investigated whether the introduction of an alternative metabolic route could suppress the marked developmental defects typical for mex1 loss-of-function mutants. To this end, we ectopically expressed in mex1 chloroplasts a functional maltase (MAL) from baker’s yeast (Saccharomyces cerevisiae, chloroplastidial MAL [cpMAL] mutants). Remarkably, the stromal MAL activity substantially alleviates most phenotypic peculiarities typical for mex1 plants. However, the cpMAL lines contained only slightly less maltose than parental mex1 plants and their starch levels were, surprisingly, even higher. These findings point to a threshold level of maltose responsible for the marked developmental defects in mex1. While growth and flowering time were only slightly retarded, cpMAL lines exhibited a substantially improved frost tolerance, when compared to wild-types. In summary, these results demonstrate the possibility to bypass the MEX1 transporter, allow us to differentiate between possible starch-excess and maltose-excess responses, and demonstrate that stromal maltose accumulation prevents frost defects. The latter insight may be instrumental for the development of crop plants with improved frost tolerance.
Introduction
The glucose-based water insoluble polymers starch and cellulose represent, besides soluble sugars, the major carbohydrate forms in vascular plants. Starch is comprised linear amylose and branched amylopectin that form a layered, semi-crystalline structure and is deposited in plastids, such as chloroplasts and amyloplasts, as insoluble granules. When photosynthesis is absent, starch serves as a carbohydrate and energy store, and provides the required metabolic precursors to fuel a wide range of cellular processes. By this, starch breakdown guarantees the maintenance of leaf mesophyll cell functions during the night, as well as continuity of carbohydrate provision to sink organs (Zeeman et al., 2010; Smith and Zeeman, 2020).
The discovery and analysis of various starch excess Arabidopsis (Arabidopsis thaliana) mutants (SEX lines) unraveled the complex mechanism of starch mobilization (Stitt and Zeeman, 2012). Initiation of starch breakdown was shown to require phosphorylation of the granule and two enzymes, namely glucan water dikinase and the phosphoglucan water dikinase, catalyze the corresponding reactions (Ritte et al., 2002; Baunsgaard et al., 2005; Kötting et al., 2005; Fettke et al., 2007). Phosphate addition leads to hydration of the granule surface, disrupts the structure of amylopectin, and exposes linear chains for subsequent hydrolysis (Hejazi et al., 2009). The cleavage by different amylases (β-amylase1, β-amylase3, α-amylase3, and isoamylase3) releases maltose, malto-oligosaccharides, and phospho-oligosaccharides (Fulton et al., 2008; Streb et al., 2012), and removal of the phosphate groups from starch and malto-oligosaccharides by the phosphoglucan phosphatases SEX4 and LSF2 allows for progression of hydrolysis (Kötting et al., 2009; Santelia et al., 2011; Silver et al., 2014). Moreover, the condensation of short-chain maltodextrins by the disproportionation enzyme 1 (DPE1) releases one glucose residue and leads, in addition, to the elongation of the glucan to be of sufficient length to act again as substrate for amylases (Critchley et al., 2001). Thus, iterative rounds of phosphorylation, dephosphorylation, and hydrolysis accomplish the degradation of transitory starch during the night. For completeness, we note that other enzymes can also contribute to starch breakdown, such as the debranching enzyme, limit dextrinase, the plastidial α-glucan phosphorylase PHS1 (Delatte et al., 2006; Malinova et al., 2014), and further regulatory and ancillary proteins (Fulton et al., 2008; Schreier et al., 2019; Abt and Zeeman, 2020).
To connect initial starch breakdown in the stroma with final conversion processes in the cytosol, the hydrolytic products have to leave to chloroplast. Separate transporters exist for the export of maltose and glucose from the chloroplast, and biochemical studies suggested that maltose is the major exported product (Weise et al., 2004). This assumption was corroborated by the analysis of mutants lacking corresponding transport proteins. The loss of the glucose transporter pGlcT did not disturb diurnal starch turnover (Weber et al., 2000; Cho et al., 2011), whereas absence of the maltose transporter MEX1 caused a marked accumulation of maltose, increased starch levels, and a dwarf, chlorotic mutant phenotype (Niittylä et al., 2004). This mex1 phenotype was exacerbated by the additional loss of pGlcT (Cho et al., 2011) indicating both, that maltose represents the major hydrolytic product of starch degradation, and that the export of all breakdown products is of high importance for plant growth and development.
Latter conclusion gained independent support by analyses of mutants lacking the cytosolic transglucosidase DPE2 (Chia et al., 2004; Lu and Sharkey, 2004). DPE2 uses the maltose exported via MEX1 for the nocturnal synthesis of a cytosolic soluble heteroglycan (SHG; Fettke et al., 2006). The loss of DPE2 activity has some similar consequences to the loss of MEX1 since both, dpe2 and mex1 mutants accumulate very high levels of maltose and exhibit a starch-excess phenotype (Chia et al., 2004; Niittylä et al., 2004). Interestingly, maltose levels in dpe2 are even higher than in mex1 mutants and this sugar is distributed between the cytosol and the chloroplast lumen, rather than being confined to the stroma (Lu et al., 2006a, 2006b). However, dpe2 plants are not chlorotic or as severely impaired in growth as mex1. Glucose added to SHG by DPE2 can subsequently be remobilized by the cytosolic α-glucan phosphorylase PHO2 (Fettke et al., 2004; Lu et al., 2006a, 2006b), which releases the terminal glucose from this polymer through phosphorolysis, liberating glucose-1-phosphate which enters further anabolic or catabolic pathways.
The transport protein MEX1 is a central player in starch mobilization since it links corresponding stromal to subsequent cytosolic enzyme reactions. The MEX1 protein lacks structural homology to other sugar transporters from pro and eukaryotes, and the corresponding gene was found in a cyanobacterial subgroup, in green algae and all plants (Niittylä et al., 2004; Reidel et al., 2008; Ryoo et al., 2013; Findinier et al., 2017). Since MEX1 loss-of-function mutants are characterized to show impaired starch mobilization, dwarf growth, and early chloroplast degradation (Niittylä et al., 2004; Stettler et al., 2009), we wondered whether an alternative route for carbon release during starch turnover could replace MEX1, and if so, what the consequences are. Accordingly, we hypothesized that stromal maltose cleavage into glucose and its subsequent export via glucose transport proteins might allow MEX1 function to be bypassed.
To this end, we expressed a cytosolic maltase (MAL) from baker’s yeast ectopically in the stroma of mex1 mutants (Niittylä et al., 2004). Although this strategy did not prevent maltose accumulation completely in the corresponding chloroplastidial MAL (cpMAL) lines, it decreased the levels of this sugar at least by half when compared to mex1. Remarkably, the cpMAL lines exhibited even higher starch levels than mex1 but lacked many phenotypic peculiarities typical for mex1. The characteristics of the cpMAL lines are valuable since they allow us to dissect possible effects caused by maltose accumulation from those caused by excessive starch, they shed further light on starch homeostasis, and provide more information on maltose metabolism and maltose functions. This is of particular interest since it has been shown that chloroplast sugar homeostasis affects fundamental plant properties, like photosynthetic performance after cold exposure, freezing-induced electrolyte release, induction of flowering, or final seed yield (Knaupp et al., 2011; Patzke et al., 2019; Trentmann et al., 2020).
Results
The N-terminal domain of NTT1 targets yeast MAL to the chloroplast
Absence of maltose transport activity in the inner envelope membrane hinders maltose release from the chloroplast. Consequently, mex1 mutants accumulate substantial amounts of this disaccharide in the stroma. The excessive stromal maltose results in multiple physiological and developmental defects of the mex1 mutants, such as inhibition of starch breakdown, chloroplast degradation, a lower chlorophylla/b ratio as well as stunted plant growth (Niittylä et al., 2004; Stettler et al., 2009; Purdy et al., 2013).
Yeast cells efficiently hydrolyze maltose into two glucose moieties by an enzyme named MAL, acting as α-glucosidase. Accordingly, we hypothesized that the presence of the baker’s yeast MAL32 (Dietvorst et al., 2007) in chloroplasts of mex1 mutants might prevent maltose accumulation and the resulting responses. However, since yeast α-glucosidases are cytosolic, we considered it unlikely that MAL32 would enter the chloroplast when ectopically expressed in plants. Therefore, we generated a hybrid construct consisting of MAL32 fused to the N-terminus of the plastid located nucleotide transporter NTT1 from Arabidopsis (Neuhaus et al., 1997). This NTT1 N-terminus comprised the first 86 amino acids, which is known to function as a plastid targeting sequence (Rolland et al., 2016).
Transient expression of the corresponding GFP fusion construct in Arabidopsis protoplasts revealed that the green fluorescence of the C-terminally attached GFP labels the chloroplast and accumulates in close proximity to the auto-fluorescence of chlorophyll (Figure 1, A–D). This fluorescence pattern is typical for soluble GFP-labeled proteins in the chloroplast stroma. Thus, the N-terminal domain of NTT1 targets the GFP-tagged yeast MAL into the chloroplast.
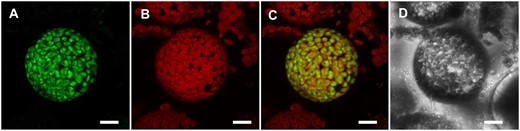
The NTT1 N-terminus targets yeast MAL to the chloroplast. Transient expression of the hybrid construct with C-terminally fused GFP in Arabidopsis protoplasts. The GFP fluorescence accumulates in close proximity to the chlorophyll autofluorescence, which is indicative for a stromal localization. (A) GFP fluorescence. (B) chlorophyll autofluorescence. (C) merge of A and B. (D) greyscale. Scale bars represent 10 µm.
In the following studies, we used the NTT1:MAL32 hybrid protein without the marker to avoid possible effects of the large GFP on protein function. The corresponding protein is abbreviated cpMAL for chloroplastidial MAL.
mex1 mutants gain MAL activity by ectopic expression of the cpMAL gene
To analyze whether it is possible to synthesize a yeast MAL functionally in chloroplasts, the cpMAL construct was used for stable transformation of the mex1 mutant (Niittylä et al., 2004). In total, we screened 24 independent transformants for presence of the recombinant cpMAL sequence using RT-qPCR. The two expressor lines cpMAL9:mex1 (below named cpMAL9) and cpMAL16:mex1 (below named cpMAL16) exhibited highest relative cpMAL transcript levels (Figure 2A; please note: both, cpMAL9 and 16 plants analyzed were homozygous for the transgene as confirmed by BASTA resistance of all seedlings). An enzyme activity assay confirmed the presence of active MAL in extracts from cpMAL9 or cpMAL16 leaves, while corresponding enzyme activity is absent in mex1 plants (Figure 2B).
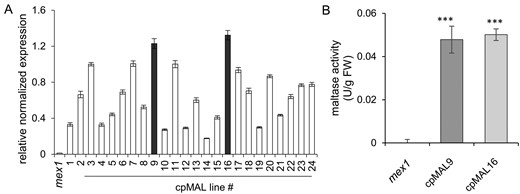
Identification of cpMAL expressing lines and determination of MAL activity. (A) cpMAL mRNA accumulation in mex1 (control) and 24 individual cpMAL expressing lines. (B) MAL activity in extracts prepared from mex1 and the cpMAL lines 9 and 16, respectively (given in units, U = 1 µmole/min). Three technical replicates were used for RT-qPCR; expression levels are given as ct-values normalized to the two chosen reference genes. Five biological replicates were used for the enzyme activity test. Standard errors are displayed and significance of differences between MAL activity in mex1 and in the two selected cpMAL lines were analyzed by student t test: ***P-value <0.001.
cpMAL activity in chloroplasts alleviates the mex1 growth defect
Blockage of maltose export out of chloroplasts causes several secondary effects, including considerable growth retardation and chlorosis (Niittylä et al., 2004; Stettler et al., 2009). Therefore, we initially documented the development of cpMAL9 and cpMAL16, and compared it with that of mex1 mutants and wild-type plants.
All plant lines germinated more or less simultaneously. However, 2 weeks after germination, seedlings of the wild-type and the cpMAL lines were slightly, but significantly larger than mex1 seedlings (Supplemental Figure S1A–D). These phenotypic differences became more obvious during further development. The mean rosettes diameter of 4-week-old mex1 mutants was 1.3 cm, whereas cpMAL9, cpMAL16, and wild-type plants were much larger (mean diameters of 2.9, 2.6, and 3.6 cm, respectively; Figure 3B). Moreover, the cpMAL lines developed further than mex1, forming more leaves (12 leaves rather than 8 in mex1) and almost reaching the developmental stage of the wild-type (14 leaves, Figure 3C). The mean rosette fresh weights of cpMAL9 and cpMAL16 plants (34–42 mg, respectively) were intermediate between wild-type (73 mg) and mex1 (7 mg) plants (Figure 3D). Six weeks after germination, the cpMAL plants were still larger than mex1 and approached the size of the wild-type in terms of rosette diameter and leaf number (∼90% of the wild-type size, Supplemental Figure S2, A–C), but were considerably lighter (Supplemental Figure S2D). As a control, we also expressed the cpMAL gene in Arabidopsis Col-0, but did not observe any phenotypic change when compared to wild-type plants (Supplemental Figure S3A and B). In summary, these data demonstrate that introduction of a plastid located MAL activity alleviates the growth defect of mex1 and restores, to a large extent, wild-type rosette development and appearance.
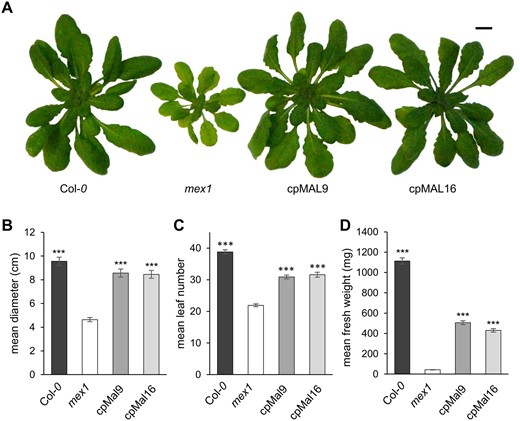
Plastidic MAL activity alleviates the dwarf phenotype of 4 weeks old mex1 mutants. (A) Rosette phenotype of representative WT (Col-0), mex1 and cpMAL overexpressor plants (cpMAL9 and cpMAL16). Mean rosette diameter (B), leaf number (C) and fresh weight (D) of 20 plants per line. Standard errors are given. Rosettes have been digitally extracted for comparison, scale bar represents 1 cm. Significance of differences between the growth of mex1 and the remaining plant lines was analyzed by Student t test: ***P-value <0.001.
Heterologous expression of cpMAL in mex1 prevents chloroplast damage and changes in mesophyll cell architecture
An impressive consequence of blocked maltose export in mex1 plants is the disruption of chloroplast integrity (Stettler et al., 2009), accompanied by a decreased chlorophylla/b ratio and impaired photosynthetic capacity (Cho et al., 2011; Purdy et al., 2013). Leaf color of the cpMAL lines clearly differed from that of mex1 and rather resembled that of wild-type plants (Figure 3A; Supplemental Figure S2A). The same holds true for the chlorophylla/b ratio (Supplemental Figure S4) and the photosynthetic parameters measured. The chlorophylla/b ratio of mex1 leaves was ∼30% lower than that of wild-type leaves, while leaves of the two cpMAL lines showed a comparatively slight decrease (by 5% in cpMAL9 and 14% in cpMAL16, respectively, Supplemental Figure S4). Moreover, mex1 rosettes exhibited 66% of the maximum photosynthetic quantum yield of wild-type leaves, while leaves of the both cpMAL lines reached ∼92% (Figure 4A and B). The observed decreases in maximum quantum yield were accompanied by increases in non-photochemical quenching (NPQ, Figure 4C). Thus, the two cpMAL lines were closer to wild-types than to mex1 mutants in terms of both the photosynthetic efficiency, reflected by the Fv/Fm ratio (Figure 4A and B), and the dissipation of excess light energy by NPQ (Figure 4C).
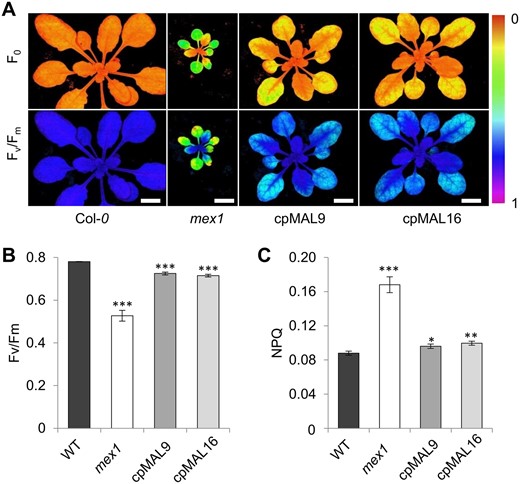
Plastidic MAL activity alleviates photosynthetic defects of the mex1 mutant. (A) representative images of minimum fluorescence (F0) and maximum quantum yield (Fv/Fm) of rosette leaves from Arabidopsis wild-type (Col-0), mex1, and cpMAL lines 9 and 16. (B) values of the photosynthetic parameter Fv/Fm. (C) values of the photosynthetic parameter non-photosynthetic quenching (NPQ). Plants were grown for 4 weeks under standard light intensity (125 µmol photons m−2s−1). Data represent the mean values of ten leaves from at least six biological replicates. Standard errors are given. Scale bars represent 1 cm. Statistical analysis of differences between wild-type and mutant levels was performed with one-tailed t test: *P ≤ 0.05; **P ≤ 0.01 and ***P ≤ 0.001.
Further typical characteristics of mex1 mutants include their altered leaf architecture, with smaller mesophyll cells and thinner leaves, and the occurrence of aberrant swollen chloroplasts (Stettler et al., 2009). To check whether MAL activity in mex1 plastids can also alleviate these features, we examined the cpMAL leaves by light and electron microscopy. Leaves of mex1 plants were thinner than those of the two cpMAL lines, which were as thick as wild-type leaves (Supplemental Figure S5). Interestingly, compared to wild-type, sections of the mesophyll cells of mex1 and the cpMAL lines appeared to have fewer chloroplasts which, in addition, had a swollen, roundish appearance (Supplemental Figures S5–S7). Thus, while cpMAL leaves resembled the wild-type in terms of leaf thickness (Supplemental Figure S5), in terms of chloroplast number and organelle shape they still had similarities with mex1 (Supplemental Figures S6, S7). The images also revealed that the chloroplasts of the cpMAL lines contained many starch granules that were larger than those in the wild-type.
Heterologous expression of cpMAL alters sugar metabolism of mex1 mutants
The fact that the cpMAL lines were much larger than mex1 plants (Figure 3; Supplemental Figures S1, S2) suggests that the recombinant MAL efficiently cleaves maltose and, by this, prevents its accumulation in the stroma, which finally alleviates negative effects on chloroplast integrity and plant growth. To test this hypothesis, we determined the carbohydrate levels of rosettes from the individual plant lines during the entire diel cycle.
As reported previously (Niittylä et al., 2004), mex1 exhibits constantly elevated levels of maltose (Figure 5A). By contrast, leaves of cpMAL9 and cpMAL16 plants accumulate less maltose when compared to the mother plant mex1 (Figure 5A). However, to our surprise, this decrease was only by ∼40% on average and consequently the cpMAL expressor lines still contained ∼10– (dusk) to 100 times (dawn) more maltose than present in corresponding wild-type leaves (Figure 5A). The measurable daytime decrease of maltose demonstrates that cpMAL is active in leaves from cpMAL9 and cpMAL16 plants, although its activity did not decrease maltose to the low levels seen in wild-types (Figure 5A).

Diurnal levels of selected carbohydrates in wild-type, mex1, and cpMAL plants. (A) maltose levels; (B) starch levels; (C) glucose levels; (D) fructose levels; (E) sucrose levels. Plants were grown for 4 weeks under standard light intensity (125 µmol photons m−2s−1). Each data point represents mean values of ten leaves taken from at least four biological replicated plants. Standard errors are given.
Next, we investigated whether the observed decrease in maltose was sufficient to reactivate wild-type starch turnover in the cpMAL expressor plants. Surprisingly, the overall starch content of cpMAL9 and cpMAL16 plants was even higher than in mex1 (Figure 5B). Analysis of the data revealed that cpMAL9 and cpMAL16 accumulated more starch during the light phase than mex1, but still slightly less than wild-type plants. Wild-type leaves incorporated 53 µmol glucose g/Fw into starch, whereas the two cpMAL expressor lines incorporated ∼45 µmol glucose g/Fw, and mex1 only 32 µmol glucose g/Fw (Figure 5B). While wild-type and mex1 plants released 52 and 28 µmol glucose g/Fw, respectively, from their starch reserves, cpMAL9 and cpMAL16 degraded starch corresponding to ∼40 µmol glucose g/Fw (Figure 5B). Thus, the amount of nocturnal starch degradation in both cpMAL lines was in-between the corresponding rates observed in wild-type and mex1 plants. Therefore, although the expression of cpMAL reactivates starch turnover, this process does not fully reach the turnover rates present in wild-types. The combination of a stimulated starch synthesis and the slightly increased starch breakdown, when compared to mex1 mutants (Figure 5B), explains why cpMAL9 and cpMAL16 accumulate even more starch over time than mex1.
Next, we investigated whether reactivation of starch turnover in cpMAL9 and cpMAL16 affects the levels of glucose, fructose, or sucrose. In the light, the glucose contents of cpMAL9 and cpMAL16 leaves were lower than in mex1, but higher than in the wild-type (Figure 5C). In all lines, the amount of glucose increased after dawn and reached a maximum after 4 h of illumination. The increase and maximal glucose level was higher in mex1 and the two cpMAL lines than in the wild-type (Figure 5C). During the next 4 h, glucose in cpMAL9 and cpMAL16 dropped to almost wild-type levels, whereas in mex1 glucose declined more slowly (Figure 5C). During the night, glucose levels continued to drop but remained higher in mex1 plants than in the other lines. Fructose also reached maximal levels after 4 h of illumination (Figure 5D). As for glucose, fructose was higher in mex1 and the cpMAL lines than in the wild-type. Fructose levels declined during the latter part of the day and the subsequent night, converging soon after dusk (Figure 5D). After dawn, all plant lines showed a rapid increase in sucrose, which approached maximum values of ∼1.5 µmol g/Fw after 2 h of illumination (Figure 5E). Between 4 and 8 h of light, the sucrose level of the wild-type and mex1 plants remained quite constantly high, while it decreased in both cpMAL lines leading to less sucrose at the end of the light phase, when compared to the other two plants lines (Figure 5E). After dusk, the sucrose levels of mex1, cpMAL9, and cpMAL16 dropped within 2 h to minimum values and remained substantially lower than in wild-type plants throughout the night (Figure 5E).
Delayed inflorescence development in mex1 is rescued by expression of cpMAL
Various Arabidopsis mutants with defects in starch catabolism (e.g. sex1, sex4, and bam3 plants) exhibit an extended, photoperiod-insensitive vegetative phase (Matsoukas et al., 2013). Thus, impaired starch turnover of starch-excess mutants and the resulting limitation in nocturnal carbohydrate availability were proposed to cause the delay in transition from the vegetative to the reproductive phase. Since expression of cpMAL reactivated starch turnover compared to the mex1 parental line (Figure 5B) and restored the vegetative growth to almost wild-type levels (Figure 3; Supplemental Figures S1 and S2), we were also interested to analyze the photoperiod-sensitive development of the reproductive tissues.
We transferred 4 weeks old plants from short- to long-day (LD) conditions and measured the resulting inflorescence lengths (Figure 6). Twenty-three days after transfer, 25% of the wild-type, 17% of cpMAL9, and 4% of cpMAL16 plants, respectively, had bolted (inflorescence length of at least 1 cm; see inset Figure 6). After 26 d, all wild-type plants had bolted, while all cpMAL expressing plants had bolted by Day 27. In contrast, at this time point, only the first few mex1 mutants (5%) had started bolting, and only by 35 d after transfer had all mex1 plants bolted. Thus, mex1 bolted more than a week later than wild-types and cpMAL mutants (Figure 6), demonstrating that expression of cpMAL alleviates the mex1 phenotype, although not fully restoring the wild-type flowering properties.

Analysis of inflorescence development of wild-type and mutant plants. Determination of differences in the onset of bolting. Plants were cultivated for 4 weeks under standard conditions (120 µE, 10 h light/14 h dark) and transferred to LD conditions (250 µE, 16 h light/8 h dark) for flowering induction. When its shoot length reached 1 cm, the corresponding plant was defined as bolted. Horizontal arrows indicate when plants reached 100% bolting, vertical arrows indicate day of harvest. Data represent the mean ± SEM of three replicates with ≥8 plants each.
Elevated plastidic maltose affects plants responses to cold temperature
There is ample evidence that defects in sugar and starch metabolism result in an impaired ability of the plant to adapt to cold and to resist frost periods (Kaplan and Guy, 2005; Kaplan et al., 2006; Pommerrenig et al., 2018). To check whether expression of a plastid-located MAL in mex1 plants modifies the frost tolerance of corresponding mutants, we conducted freezing recovery analyses (Trentmann et al., 2020). To confirm that our experimental setup provides reliable data, we included dpe2–5 mutants in the analysis. Latter plants lack the cytosolic transglucosidase DPE2 and are known to exhibit increased frost tolerance (Li et al., 2011).
Indeed, after retransfer from freezing (−10°C) to ambient conditions, all dpe2–5 mutants recovered well and continued growing whereas only 40% of the wild-type plants survived this stress stimulus (Figure 7A and B). One might expect that the dwarf growth of mex1, its developmental defects (Figure 3; Supplemental Figures S2, 5B and 6B) and particularly its lower photosynthetic capacity (Figure 4) might cause increased freezing sensitivity when compared to wild-type plants. However, the freezing recovery rate of mex1 was 40%, which is identical to that of wild-type plants (Figure 7A and B). This observation indicates that mex1, despite its various biochemical and developmental defects, can cope well with such cold temperature. Interestingly, both cpMAL lines exhibited an improved ability to survive freezing, since 60% of these mutant plants recover from the freezing stress stimulus (Figure 7A and B).
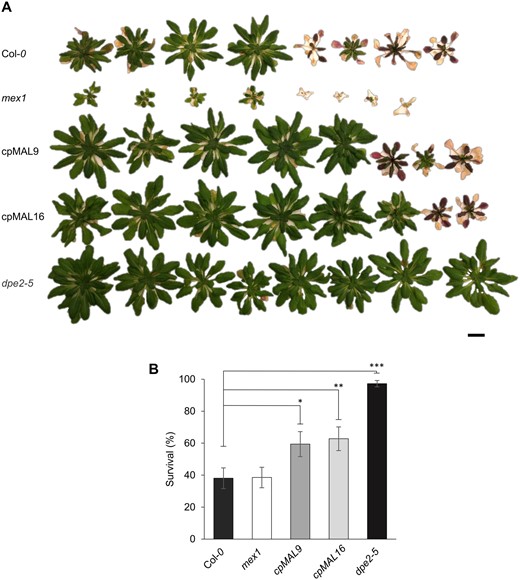
Analysis of the freezing tolerance of wild-type and mutant plants. (A) Representative picture of a set of wild-type and mutant plants after 3 weeks’ recovery from −10°C freezing. (B) quantification of the survival rate. Scale bar represents 1 cm. Statistics: n = 12 sets (of at least eight plants per line), data represent the mean ± SEM: *P ≤ 0.05; **P ≤ 0.01 and ***P ≤ 0.001, estimated by student’s t test.
Discussion
Nocturnal starch mobilization in leaf chloroplasts provides the surrounding cell and accordingly sink tissues with carbon and energy. The transporter MEX1 in the inner plastid envelope fulfills a central role in this process since it delivers maltose, the major starch degradation product, to the cytosol (Niittylä et al., 2004; Purdy et al., 2013; Supplemental Figure S8A). Missing MEX1 activity traps maltose in the chloroplast and by this hinders its feeding into cytosolic carbohydrate metabolism. As a result, the corresponding mex1 mutants contain high levels of maltose in their chloroplasts and accumulate starch due to its impaired diurnal turnover (Niittylä et al., 2004; Purdy et al., 2013), Supplemental Figure S8B).
Just like other starch-excess mutants, mex1 plants exhibit stunted growth and developmental defects (Matsoukas et al., 2013). Interestingly, mex1 leaves become chlorotic during maturation (Niittylä et al., 2004; Stettler et al., 2009; Purdy et al., 2013). This mex1 characteristic could be attributed to a stimulated degradation of chloroplasts, which substantially decreases their number in mesophyll cells (Niittylä et al., 2004; Stettler et al., 2009; Purdy et al., 2013). It is not completely clarified which metabolic perturbations are causative for the individual phenotypes of mex1 plants. The accumulation of maltose was proposed to lead to malfunction of the chloroplast, which finally triggers its degradation (Niittylä et al., 2004; Stettler et al., 2009; Purdy et al., 2013).
Here, we show that ectopic expression of a yeast MAL in chloroplasts can compensate to some extent for the missing MEX1 function in mex1. We confirmed that the usually cytosolic yeast MAL could be successfully targeted to the chloroplast stroma by extending its coding sequence with the 83 amino-acid transit peptide of the ATP/ADP transporter NTT1 (Figure 1). Our data also show that the yeast MAL is functional in the chloroplasts of the resulting cpMAL lines. Enzymatic tests in plant extracts from mex1 plants failed to cleave maltose whereas MAL activity was readily detectable in extracts of the two representative cpMAL lines 9 and 16 (Figure 2B). Furthermore, cpMAL expression substantially reduced the maltose accumulation when compared to mex1 (Figure 5A). However, it is important to note that the cpMAL lines still contained ∼50% of the maltose present in mex1. Therefore, cpMAL activity could only reduce but not prevent maltose accumulation. At first glance, the comparatively moderate decrease of the maltose level appears remarkable (Figure 5A). However, it is in accordance with the biochemical properties of the yeast MAL. The affinities of all baker’s yeast MAL isoforms for its substrate are generally very low (Km of ∼100 mM; Tabata et al., 1984) and thus are likely insufficient to allow for maximal maltose cleavage in the cpMAL lines. A direct comparison between the activity of the recombinant MAL in the two cpMAL lines and stimulated carbon flux of starch degradation was not possible, since neither the exact substrate (maltose) concentration in the stroma, nor the pH optimum of the enzyme or a possible interaction of the recombinant protein with other stromal sugars and sugar polymers can be approximated.
When compared to wild-type plants, the two cpMAL lines show a slower rate of starch degradation, accompanied by increasing maltose levels and a fast depletion of nocturnal sucrose (Figure 5). These observations suggest that there is insufficient maltose cleavage, and that this still limits starch degradation and the provision of cytosolic processes with carbohydrates (Supplemental Figure S8C). Moreover, although MAL hydrolyses maltose to two molecules of glucose, the glucose levels of the cpMAL expressor lines were always lower when compared to mex1 (Figure 5C). We nevertheless assume that glucose generated via MAL in the stroma is exported to the cytosol where it is metabolized rapidly. This might not be easy to detect since glucose can also arise from the turnover of sucrose. Indeed, the similarity in the diel profiles of glucose and fructose suggest that these sugars derive from sucrose hydrolysis, presumably in the vacuole (Vu et al., 2020).
Generally, glucose can leave the chloroplast via either of two distinct transport proteins, namely pGlcT and pSuT (Weber et al., 2000; Patzke et al., 2019), or after phosphorylation by the plastidic hexokinase (HxK3) as glucose 6-phosphate (G6P), via the G6P/Pi antiporter GPT (Kammerer et al., 1998). However, since the activities of GPT and the plastidic nucleotide transporter NTT, which is required for nocturnal ATP supply in chloroplasts (Reinhold et al., 2007), are quite low (Kampfenkel et al., 1995; Quick et al., 1995; Reiser et al., 2004) we assume that glucose export in cpMAL plants most likely takes place via the transporters pGlcT and/or pSuT.
The loss of MEX1 results in a dwarf morphology, pale leaves, and early disintegration of chloroplast architecture (Niittylä et al., 2004; Stettler et al., 2009; Purdy et al., 2013). Slow growth is a feature typical for several starch-excess mutants (Streb and Zeeman, 2012) but is usually less pronounced in Arabidopsis plants with only slightly increased starch levels (Baunsgaard et al., 2005). Our observations suggest that the dwarf morphology of mex1 does not result solely from excessive starch. The fact that cpMAL plants almost reach wild-type growth (Figure 3A and B; Supplemental Figure S2A and B) but contain even more starch than mex1 (Figure 5B; Supplemental Figure S7A–D) indicates that starch can accumulate to much higher levels without triggering dwarfism like that of mex1. Rather, the dwarf growth of mex1 mutants corresponds with its low chlorophyll-containing pale leaves (Purdy et al., 2013) due to an early onset of chloroplast disintegration (Stettler et al., 2009).
A detailed comparison of these phenotypic properties in starch-excess- and starch-free mutants led to the conclusion that accumulation of excess maltose, or malto-oligosaccharides, is causative for early onset of chloroplast disintegration on the levels of membrane architecture and biochemistry (Stettler et al., 2009). In this context, it is important to mention that both cpMAL lines, although looking similar to the wild-type (Figsures 5B, 3), exhibit only a modest reduction in maltose compared to mex1 leaves and having very high starch levels (Figure 5A). Both cpMAL lines contain maltose concentrations exceeding wild-type levels up to 100-fold (e.g. directly after onset of light, Figure 5B). This argues against the assumption that any increase in stromal maltose levels are deleterious and causative for the type of defects seen in mex1 (Stettler et al., 2009). Rather, it indicates that the Arabidopsis can tolerate a certain degree of maltose accumulation easily. Indeed, it was previously demonstrated that maltose concentrations can transiently increase manifold in wild-type plants in response to cold stress (Kaplan and Guy, 2004).
Our data imply that stromal maltose has to exceed a certain threshold concentration to induce the defects that are present in mex1 but absent in cpMAL. Interestingly, there are several examples of where developmental processes in vascular plants are dependent upon reaching threshold concentrations of certain intracellular signals, including sugars (Garcia et al., 2003; Yang et al., 2013; Kurepa et al., 2014; Vaddepalli et al., 2019). In fact, maltose itself was already been shown to act as signal inducing responses specific for this type of sugar (Teng et al., 2005) and at least in case of the green micro-algae Chlamydomonas reinhardtii there is solid evidence that MEX1 itself is involved in sensing and transmitting a maltose signal out of the chloroplast (Findinier et al., 2017). In this respect, it appears worth to mention that Arabidopsis contain, in addition to the well-analyzed cytosolic SNF1-related protein kinase 1 (SnRK1) complex, all corresponding SnRK1 subunits in the chloroplast (Avila-Castañeda et al., 2014). This chloroplast located SnRK1 complex associates spatially with starch and its reguatory subunits are, moreover, able to bind maltose (Ruiz-Gayosso et al., 2018). Accordingly, Arabidopsis chloroplasts contain a signaling machinery which might generally allow the preception and transduction of a maltose-derived signal. Thus, since it was proposed that in mex1 mutants there is a maltose-dependent stress or signal from the chloroplast to the nucleus triggering chloroplast degradation or lysis (Stettler et al., 2009), we speculate that the decrease in maltose in cpMAL lines is sufficient to take it below the threshold concentration that elicit the developmental aberrations.
It was surprising to see, that throughout the diel cycle the two cpMAL lines contain starch levels markedly exceeding even the starch contents present in mex1 mutants (Figure 5B). We assume that the accumulation of such high starch levels is possible because, unlike in mex1, the cpMAL plants are unimpaired in their photosynthetic performance (Figure 4A–C; Supplemental Figure S8C). The photosynthetic parameters like maximum quantum yield, chlorophylla/b ratios, non-photosynthetic quenching as well as the overall leaf architecture of the cpMAL plants were much more similar to wild-type plants than to the mex1 parental line (Figure 4; Supplemental Figures S4–S6).
The similarities in the appearance of the chloroplasts from cpMAL lines to those of mex1 (Supplemental Figures S6, S7) might result from both, the high starch and the high maltose present. The cpMAL chloroplasts are nevertheless fully functional, with a comparatively high photosynthetic efficiency, unlike in mex1 (Figure 4 A–C). These observations support our assumption that some mex1 characteristics such as dwarfism require a high threshold maltose concentration for induction, while others, such as changes in the chloroplast morphology are already reached in the cpMAL lines.
It has been proposed that nocturnal depletion of sugars in starch mutants (starch-less and starch-excess plants) correlates with slow growth (Casper et al., 1986; Gibon et al., 2004; Streb et al., 2012). Interestingly, neither the mex1 mutant nor the cpMAL lines seem to follow this general correlation. While mex1 plants contain low nocturnal sucrose levels, they still have substantially elevated glucose concentrations at night, which should basically compensate for the missing sucrose availability and thus allow nocturnal plant growth (Smith and Stitt, 2007). However, this mutant clearly exhibits a dwarf phenotype. By contrast, although showing decreased sucrose levels (Figure 5E) the cpMAL lines almost reach the size of the wild-types. The limited sucrose availability in these plant lines is not compensated by the reducing sugars glucose and fructose, since their concentrations are not increased when compared to the corresponding wild-type levels (Figure 5 C–D). Therefore, despite their nocturnal sugar depletion, cpMAL lines are not impaired in growth, whereas mex1 are small even though their sugar levels at night are higher. These findings challenge to some degree the paradigm that low nocturnal sugar levels per se prevent proper growth and emphasis the need to consider both, subcellular compartmentation and fluxes through sugar pools (Smith and Stitt, 2007) alongside simpler measurements of sugar levels.
The transition from vegetative growth to the onset of flowering is a key process for plants to propagate and are under control of factors like plant age, day length, and nutritional status, as reflected by the sugar availability (Moghaddam and Van den Ende, 2013; Lastdrager et al., 2014). We observed that mex1 mutants exhibit a marked delay in the onset of bolting when compared to wild-type plants. This impaired flowering initiation and inflorescence development are still present, but less pronounced in both cpMAL lines (Figure 6) and might to some degree result from the slightly slower development (Figure 3). However, the observed delay of flowering in all three mutants concurs with their nocturnally lowered sucrose levels (Figure 5E). It is well known that high cytosolic sucrose contents induce the expression of the FLOWERING LOCUS T gene (Wahl et al., 2013), coding for the FT protein which is of critical importance for initiation of flowering (Wickland Daniel and Hanzawa, 2015). Thus, the marked sucrose starvation due to the suddenly lower concentrations of this sugar in the night phases (Figure 5E) might overrule the high daytime sucrose levels, which are generally supportive for flowering induction.
In vascular plants, sufficient sugar availability is a prerequisite for the acquisition of freezing tolerance (Alberdi and Corcuera, 1991; Pommerrenig et al., 2018). Therefore, controlled starch degradation is not only essential to drive anabolic reactions required for growth and development, but also to provide soluble sugars during phases of low temperatures. Analyses of various mutants defective in starch metabolism revealed that these plants exhibit problems in adapting to low temperatures (Kaplan and Guy, 2004; Yano et al., 2005; Kaplan et al., 2006; Sicher, 2011). In this context, it seems remarkable, that mex1 mutants, despite their impairments in growth, development, metabolism, and chloroplast properties (Figure 5; Supplemental Figures S6B, 7B; Niittylä et al., 2004; Stettler et al., 2009; Purdy et al., 2013), still exhibit freezing tolerance similar to that of wild-type plants (Figure 7; Trentmann et al., 2020). Here it is important to note that both cpMAL lines exhibit even higher freezing tolerance. Therefore, we attribute the capacity of mex1 mutants to cope with cold temperatures to the high maltose levels in their chloroplasts (Lu et al., 2006a, 2006b).
Several observations suggest that accumulation of maltose in the stroma is an important factor in cold acclimation. First, the overall maltose level of leaves increases up to 100-fold when plants are exposed to low temperatures (Kaplan and Guy, 2004). Second, maltose was shown to act an efficient cryo-protectant for proteins, for membranes, and for the photosynthetic electron transport chain in vitro (Kaplan and Guy, 2004). Third, cytosolic maltose consumption by the enzyme DPE2 is inhibited in the cold due to its aggregation under those temperatures (Li et al., 2011) and DPE2 loss-of-function mutants exhibit increased freezing tolerance (Li et al., 2011). Fourth, low temperatures stimulate maltose release from starch via increased expression of ß-amylases (Kaplan and Guy, 2005; Kaplan et al., 2006; Pommerrenig et al., 2018). Fifth, cold treatment decreases the abundance of MEX1 in the envelope membrane and thus limits maltose export into the cytosol (Trentmann et al., 2020).
Consequently, depletion of stromal maltose by decreased ß-amylase activity (Kaplan and Guy, 2005; Kaplan et al., 2006; Pommerrenig et al., 2018) or in MEX1 overexpressor lines (Trentmann et al., 2020) resulted in an increased cold sensitivity of the corresponding plants. Obviously, Arabidopsis alters several cellular properties in the cold to increase the concentration of protective maltose in its chloroplasts. That the specific accumulation of this sugar in the chloroplast interior prevents freezing-induced injuries (Figure 7) concurs with data on mutants exhibiting altered levels of raffinose, representing another sugar accumulating after cold treatment (Nägele and Heyer, 2013; Nagler et al., 2015). This sugar has specifically to accumulate in chloroplasts to prevent injuries of the delicate structures located in the thylakoid membranes (Knaupp et al., 2011). Therefore, fine-tuning of the subcellular concentrations of the individual sugars is required to allow for their specific protective functions and optimal plant adaptation to cold temperatures.
The generation of the cpMAL lines paved the way in dissecting plant responses caused by a lack of starch turnover from those caused by maltose accumulation. We are optimistic that upcoming studies on cpMAL plants will provide further interesting insights into the regulation of starch degradation, and the role of maltose in the acclimation responses to environmental cues.
Materials and methods
Plant material and growth conditions
Arabidopsis (A. thaliana) plants, ecotype Columbia (Col-0) and corresponding mutant plants were cultivated on soil (type ED73, Hermann Meyer KG,//www.meyer-shop.com) in a growth chamber (Fitotron model SGR223, Weis-Gallenkamp, Heidelberg, Germany). Prior to sowing, the seeds were stratified at 4°C for 24 h in darkness. Generally, plants were grown at 125 µmol m−2s−1 light intensity under short day conditions (10 h light, 22°C/14 h darkness, 18°C). The relative humidity was 60%.
Generation of cpMAL expression constructs
For generation of the cpMAL fusion construct, the sequence coding for the yeast MAL MAL32 (YBR299W) was amplified from genomic DNA of the bakers-yeast (Saccharomyces cerevisiae) strain W303 by colony PCR with Pfu-Polymerase using the primers MAL32BamH1_s and MAL32_as (Supplemental Table S1). The amplicon (mal32) was inserted into the vector pBSK via the EcoRV restriction site. Next, the sequence coding for the first 86 amino acids of the NTT1 N-terminus was amplified via PCR with Pfu-Polymerase from Arabidopsis cDNA with the primers NTT1BamHI_s and NTT1BamH1_as (Supplemental Table S1). The resulting amplicon was fused with the mal32 sequence in pBSK via BamHI restriction and ligation. The corresponding BamHI restriction sites were introduced by the primers during the PCR. After verifying the sequence, the fusion construct served as template for amplification with primers containing attB1 and attB2 sites for recombination of the amplicon with pDONRzeo (Invitrogen) via its attP sites (Gateway BP reaction, generation of the entry clone). Expression constructs were generated by recombination of the attL sites of the entry clone and the attR sites of the destination vectors pUBDest-GFP or pUBDest (Karimi et al., 2002; Gateway LR reaction). The construct for transient expression of the cpMAL-GFP fusion was generated by using the primer pair cpMal_s/cpMal_as and the destination vector pUBDest-GFP. The construct for stable plant transformation was generated by using the primer pair cpMal_s/cpMal*_as and the vector pUBDest.
Plant transformation and localization studies
Transient transformation of Arabidopsis mesophyll protoplasts with above-mentioned constructs was performed as described by Yoo et al. (2007). A Leica TCS SP5 II confocal laser-scanning microscope (http://www.leica-microsystems.com) was used for imaging of the transformed protoplasts. All pictures were taken using the Leica HCX PL APO 63·/1.20 w mot CORR CS objective with a VIS‐Argon laser suitable for GFP constructs. GFP excitation was initiated at 488 nm, emission bandwidth was measured between 495 and 520 nm and gain was set to levels allowing a maximal dynamic range.
The floral dip method was used to generate stably transformed Arabidopsis mutant plants (Clough and Bent, 1998). cpMAL expression lines were selected by screening for the highest MAL transcript levels via RT-qPCR using the primers qRT_mal_fw and qRT_mal_rev (Supplemental Table S1). For this, RNA was extracted from frozen leaves with the NucleoSpin RNA Plant Kit (Macherey-Nagel, Düren, Germany) according to the manufacturer’s instructions. RNA was transcribed into cDNA with the qScript cDNA Synthesis Kit (Quantabio, Beverly, USA). The genes act2 (At3g8780) and pp2a (At1g13320) served as references for transcript normalization (Supplemental Table S1).
Metabolite extraction and quantification
The experimental procedure for sugar and starch extraction and quantification was performed exactly as described earlier (Patzke et al., 2019).
MAL activity assay
MAL activity was determined by measuring the increase in absorbance at 400 nm caused by the hydrolysis of p-nitrophenyl-α-D-glucopyranoside. One unit can hydrolyze one µmole of p-nitrophenyl-D-glucopyranoside under the specified conditions, at 37°C and pH 6.8 (http://www.worthington-biochem.com/MALT/assay.html). The assay conducted and data were calculated as described in the Worthington enzyme manual. Soluble proteins were isolated from 100 mg of powdered (in liquid N2) plant material by addition of 300 µL of protein extraction buffer medium (50 mM Hepes/NaOH pH 6.8, 20 mM MgCl2, and 10 mM DTT) supplemented with 1× protease inhibitor cocktail (Roche, Basel, Switzerland).
Chlorophyll extraction and determination
Chlorophyll quantification was performed with modifications according to an established method (Arnon, 1949). Ground plant material (20 mg) was dissolved in 250 µL 80% (v/v) ethanol with 10 mM Hepes/NaOH pH 7.0, heated for 20 min at 80°C and centrifuged for 5 min at 17,000× g. The extraction was repeated 2 times with the remaining sediment. All three supernatants were pooled and extinction was measured at 645 and 665 nm in a microplate reader (Tecan Infinite 200 PRO, www.tecan.com).
Microscopic analyses
Samples for light and electron microscopy analysis were prepared and imaged exactly as described earlier (Abt and Zeeman, 2020).
PAM measurements
A chlorophyll fluorometer (Imaging-PAM M-Series-System, Walz, Würzburg, Germany; http://www.walz.com) was used for photosynthetic activity determination. Plants were dark acclimated for 15 min to deplete the energy of PSII. The capacity of PSII was measured by saturation with 15 cycles of PPFD 76 (μmol photons m−2s−1) light-pulses at 0, 50, and 70 s. The ImagingWin software was used for calculation of effective quantum yield of PSII [Y(II)] and quantum yield of NPQ [Y(NPQ)] (Martins Rodrigues et al., 2020).
Freezing recovery experiment
The freezing tolerance test was determined with plants cultivated for 24 d at standard conditions. Cold acclimation and application of freezing temperatures were conducted as described previously in (Trentmann et al., 2020). In brief, prior to freezing, plants were acclimated to cold by exposure to 4°C for 4 d. For subsequent freezing, the temperature was decreased stepwise by 2°C/h to −10°C finally. Plants were kept at −10°C for 15 h. For retransfer to standard conditions (22°C day and 18°C night), the cultivation temperature was raised again stepwise by 2°C/h. Plants were then cultivated at standard conditions for 3 weeks. The plants were photographed and the survival rate was calculated.
Accession numbers
Plastidic maltose exporter MEX1 At5g17520, Bakers-yeast (S. cerevisiae) MAL32: F8LG06_YEASX (Uniprot).
Supplemental Data
The following supplemental materials are available in the online version of this article.
Supplemental Figure S1. Plastidic MAL activity alleviates the dwarf phenotype of 2-week-old mex1 mutants.
Supplemental Figure S2. Plastidic MAL activity alleviates the dwarf phenotype of 6-week-old mex1 mutants.
Supplemental Figure S3. Identification of cpMAL in Arabidopsis wild-type background and morphological phenotype.
Supplemental Figure S4. Plastidic MAL activity restores the chlorophyll a/b ratio of mex1.
Supplemental Figure S5. Microscopic analysis of leaf thickness.
Supplemental Figure S6. Analysis of the chloroplast morphology in mesophyll cells.
Supplemental Figure S7. Analysis of mesophyll cell chloroplast ultrastructure.
Supplemental Figure S8. Graphic scenario displaying effects of the various maltose levels in wild-type, mex1, and cpMAL chloroplasts.
Supplemental Table S1. Primers used for cloning and qRT-PCR.
Acknowledgments
We thank Dr. Jörg Fettke (University of Potsdam, Germany) for providing dpe2–5 seeds.
Funding
Deutsche Forschungsgemeinschaft (DFG) Transregio-Sonderforschungsbereich TRR175 (project B03).
Conflict of interest statement. None declared.
J.C. designed and performed research, I.H. wrote the article, R.R. performed research, I.K., performed research, B.P. analyzed data, O.T. performed research, J.A. performed research, M.F.-S. contributed new tools, S.E. contributed new tools, S.C.Z. analyzed the data, H.E.N. designed research, analyzed the data, and wrote the article.
The author responsible for distribution of materials integral to the findings presented in this article in accordance with the policy described in the Instructions for Authors (https://dbpia.nl.go.kr/plphys/pages/general-instructions) is: Ekkehard Neuhaus ([email protected]).
References
Author notes
Senior authors.