-
PDF
- Split View
-
Views
-
Cite
Cite
Xinyu Xu, Zhiguo E, Dongping Zhang, Qianbin Yun, Yong Zhou, Baixiao Niu, Chen Chen, OsYUC11-mediated auxin biosynthesis is essential for endosperm development of rice, Plant Physiology, Volume 185, Issue 3, March 2021, Pages 934–950, https://doi.org/10.1093/plphys/kiaa057
- Share Icon Share
Abstract
Auxin is a phytohormone essential for plant development. However, our understanding of auxin-regulated endosperm development remains limited. Here, we described rice YUCCA (YUC) flavin-containing monooxygenase encoding gene OsYUC11 as a key contributor to auxin biosynthesis in rice (Oryza sativa) endosperm. Grain filling or storage product accumulation was halted by mutation of OsYUC11, but the deficiencies could be recovered by the exogenous application of auxin. A rice transcription factor (TF) yeast library was screened, and 41 TFs that potentially bind to the OsYUC11 promoter were identified, of which OsNF-YB1, a member of the nuclear factor Y family, is predominantly expressed in the endosperm. Both osyuc11 and osnf-yb1 mutants exhibited reduced seed size and increased chalkiness, accompanied by a reduction in indole-3-acetic acid biosynthesis. OsNF-YB1 can bind the OsYUC11 promoter to induce gene expression in vivo. We also found that OsYUC11 was a dynamically imprinted gene that predominantly expressed the paternal allele in the endosperm up to 10 d after fertilization (DAF) but then became a non-imprinted gene at 15 DAF. A functional maternal allele of OsYUC11 was able to recover the paternal defects of this gene. Overall, the findings indicate that OsYUC11-mediated auxin biosynthesis is essential for endosperm development in rice.
Introduction
The endosperm of cereal crops, which is essential for embryonic and seed development, provides most of the calories consumed by humans (Leroux et al., 2014). In angiosperms, endosperm originates from a double fertilization event (Olsen, 2001). The egg and central cells of an embryo sac are fertilized by two sperm cells and develop into the embryo and endosperm, respectively. In rice (Oryza sativa), endosperm development can be divided into four main phases according to histological characteristics (Wu et al., 2016): (1) coenocyte [1–2 d after fertilization {DAF}], (2) cellularization (3–5 DAF); (3) storage product accumulation (6–20 DAF); and (4) maturation (21–30 DAF). The storage product accumulation phase is also known as grain filling, and this phase largely determines the final yield and quality of rice. Most carbohydrates and storage proteins are accumulated at this stage (Wu et al., 2016).
Auxin and its interactions with other phytohormones are crucial to the regulation of plant development (Zhao, 2018). Indole-3-acetic acid (IAA) is an active endogenous form of auxin. The TRYPTOPHAN AMINOTRANSFERASE OF ARABIDOPSIS (TAA) family of aminotransferases can convert tryptophan into indole-3-pyruvate (IPA), which is then converted into IAA by a second reaction catalyzed by YUCCA (YUC) flavin-containing monooxygenases (Ljung, 2013; Zhao, 2018). This two-step reaction for IAA biosynthesis is known as the TAA–YUC pathway. Many genes involved in auxin production are indispensable for embryogenesis. For example, taa1, tar1, and tar2 triple mutants and yuc1, yuc4, yuc10, and yuc11 quadruple mutants exhibited severe embryo developmental defects in Arabidopsis (Arabidopsis thaliana; Cheng et al., 2007; Stepanova et al., 2008).
Compared with our knowledge of auxin-regulated patterning and embryogenesis, our understanding of auxin-regulated endosperm development remains poor (Basunia and Nonhebel, 2019). Fertilization induces auxin production to reduce the repression of central cell proliferation (Figueiredo et al., 2015). Conversely, increased auxin biosynthesis in the endosperm prevents cellularization in Arabidopsis (Batista et al., 2019). However, application of 2,4-dichlrophenoxyacetic acid, an active analog of IAA, to the developing maize kernel was shown to promote endosperm cellularization (Lur and Setter, 1993a). The quantitative trait locus Thousand-Grain Weight 6 (TGW6) of rice encodes an IAA-glucose hydrolase, which catalyzes the conversion of IAA-glucose to free IAA in the developing caryopsis (Ishimaru et al., 2013). Cellularization of these accessions with functional TGW6 is accelerated as a result of increased auxin accumulation in the endosperm (Ishimaru et al., 2013). With the assistance of AGAMOUS-like 62 (AGL62), a MADS-box family transcription factor (TF), auxin produced in Arabidopsis endosperm can drive seed coat development in response to fertilization (Figueiredo et al., 2016). Auxin is also believed to be important for aleurone cell differentiation (Becraft and Yi, 2011). Correspondingly, application of the auxin transport inhibitor N-1-naphthylphthalamic acid (NPA) resulted in multiple aleurone layers in maize (Forestan et al., 2010). The small kernel maize mutant miniature1 (mn1) exhibited decreased auxin levels in the developing seeds as a result of loss-of-function of the cell wall invertase INCW2 (LeClere et al., 2010). Mutants such as defective kernel6 (dek6), dek18, and dek26 also had lower IAA levels in the kernel, whereas dek8, dek12, and dek24 had higher IAA levels than the corresponding wild-type (WT) plants (Lur and Setter, 1993b; Bernardi et al., 2016). Exogenous application of auxin reception and biosynthesis inhibitors during grain filling led to seed weight reduction in rice (Tamaki et al., 2015). These findings suggest that auxin plays essential roles in endosperm development by modulating the timing of cellularization, differentiation of aleurone cells, and accumulation of storage products or grain filling.
Genomic imprinting is a non-Mendelian phenomenon that occurs in parental genomes through the differential modification of epigenetic marks such as DNA methylation and histone modification. This leads to monoallelic expression, or the preferential expression of a single allele, at a certain locus in a parent-of-origin-dependent manner (Köhler et al., 2012; Gehring, 2013). Monoallelic expression of the imprinted genes increases the risk of genetic mutations altering function due to the lack of a second allele to complement the adverse effects caused by the mutations. Several hypotheses such as the parental conflict theory have been proposed to explain the potential advantages of genomic imprinting from an evolutionary perspective (see the review by Rodrigues and Zilberman, 2015). Several studies have shown that imprinted genes, most of which are maternally expressed genes, are necessary for seed development in angiosperms (Ohad et al., 1996; Chaudhury et al., 1997; Kiyosue et al., 1999; Köhler et al., 2003; Tiwari et al., 2008; Gerald et al., 2009; Costa et al., 2012; Liu et al., 2014; Cheng et al., 2020a, 2020b; Niu et al., 2020). In contrast, mutations in paternally expressed genes (PEGs) typically do not cause obvious developmental defects in Arabidopsis (Wolff et al., 2015). Recent findings suggest that PEGs are likely important for endosperm-based hybridization barriers (Kradolfer et al., 2013; Wolff et al., 2015; Jiang et al., 2017; Wang et al., 2018).
Genomic imprinting predominantly occurs in the endosperm of angiosperms (Pires and Grossniklaus, 2014). However, imprinted genes often exhibit limited conservation in plants (Waters et al., 2013; Hatorangan et al., 2016; Chen et al., 2018; Yang et al., 2018). Some genes involved in auxin biosynthesis, including YUC and TAR, are rare examples of imprinted genes conserved among different plant species (Xin et al., 2013; Chen et al., 2018). For example, Arabidopsis YUC10 (AtYUC10), maize YUC1 (ZmYUC1), and rice YUC11 (OsYUC11) as well as AtTAR1 of Arabidopsis, OsTAR1 of rice, and ZmTAR1 of maize, are PEGs that represent groups of homologs (Chen et al., 2018).
The atyuc10 mutant expressed no distinct seed phenotype despite the necessity for auxin in endosperm development, which likely occurred due to compensation through other functional YUCs encoded by the Arabidopsis genome (Figueiredo et al., 2015). ZmYUC1 is the causal gene of defective endosperm18 (de18), which showed <50% dry weight accumulation in the kernel as well as reduced endoreduplication (Bernardi et al., 2012). The mutant strain only accumulated 1%–7% free IAA of that observed in the WT (Bernardi et al., 2012), confirming that ZmYUC1 was a key enzyme in IAA biosynthesis in maize endosperm. The mutant strains of many PEGs do not exhibit visible defects, which contends the importance of imprinted genes (Rodrigues and Zilberman, 2015; Wolff et al., 2015). ZmYUC1 is an example of a PEG that may also function in endosperm and seed development (Bernardi et al., 2012, 2019). To date, the effects of auxin on the regulation of endosperm development in rice remain poorly understood. Several endosperm-preferential YUCs, including OsYUC9, OsYUC11, and OsYUC12, likely influence seed development because they are abundant in developing caryopsis, but this lacks genetic evidence (Abu-Zaitoon et al., 2012; Zhang et al., 2020). In the present study, we unambiguously confirmed that YUC-mediated auxin biosynthesis is essential for the development of rice endosperm.
Results
A phylogenetic group of YUCs is preferentially expressed in rice endosperm
The Arabidopsis and rice genomes encode 11 and 14 YUC genes, respectively (Zhang et al., 2018). On analyzing the YUC protein sequences, we found that AtYUC10 and 11, as well as OsYUC9, 10, 11, 12, 13, and 14, grouped together to form a specific phylogenetic clade (Figure 1A). Previous studies showed that AtYUC10 and 11 are involved in Arabidopsis embryogenesis and endosperm development (Cheng et al., 2007). Similarly, our reverse transcription quantitative polymerase chain reaction (RT-qPCR) analysis indicated that OsYUC9, 11, and 12 were highly expressed in the caryopsis of Kitaake (O. sativa ssp. japonica; Supplemental Figure S1, A–D). OsYUC9 was also expressed in the shoot, root, stem, and young panicle (Supplemental Figure S1A), whereas OsYUC11 and 12 were barely detectable in vegetative tissues (Supplemental Figure S1, B and C). A time-course analysis indicated the stable activation of OsYUC9 in the caryopsis from 5 to 15 DAF (Figure 1B). Similarly, OsYUC11 was activated at approximately 5 DAF, and its expression persistently increased before peaking at 15 DAF (Figure 1B). OsYUC12 was temporally activated at 3–5 DAF and was then quickly silenced (Figure 1B), which suggested that OsYUC12 plays a role that is distinct from that of OsYUC9 and 11 in seed development. OsYUC10 predominantly expressed in leaves, whereas OsYUC13 and 14 showed extremely low expression in all analyzed tissues (Figure 1B; Supplemental Figure S1D). We therefore focused on OsYUC9, 11, and 12 to investigate YUC-mediated biosynthesis for rice endosperm development.
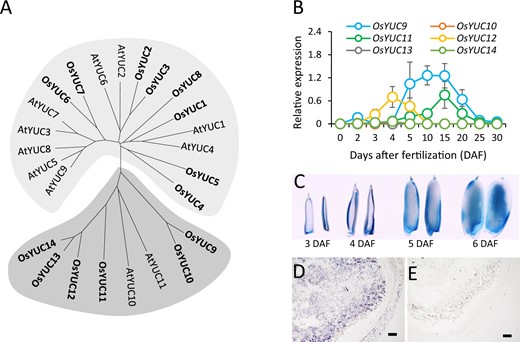
Phylogenetic and expression analyses of OsYUC9, 10, 11, 12, 13, and 14. (A) Neighbour-joining tree of the YUCCA family members in Arabidopsis (AtYUCs) and rice (OsYUCs). (B) Relative expression of OsYUC9, 10, 11, 12, 13, and 14 in different-aged developing caryopses of Kitaake (O. sativa ssp. japonica). Three biological replicates were used for the RT-qPCR. Error bars indicate standard errors. (C) GUS-staining reactions of the caryopses produced by the pOsYUC11::GUS reporter line. The GUS gene was driven by the promoter of OsYUC11. From left to right: staining of the 3-, 4-, 5-, and 6-DAF-old caryopses produced by the pOsYUC11::GUS reporter line. (D and E) In situ hybridization of OsYUC11 in caryopsis at 6 DAF, using an anti-sense (D) and a sense probe (E). Scale bars = 100 μm.
Next, we detected the expressions of OsYUC9, 11, and 12 in the embryo and endosperm at 7 DAF. The results indicated that OsYUC9 and 11 were predominantly expressed in the rice endosperm (Supplemental Figure S1E). OsYUC12 expression was barely detectable in both the endosperm and embryo at 7 DAF (Supplemental Figure S1E), consistent with the transient activation of OsYUC12 in seeds (Figure 1B). We generated transgenic lines that expressed β-glucuronidase (GUS) driven by the OsYUC11 promoter. GUS staining signals were observed in the endosperm at approximately 5–6 DAF (Figure 1C). Consistent with this observation, in situ hybridization assay results suggested that OsYUC11 is predominately expressed in endosperm cells at 6 DAF (Figure 1, D and E).
OsYUC11 mutation led to grain filling defects
To further elucidate the effect of OsYUC11 on seed development, we prepared two independent CRISPR/Cas9 constructs to target different OsYUC11-encoding regions for gene mutation. Four distinct homozygous mutants were obtained at T0 generation (Supplemental Figure S2A). All mutant strains produced truncated proteins due to frame shifting. No vegetative defects were observed in the osyuc11 mutant strains (Figure 2A; Supplemental Figure S2B). Agronomic traits such as plant height, panicle number, and setting rate were not noticeably affected in the osyuc11 mutants (Supplemental Figure S2, C–E). However, the seed weight of osyuc11 was reduced to 60%–70% of that of the WT (Figure 2B). The decreased seed weight was largely due to a significant decrease in seed thickness (Supplemental Figure S2, F and G). The phenotypes were consistent among the different mutant alleles (Figure 2, B and C;Supplemental Figure S2, C–G).
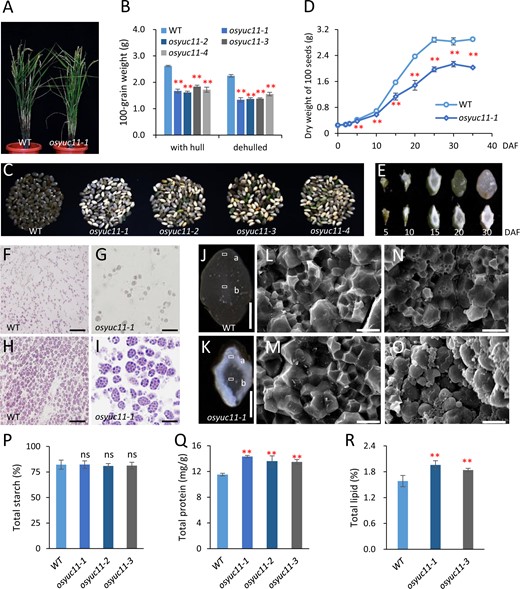
Phenotypic characterization of osyuc11 mutants. (A) Plant morphologies of the WT (left) and osyuc11-1 (right) at the heading stage. (B) One-hundred grain weight with or without hulls. Three biological replicates were used for phenotyping. Each replicate consisted of at least 200 seeds. Error bars indicate standard deviation. Student’s t test was used for statistical analysis. **P<0.01. (C) Mature caryopses produced by the WT and four allelic mutants. From left to right: WT, osyuc11-1, osyuc11-2, osyuc11-3, and osyuc11-4. (D) Dynamic curves of the dry weight accumulation in the caryopses of WT and osyuc11-1. Three biological replicates were used for phenotyping. Error bars indicate standard deviation. Student’s t test was used for statistical analysis. **P<0.01. (E) Morphology of the dried caryopses of the WT (upper) and osyuc11-1 (lower), at 5, 10, 15, 20, and 30 DAF (from left to right). (F–I) The starch granules accumulated in the WT (F and H) and osyuc11-1 (G and I) at 5 DAF (F and G) and 8 DAF (H and I). Scale bars = 100 μm. (J, K) Cross-sections of caryopses produced by the WT (J) and osyuc11-1 (K). Scale bars = 1 mm. “a” and “b” indicate the peripheral and central endosperms, respectively, that were used for SEM observations in (L–O). (L–O) SEM images of the starch accumulated in the central (L and M) and peripheral endosperm (N and O) of the WT (L and N) and osyuc11-1 (M and O). Scale bars =100 μm. (P–R) Total starch (P), protein (Q), and lipid (R) contents of mature seeds produced by the WT and osyuc11 mutants. Three biological replicates were used for analysis. Error bars indicate standard deviation. Student’s t test was used for statistical analysis. NS, not significant; **P<0.01.
The dry weight of mutant caryopses was significantly lower than that of the WT from 5 DAF (Figure 2D). We performed morphological comparisons and found that a dried osyuc11 caryopsis at 30 DAF resembled the WT at 15 DAF (Figure 2E). These findings suggest that OsYUC11 is essential for grain filling in rice. Microscopic observation showed that the starch granules of osyuc11-1 were much smaller than those of the WT at both 5 and 8 DAF (Figure 2, F–I). Consistently, the expression of important genes, such as OsSSSIIa, OsBEIIb, OsSSIIIa, and OsGBSSI, which are involved in starch biosynthesis, was deregulated in developing osyuc11 caryopsis (Supplemental Figure S3, A–D). Sucrose and fructose contents showed no differences between the mutant and WT at 5, 10, and 15 DAF (Supplemental Figure S4, A and B). The osyuc11-1 caryopses had significantly higher glucose contents even at 10 and 15 DAF (Supplemental Figure S4C), suggesting that slow grain filling in the mutants was not because of defects in sugar loading or conversion from the source organs.
A previous study showed that YUC-mediated auxin biosynthesis is crucial for early endosperm development in Arabidopsis (Figueiredo et al., 2015). However, no developmental defect was observed in the early-developing osyuc11-1 endosperm (Supplemental Figure S5, A–F). In addition, aleurone cell differentiation was not influenced (Supplemental Figure S5, G and H). Mature osyuc11 seeds germinated slower than WT seeds, although the final germination rate remained largely unaffected (Supplemental Figure S5I). Because the embryo development of osyuc11-1 was normal (Supplemental Figure S5, J and K), we believe that delayed germination is a possible consequence of endosperm filling defects.
Storage product accumulation in osyuc11 endosperm
A large proportion of mature osyuc11 seeds were chalky (Figure 2C), of which peripheral endosperm was usually floury with a normal central endosperm (Figure 2, J and K). Scanning electron microscopy (SEM) analysis revealed that starch was loosely packed in peripheral osyuc11-1 endosperm cells but was normal in the central endosperm cells (Figure 2, L–O). The mutants and WT both contained similar amounts of total starch (Figure 2P). Biochemical and physical properties of the starch collected from osyuc11-1 were generally unchanged, although the gel consistency was reduced (Supplemental Figure S6, A–C).
The total protein content of osyuc11-1 caryopses was significantly higher than that of the WT (Figure 2Q). Quantitative and sodium dodecyl sulfate–polyacrylamide gel electrophoresis (SDS–PAGE) analyses further confirmed that a higher amount of prolamins and glutelins accumulated in the osyuc11-1 and osyuc11-3 endosperms, whereas albumin and globulin amounts were less affected (Supplemental Figure S7, A–E). The total lipid contents of osyuc11-1 and osyuc11-3 were higher than that of the WT (Figure 2R). The lipid compositions were also changed; for example, palmitic acid (C16:0) and stearic acid (C18:0) amounts were reduced, but the amounts of linolenic acid (C18:3) and arachidic acid (C20:0) were increased in mature mutant seeds (Supplemental Figure S7F).
Auxin biosynthesis was depleted in osyuc11 caryopses
DR5 is an artificially synthesized auxin-responsive element, which can be used as a reporter to visualize the endogenous distribution of auxin (Ulmasov et al., 1997). Using the DR5::GUS line, we found that auxin accumulation in the endosperm rapidly increased at 5 DAF (Figure 3A). This is consistent with previous findings which showed that IAA concentrations in rice endosperm rapidly increased during this stage (Nonhebel and Griffin, 2020; Zhang et al., 2020). Notably, the rapid IAA accumulation in our study occurred in parallel with the expression of OsYUC11 in rice (Figure 1B). On measuring IAA content, we found that the OsYUC11 defects halted IAA biosynthesis in the caryopsis. The IAA content of mutant plants was only 0.5%–2% of that in WT plants at 10 DAF (Figure 3B). Additionally, the expression of some genes responsible for auxin biosynthesis, such as OsTAR2, OsGH3-2, and OsGH3-4, was substantially inhibited in osyuc11 caryopses (Figure 3, C–E). We also investigated the expression patterns of OsYUC9 and OsYUC12 in osyuc11 caryopses and found that OsYUC9 and OsYUC12 were activated in the mutants at 10 DAF, possibly to compensate for OsYUC11 defects (Supplemental Figure S8A).
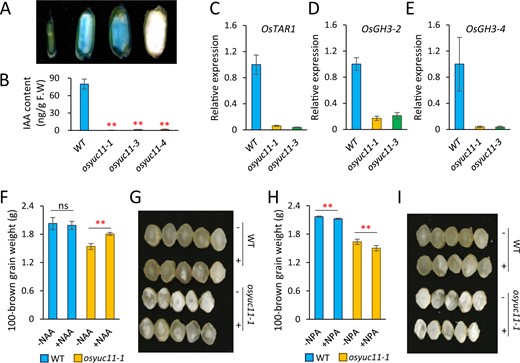
Reduced auxin biosynthesis in the caryopsis of osyuc11. (A) GUS staining of the caryopses produced by a DR5::GUS reporter line (Dongjin, O. sativa ssp. japonica). From left to right: 4-, 5-, 6-DAF-old caryopses produced by DR5::GUS, and a 6-DAF-old caryopsis produced by Dongjin, respectively. (B) Free auxin content in the 10 DAF caryopses of the WT and osyuc11 mutants. Three biological replicates were used for analysis. Error bars indicate standard deviation. Student’s t test was used for statistical analysis. **P<0.01. (C–E) Expression of OsTAR2 (C), OsGH3-2 (D), and OsGH3-4 (E) in the caryopses of the WT, osyuc11-1, and osyuc11-3 at 10 DAF. Three biological replicates were used for analysis. Error bars indicate standard deviation. (F–I) Exogenous applications of NAA (α-naphthalene acetic acid, 100 μM) (F and G) and NPA (N-1-naphthylphthalamic acid, 100 μM) (H and I) alleviated and strengthened the defects of osyuc11-1, respectively, in terms of seed weight (F and H) and chalkiness (G and I). Three biological replicates were used for analysis (F and H). Error bars indicate standard deviation. Student’s t test was used for statistical analysis. **P<0.01. “+” and “−” in (G and I) indicate the “application” and “no application” of chemicals, respectively.
To confirm that the developmental defects of osyuc11 was a consequence of auxin biosynthesis depletion, we sprayed the panicles of the mutants with 100-μM α-naphthalene acetic acid (NAA), an active synthetic auxin, every other day after anthesis. As expected, the seed weight of osyuc11-1 increased by 17.31% compared with that in untreated plants (Figure 3F). The seed weight of NAA-treated seeds recovered to ∼91% of that of the WT (Figure 3F). The chalkiness of osyuc11-1 was also alleviated (Figure 3G). In contrast, the WT response to NAA treatment was limited (Figure 3, F and G). We also treated the mutant and WT strains with NPA, a polar auxin transport inhibitor. This led to 8.93% and 2.18% reductions in the seed weight of osyuc11-1 and WT, respectively (Figure 3, H and I). These findings strongly imply that OsYUC11 regulates grain filling via auxin biosynthesis in rice endosperm.
Transcriptome analysis revealed metabolic disorders in the osyuc11 caryopses
We performed RNA-sequencing to investigate transcriptome changes in WT and mutant caryopses at 10 DAF, given that OsYUC11 had been activated and the morphological differences were observable at this stage of development. There were 282 down- and 242 upregulated genes in both osyuc11-1 and osyuc11-3 [false discovery rate {FDR} <0.05, fold change >2] (Supplemental Table S1). The gene ontology (GO) analysis indicated that the differentially expressed genes (DEGs) were enriched in primary metabolic (FDR = 5.3e–70), lipid metabolic (FDR = 1.5e–14), and protein metabolic processes (FDR = 4.1e–14), consistent with the finding that storage product accumulation was disrupted in osyuc11. Several key genes involved in endoplasmic reticulum stress in rice, such as BiP1, BiP4, PDIL1-1, OsbZIP60, and CTR1, were differentially expressed (Supplemental Table S1). Additionally, many heat shock protein- and dehydration-responsive element-binding protein encoding genes were significantly activated in the osyuc11 endosperm (Supplemental Table S1). The GO term “response to stress” was consequently overrepresented (FDR = 8.8e–23). Genes encoding TFs were also enriched among the DEGs (FDR = 3.1e–08), indicating deregulation of gene expression in the mutant. For instance, OsEBP-89, which was significantly repressed in the mutant endosperm, is a key regulator of starch biosynthesis (Zhu et al., 2003). The GO term “response to endogenous stimulus” was enriched (FDR = 0.021). Several auxin-responsive genes, including OsGH3-2, OsGH3-4, OsGH3-8, OsTAR2, and OsIAA15, were downregulated in the mutant strain (Supplemental Table S1).
OsNF-YB1 regulates OsYUC11 expression
To study the transcriptional regulation of OsYUC11, we searched for TFs that could bind to the OsYUC11 promoter (−350 to −1) using a yeast-one-hybrid approach. Forty-one TFs belonging to different TF families were identified (Supplemental Table S2). On analyzing the publicly available expression atlas, we found that among the 41 TF-encoding genes, a CCAAT family member, OsNF-YB1, and a MADS-box gene, OsMADS14, exhibited expression patterns with the highest similarity to that of OsYUC11 in the developing caryopsis (Figure 4A). Whereas OsMADS14 was also expressed in other vegetative and reproductive tissues (Arora et al., 2007), OsNF-YB1 was exclusively expressed in the endosperm. A mutation in OsNF-YB1 led to reduced seed size and increased chalkiness (Figure 4B; Bai et al., 2015; Xu et al., 2016; E et al., 2018), similar to the phenotype observed in osyuc11. Therefore, we assumed that OsNF-YB1 might directly regulate the expression of OsYUC11.
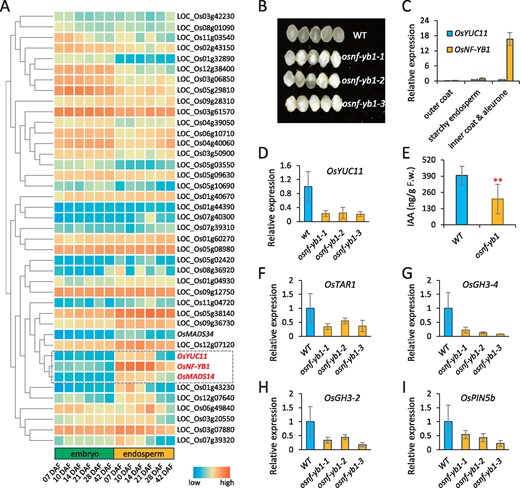
OsNF-YB1 defects suppressed OsYUC11 expression and IAA biosynthesis in the caryopses. (A) Clustering analysis of the expression of 41 TFs with binding abilities to the OsYUC11 promoter, which were identified by a yeast-one-hybrid approach. (B) Morphology of mature caryopses produced by the WT and the osnf-yb1 mutants. (C) Expression of OsYUC11 and OsNF-YB1 in the outer caryopsis coat, inner caryopsis coat including aleurone cells, and starchy endosperm cells of WT. Three biological replicates were used for analysis. Error bars indicate standard deviation. (D) Relative expression of OsYUC11 in the caryopses of WT and osnf-yb1 mutants, at 8 DAF. Three biological replicates were used for analysis. Error bars indicate standard deviation. (E) Free auxin content in the 10-DAF-old caryopses produced by WT and osnf-yb1-1. Three biological replicates were used for analysis. Error bars indicate standard deviation. Student’s t test was used for statistical analysis. **P<0.01. (F–I) Expression of OsTAR1 (F), OsGH3-4 (G), OsGH3-2 (H), and OsPIN5b (I) in the caryopses of the WT and osnf-yb1 mutants, at 8 DAF. Three biological replicates were used for analysis. Error bars indicate standard deviation.
Previous studies suggested that OsNF-YB1 is predominantly expressed in aleurone cells (Bai et al., 2015; Xu et al., 2016), whereas OsYUC11 is likely ubiquitously expressed in endosperm cells (Figure 1D). Because the aleurone and sub-aleurone layers are tightly attached to the inner caryopsis coat (Hay and Spanswick, 2006), we compared the expression of OsNF-YB1 and OsYUC11 in the dissected endosperm, as well as in the outer and inner caryopsis coats (10 DAF). OsNF-YB1 was overexpressed in the inner but not outer coat of caryopses (Figure 4C), consistent with OsNF-YB1 overexpression in aleurone cells. We also detected OsNF-YB1 expression in the starchy endosperm, but at lower levels, and the expression level was equivalent to that of OsYUC11 (Figure 4C). These findings suggested that OsNF-YB1 and OsYUC11 were co-expressed in the aleurone and starchy endosperm cells, with higher OsNF-YB1 expression in aleurone cells than OsYUC11 expression.
In comparison to the expression of OsYUC11 in WT caryopsis, we found that OsYUC11 was substantially repressed in three independent osnf-yb1 mutant lines (Figure 4D). Coincidently, osnf-yb1 accumulated a lower amount of IAA than WT in the 10-DAF-old caryopses (Figure 4E). The expressions of several auxin-responsive genes such as OsTAR2, OsGH3-2, OsGH3-4, and OsPIN5b, were also downregulated in the mutants (Figure 4, F–I). These results indicated that OsNF-YB1 may activate OsYUC11 expression. In contrast, OsNF-YB1 expression was not different from the WT in the caryopsis of osyuc11 (Supplemental Figure S8B). We also investigated the expression of OsYUC11 in the OsNF-YB1 overexpression line OsNF-YB1OX. As expected, accompanied by the activation of OsNF-YB1, OsYUC11 was upregulated in the 5-DAF-old OsNF-YB1OX caryopses and seedlings (Figure 5, A–D).
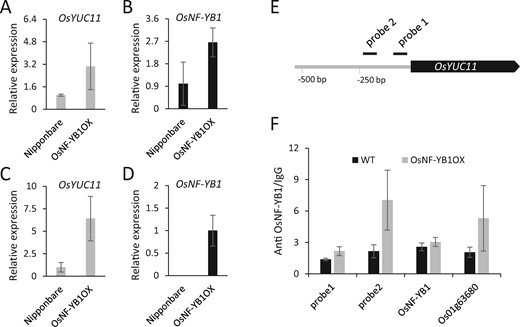
OsNF-YB1 activates OsYUC11 expression in vivo. (A and B) Expression of OsYUC11 (A) and OsNF-YB1 (B) in OsNF-YB1OX caryopses at 5 DAF. Three biological replicates were used for analysis. Error bars indicate standard deviation. (C and D) Expression of OsYUC11 (C) and OsNF-YB1 (D) in the OsNF-YB1OX seedlings. Three biological replicates were used for analysis. Error bars indicate standard deviation. (E) A schematic illustration of the probes used for ChIP. (F) ChIP analysis of OsNF-YB1 binding of the promoter of OsYUC11 in WT and OsNF-YBOX plants. Enrichment of OsNF-YB1 binding of the OsYUC11 promoter (probes 1 and 2), OsNF-YB1 (negative control) and Os01g63680 (positive control) were evaluated using quantitative PCR. Two biological replicates both showed similar results, and one replicate is shown here. Error bars indicate standard deviation. Leaves of 2-week-old WT and OsNF-YB1OX seedlings were used for analysis.
To test the hypothesis that OsNF-YB1 directly regulates the expression of OsYUC11 in rice, we performed an electrophoretic mobility shift assay to detect the binding ability of OsNF-YB1 to the OsYUC11 promoter in vitro. However, by surveying six probes spread across the promoter region, we failed to detect this binding ability (Supplemental Figure S9, A and B). Dual-luciferase reporter assay results also suggested the inability of OsNF-YB1 to activate the reporter gene driven by OsYUC11 promoter in the epidermal cell of Nicotiana tabacum (Supplemental Figure S9C). Considering that NF-Y TFs usually form heterotrimers for their function, we assumed that NF-YB1 alone is not sufficient to activate OsYUC11 expression in vitro. Because ectopic expression of OsNF-YB1 activated OsYUC11 in the OsNF-YB1OX seedlings (Figure 5C), we performed a chromatin immunoprecipitation (ChIP) assay coupled with qPCR (ChIP-qPCR) to analyze the binding ability of OsNF-YB1 in vivo. The levels of probe 2 targeting the OsYUC11 promoter (Figure 5E) were substantially enriched, similar to that of the positive control Os01g63680 (Xu et al., 2016), whereas the negative control OsNF-YB1 (Xu et al., 2016) showed no enrichment in the OsNF-YB1OX seedlings (Figure 5F). These findings suggest that OsNF-YB1 binds to the OsYUC11 promoter to activate gene expression in vivo.
OsYUC11 is dynamically imprinted in the rice endosperm
OsYUC11 was previously identified as an imprinted gene that predominantly expressed the paternal allele in the endosperm (Luo et al., 2011; Yuan et al., 2017; Chen et al., 2018). To assess whether imprinting of OsYUC11 is essential for endosperm development, we performed reciprocal crosses between WT and osyuc11 mutants. By using osyuc11 as the pollen donor and WT as the mother, we assumed that hybrid seeds would exhibit phenotypes similar to those of the mutant as a result of the inactivation of the maternal allele in the endosperm. Surprisingly, we found that WT (♀)/osyuc11-1 (♂) seeds were more similar to WT (♀)/WT (♂) seeds than to osyuc11-1 (♀)/osyuc11-1 (♂) seeds (Figure 6, A and B). Similar results were obtained when osyuc11-3 was used for the crosses (Figure 6, A and B).
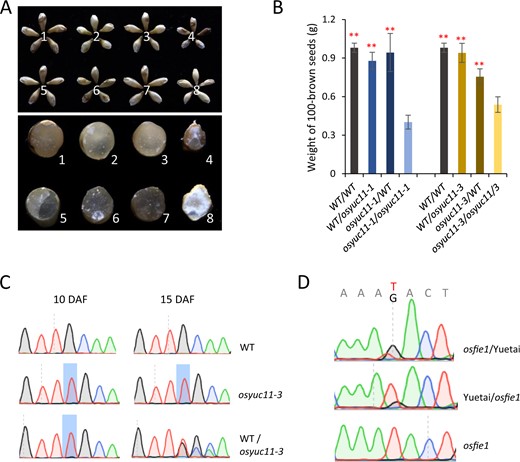
Dynamic imprinting of OsYUC11. (A and B) Reciprocal crosses showing that a maternally originated OsYUC11 was able to recover the paternal defect of osyuc11. The upper and lower panel shows the intact caryopses produced by different crossing combinations and their cross sections, respectively. 1, WT/WT; 2, osyuc11-1/WT; 3, WT/osyuc11-1; 4, osyuc11-1/osyuc11-1; 5, WT/WT; 6, osyuc11-3/WT; 7, WT/osyuc11-3; 8, osyuc11-3/osyuc11-3. For convenience, the first parent in a combination indicates the mother used for crossing. (B) One hundred brown seed weights of the seeds produced by different crossing combinations. Three biological replicates were used for the quantification of WT (♀)/WT (♂), osyuc11-1 (♀)/WT (♂), WT (♀)/osyuc11-1 (♂), WT (♀)/WT (♂), osyuc11-3 (♀)/WT (♂), and WT (♀)/osyuc11-3 (♂). Error bars indicate standard deviation. Student’s t test was used for statistical analysis. **Statistical significance at P < 0.01 level when compared with corresponding artificially crossed mutant seeds. (C) Sanger sequencing of the RT-PCR products of OsYUC11 amplified from the endosperm of WT/osyuc11-3, at 10 and 15 DAF. (D) The paternal expression bias of OsYUC11 remained unchanged in the endosperm produced by osfie1 (in Kitaake background) and Yuetai (O. sativa ssp. indica) reciprocal crosses, at 7 DAF.
Considering the possibility for dynamic imprinting during seed development, we sequenced OsYUC11 complementary DNAs (cDNAs) in WT (♀)/osyuc11-3 (♂) endosperm at various ages. The results showed that the paternal OsYUC11 allele was exclusively expressed in the endosperm at 10 DAF. However, the expression of the maternal allele was substantially activated at 15 DAF (Figure 6C). These findings indicated that OsYUC11 expression was no longer monoallelic at 15 DAF. We believe that this is why the functional maternal OsYUC11 allele could recover the paternal defects in WT (♀)/osyuc11 (♂) seeds.
Polycomb Repressive Complex 2 (PRC2)-mediated trimethylation of Histone 3 at lysine 27 (H3K27me3) is important for imprinting regulation. OsFIE1 and OsEMF2a are members of PRC2 in rice. In reciprocal crosses of osfie1 (in the Kitaake background) and Yuetai (O. sativa ssp. Indica), we found that OsYUC11 expression showed paternal bias at 10 DAF (Figure 6D), indicating that the paternal expression bias of OsYUC11 was not significantly affected by OsFIE1 mutation. We also investigated OsYUC11 imprinting in the reciprocal crosses of osemf2a [in Zhonghua11 {O. sativa ssp. Japonica} background] and 9311 (O. sativa ssp. Indica). However, OsYUC11 expression was significantly downregulated in the endosperm cells of osemf2a (♀)/9311 (♂), and we therefore failed to unambiguously detect the parental expression bias. Nevertheless, the paternal expression preference remained unchanged in the endosperm cells of 9311 (♀)/osemf2a (♂).
Mutation of OsYUC9 and OsTAR1 exhibited similar effects on grain filling
OsYUC9 and OsYUC11 showed similar expression profiles in the developing caryopsis (Figure 1B). To determine whether OsYUC9 plays a similar role in grain filling, we generated osyuc9 mutants using a CRISPR/Cas9 approach. Three nonfunctional mutant alleles were obtained (Supplemental Figure S10A). Two of the mutant lines, osyuc9-2 and osyuc9-3, exhibited reduced seed weight, and all mutant lines (osyuc9-1, osyuc9-2, and osyuc9-3) showed increased chalkiness, although the phenotype was less severely manifested than that in osyuc11 (Figure 7, A and B). Similar to OsYUC11, OsYUC9 expression was downregulated in the osnf-yb1 mutants but upregulated in the OsNF-YB1OX line (Supplemental Figure S10, B and C), suggesting that OsYUC9 is possibly regulated by OsNF-YB1 as well. We also generated three osyuc12 mutant lines (Supplemental Figure S11A), which showed no significant changes in seed size or chalkiness (Supplemental Figure S11, B–D). Moreover, the timing of syncytium-cellularization transition in osyuc12 was not affected (Supplemental Figure S11, E–M), although OsYUC12 expression coincided with endosperm cellularization in rice.
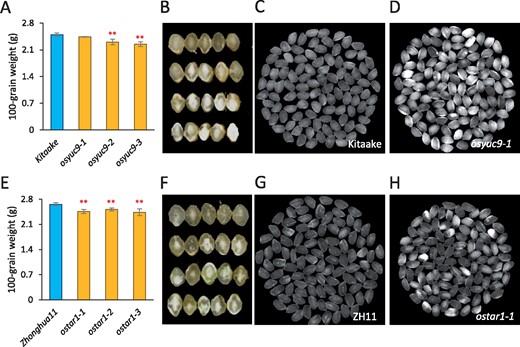
OsYUC9 and OsTAR1 are involved in the development of rice caryopsis. (A) Reduction of seed weight in the osyuc9 mutants. Three biological replicates were used for analysis. Error bars indicate standard deviation. Student’s t test was used for statistical analysis. **P<0.01. (B) Morphology of caryopses produced by the osyuc9 mutants. (C and D) Polished seeds of the WT (Kitaake) (C) and osyuc9-1 (D). (E) Reduction of seed weight in the ostar1 mutants. Three biological replicates were used for analysis. Error bars indicate standard deviation. Student’s t test was used for statistical analysis. **P<0.01. (F) Morphology of caryopses produced by the ostar1 mutants. (G and H) Polished seeds of the WT (ZH11) (C) and ostar1-1 (D).
OsTAR1 and OsTAR2 catalyzed the first step of auxin biosynthesis. OsTAR1 was predominantly expressed in the pollen and developing caryopsis (Supplemental Figure S1F). Compared with WT, the ostar1 strain produced smaller seeds with increased chalkiness (Zhonghua11, O. sativa ssp. japonica; Figure 7, E–H). These results indicate that grain filling in ostar1 was delayed, similar to that in osyuc9 and osyuc11. Collectively, these findings strongly suggest that auxin plays an important role in rice grain filling.
Discussion
The TAA–YUC pathway in plants is conserved for auxin biosynthesis (Zhao, 2014). In rice, the TAA1 mutant fish bone1 (fib1) exhibited pleotropic phenotypes due to impaired auxin biosynthesis (Yoshikawa et al., 2014; Guo et al., 2020). Knock-out or knock-down of rice YUCs led to developmental shoot and root defects (Woo et al., 2007; Yamamoto et al., 2007; Fujino et al., 2008). However, the exact mechanism by which auxin influences seed development in rice, especially grain filling, is not well understood. By analyzing the expression dynamics of rice YUCs during endosperm development, several studies found that OsYUC9, OsYUC11, and OsYUC12 were highly expressed in developing caryopsis (Abu-Zaitoon et al., 2012; Zhang et al., 2020). Here, we provide genetic evidence of YUC-mediated auxin biosynthesis for the regulation of rice grain filling.
Expressional regulation of OsYUC11 in the endosperm
Considering the phenotypic severity, we believe that OsYUC11 is the most important YUC in auxin biosynthesis in the endosperm. Grain filling in the osyuc11 strain was mostly halted from 5 DAF (Figure 2D). Storage product accumulation was also impaired in osyuc11 mutants. For example, the contents of storage proteins and lipids were increased in the caryopsis (Figure 2, Q and R). By screening a TF library, we identified 41 TFs that may bind to the OsYUC11 promoter (Supplemental Table S2). Specifically, we found that OsNF-YB1, a well-studied regulator of endosperm development (Bai et al., 2015; Xu et al., 2016; E et al., 2018; Bello et al., 2019), exhibited an expression profile similar to that of OsYUC11. Additionally, osyuc11 phenocopied osnf-yb1 in terms of reduced seed size and increased chalkiness (Figure 4B). Moreover, OsYUC11 expression or IAA biosynthesis was suppressed in the caryopsis of osnf-yb1 (Figure 4, D and E) but was upregulated in the OsNF-YB1-OX line (Figure 5, A and C).
Previous studies suggested that OsNF-YB1 regulates endosperm development through multiple pathways, including transcriptional activation of sucrose transporter genes such as SUT1, 3, and 4 (Bai et al., 2015) and the starch synthase gene Wx (Bello et al., 2019). OsNF-YB1 function usually involves coordination with other TFs (Xu et al., 2016; E et al., 2018; Xiong et al., 2019). Our findings strongly imply that OsYUC11 is regulated by OsNF-YB1 (Figure 8). Although in vitro experiments failed to detect the relevant binding and activation activities (Supplemental Figure S9, A–C), OsNF-YB1 was able to bind the OsYUC11 promoter to activate OsYUC11 in vivo (Figures 4D and 5, A–F). Considering that NF-Y family TFs usually form heterotrimers consisting of NF-YA/NF-YB/NF-YC for function (Laloum et al., 2013), we assumed that it requires other NF-Y family members to coordinate with OsNF-YB1 for OsYUC11 activation in rice plant.
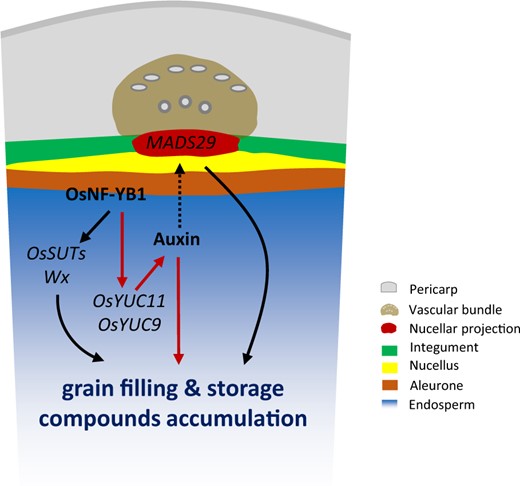
Schematic illustration of the auxin-regulated grain filling in rice. The auxin signals involved in the regulation of grain filling and storage compound accumulation of rice seeds. OsYUC11 and OsYUC9 are two YUC genes responsible for auxin biosynthesis in the endosperm. It is possible that the auxin synthesized in the endosperm is able to be transported to the maternal tissues, and modulate the expression of genes, like OsMADS29, an important regulator for rice filling that predominantly expresses in the nucellus and the nucellar projection. The expression of OsYUC11 is regulated by OsNF-YB1, a NF-Y TF member highly expressed in rice caryopsis. In addition to OsYUC11, the sucrose transporter genes OsSUT1, OsSUT3, and OsSUT4, and the key granule-bound starch synthase gene Wx are downstream genes directly regulated by OsNF-YB1.
In Arabidopsis, auxin synthesized in the endosperm can be exported to the maternal tissues for seed coat development (Figueiredo et al., 2016). OsMADS29 is a gene that is preferentially expressed in the nucellus and nucellar projection, and in a previous study, its inhibition resulted in slow grain filling and defective starch synthesis (Yin and Xue, 2012). OsMADS29 expression was shown to be induced by auxin (Yin and Xue, 2012). Therefore, auxin synthesized in the endosperm is potentially transported to the maternal tissues, as in Arabidopsis, and activates OsMADS29 expression for grain filling (Figure 8). On screening the TF libraries, we identified three MADS-box TFs, OsMADS14, OsMADS16, and OsMADS34, which were also able to bind to the OsYUC11 promoter (Supplemental Table S2). OsMADS14 and OsMADS34 showed expression patterns similar to that of OsYUC11 during the development of rice caryopsis (Figure 4A). In addition, OsMADS34 plays an important role in rice grain size and yield determination (Zhang et al., 2016). In addition to the MADS-box TFs, several other TF family members, such as the B3 and AP2 family TFs, could bind to the OsYUC11 promoter (Supplemental Table S2). Future studies should investigate how these TFs regulate OsYUC11.
Auxin-regulated endosperm cellularization in rice
Auxin is produced immediately after fertilization in Arabidopsis (Figueiredo et al., 2015). The taa1 tar1 tar2 triple mutant showed severe endosperm proliferation defects because of the retardation of auxin biosynthesis (Figueiredo et al., 2015). Cellularization failures induced by the over-proliferation of endosperm cells were associated with the overaccumulation of auxin in the paternal-excess triploid seeds of Arabidopsis, whereas overaccumulation of auxin in a diploid seed could phenocopy the endosperm defects (Batista et al., 2019). These findings suggest that auxin is an important cellularization signal.
Exogenous application of auxin accelerated maize endosperm cellularization, whereas auxin biosynthesis inhibitor delayed it (Lur and Setter, 1993a). These findings imply that auxin shows distinct functions for cellularization in monocots and dicots; it prevents cellularization in Arabidopsis but promotes cellularization in maize. The quantitative trait locus TGW6 of rice encodes an IAA-glucose hydrolase that converts IAA-glucose into free IAA in the developing caryopsis (Ishimaru et al., 2013). The accession Nipponbare (O. sativa ssp. japonica), which carries functional TGW6, exhibits higher endosperm IAA levels, and therefore, cellularization occurs earlier than in Kasalath (O. sativa ssp. indica), which carries a dysfunctional tgw6 allele.
Cellularization of rice is usually initiated at 3 DAF and is completed at 5 DAF depending on environmental conditions such as temperature (Chen et al., 2016; Wu et al., 2016). The transient expression of OsYUC12 coincided with cellularization in rice (Figure 1B). Similarly, we recently found that mutation of the PRC2 gene OsEMF2a in rice delayed cellularization but activated OsYUC12 expression in the endosperm (Cheng et al., 2020b). These findings imply that OsYUC12 is important for cellularization. However, cellularization in osyuc12 mutants remained unaffected (Supplemental Figure S11, E–M). This could be due to gene redundancy, because other YUCs, such as OsYUC7 and OsYUC8, were expressed in the caryopsis during the same stage (Supplemental Figure S12). Notably, OsYUC8 is a homolog of AtYUC1 and AtYUC4 (Zhang et al., 2018), which coordinates with AtYUC10 and AtYUC11 for embryogenesis in Arabidopsis (Cheng et al., 2007). Further investigation is required to determine whether OsYUC12 is involved in the cellularization of rice. OsYUC13 and OsYUC14 are homologs of OsYUC12 (Figure 1A). The expression of OsYUC13 and OsYUC14 was activated along with OsYUC12 in the endosperm of osemf2a (Cheng et al., 2020b). Generation of the osyuc12 osyuc13 osyuc14 triple mutant will be helpful in determining the importance of auxin in the cellularization in rice.
OsYUC11-mediated auxin biosynthesis plays a predominant role in grain filling
The activation of IAA biosynthesis coincides with the major starch production phase in both rice and maize (Basunia and Nonhebel, 2019). OsYUC9 and OsYUC11 are highly expressed during the grain filling stage. Both osyuc9 and osyuc11 mutants exhibited reduced seed weight and increased chalkiness (Figures 2, B and C and 7, A–D). However, the osyuc11 phenotype was more severely expressed than the osyuc9 phenotype, and ambient OsYUC9 was more abundantly expressed in the caryopsis than OsYUC11 (Figure 1B). Auxin biosynthesis was almost completely inhibited in the osyuc11 endosperm (Figure 3B). These results suggest that OsYUC11 plays a major role in auxin biosynthesis in the endosperm during grain filling. Similarly, ZmYUC1/De18 also predominantly regulates auxin biosynthesis in maize kernels. Free IAA in de18 was reduced to 1%–7% of that in WT (Bernardi et al., 2012). It remains unknown why OsYUC9 had a limited impact on endosperm development; this could be attributed to the redundancies between OsYUC9 and OsYUC10 due to the high sequence similarity (Figure 1A). YUC family members function in various auxin biosynthesis pathways (Zhao, 2014). Another possible explanation is that OsYUC9 may not be as active as OsYUC11 in catalyzing the conversion of IPA to IAA.
In maize, ZmYUC1 was significantly suppressed in the developing kernel of mn1 due to sugar availability (LeClere et al., 2010). OsYUC11 expression was not downregulated in grain incomplete filling 1, which is an equivalent mutant of mn1 in rice. However, OsYUC9 expression was markedly reduced (French et al., 2014). Considering the differences in expression, regulation, and phenotype of the endosperm-preferential YUCs of rice, we inferred that OsYUC9–14 functionally diverged during evolution.
Imprinting of OsYUC11 had limited impacts on grain filling
OsYUC11, AtYUC10, and ZmYUC1 serve as rare examples of conserved imprinting across monocots and dicots (Chen et al., 2018). However, the biological significance of imprinting at this locus remains unknown. OsYUC11 and AtYUC10 expression disorders have been found to be associated with endosperm abortion of interspecific or interploidy crosses (Schatlowski et al., 2014; Tonosaki et al., 2018). Maternal repression of OsYUC11 is likely caused by the hypomethylation and increase in H3K27me3 in the gene body of the maternal allele (Du et al., 2014). The paternal expression bias of OsYUC11 was impaired in the hybrid endosperm of O. sativa (♀)/O. longistaminata (♂; Tonosaki et al., 2018). PRC2 complex is essential for imprinting, especially for PEGs (Zhang et al., 2014; Moreno-Romero et al., 2016). OsFIE1, a maternally expressed gene that is exclusively expressed in the endosperm, is an essential PRC2 member of rice (Luo et al., 2009; Zhang et al., 2012; Cheng et al., 2020a). However, our results suggest that OsFIE1 has limited effects on OsYUC11 imprinting (Figure 7D). We also found that OsYUC11 imprinting is dynamic (Figure 6C). We believe that this was why a WT mother could rescue the paternal defects of OsYUC11 (Figure 6, A and B). For many imprinted genes, imprinting is usually a quantitative modification rather than an on/off switch (Hornslien et al., 2019). Therefore, weak expression of the maternal allele at an early stage may also contribute to the recovery of osyuc11 (♀)/WT (♂).
Materials and methods
Plant growth conditions and treatments
The experiments were conducted in the paddy fields of Yangzhou University (32°30′N, 119°25′E) in the summer season and in Hainan (18°48′N, 110°02′E) in the winter season. The same standard of water and nutrients were used throughout the course of the plants’ life. Rice (O. sativa) spikelets were labeled with a marker pen when flowering, and the caryopses were sampled at scheduled time points.
For NAA and NPA treatments, panicles of WT and osyuc11 were sprayed with 100-μM NAA and NPA, at a rate of ∼10 mL per plant, every other day since the day of flowering. The hormones were applied between 16:00 h and 18:00 h to avoid photolysis of the chemicals. NAA solution contained 0.8% (v/v) ethanol and NPA solution contained 1.6% (v/v) DMSO. The corresponding mock solutions contained the same concentrations of ethanol or DMSO dissolved in water. Each treatment consisted of 16 plants, and the seeds produced by the treated plants were harvested upon maturation for phenotyping.
For artificial hybridization, the spikelets of the maternal parent were emasculated the day before flowering. First, the top of each spikelet was cut off using scissors, and then each anther of a spikelet was gently removed using forceps. The panicles with the emasculated spikelets were then covered with crossing bags to transfer pollen from the desired paternal parent to the emasculated spikelets.
Vector construction and transformation
The OsYUC9, 10, and 12 mutants were generated via a CRISPR/Cas9 approach. The targets (Supplemental Table S3) were cloned into BGK032 using a CRISPR/Cas9 vector construction kit (Biogle). To generate the pYUC11-GUS transgenic line, the YUC11 promoter (approximately 2,000-bp upstream of the start codon) was amplified and transfected into vector1300-GN using the ClonExpress® II One Step Cloning Kit (Vazyme). The constructs were then transformed into rice calli as previously described (Chen et al., 2016).
Measurements of the storage compounds in caryopses
A starch quantification kit (DF-2-Y, Comin), glucose quantification kit (PT-2-Y, Comin), sucrose quantification kit (ZHT-2-Y, Comin), and fructose quantification kit (GT-2-Y, Comin) were used to quantify starch, glucose, sucrose, and fructose contents, respectively, in the caryopses at 5, 10, and 15 DAF according to the manufacturers’ instructions.
Ten-DAF-old WT and mutant caryopses were finely grounded using a mortar and pestle precooled with liquid nitrogen. The homogenized powders were then transferred to a 1.5-mL centrifuge tube containing protein extraction buffer [125-mM Tris/HCl pH7.5, 4-M urea, 4% {v/v} SDS, and 5% {v/v} β-mercaptoethanol]. Total protein was extracted in an incubator for 3 h with shaking at 220 r.p.m. at 37°C. The extracts were then centrifuged at 15,000 r.p.m. for 20 min at 4°C. The supernatants were subjected to gel electrophoresis using a 10% SDS–PAGE gel.
Sectioning and microscopy
The caryopses were fixed with formaldehyde-acetic acid-ethanol fixative solution and stored at 4°C for later use. This was followed by a gradient ethanol series and infiltration with xylene for embedding in resin. Samples were sliced in 2.5-μm-thick sections using a microtome (Leica EM) and stained with 0.1% (w/v) Toluidine Blue O or I2-KI solution (80 mg KI, 10 mg I2 per mL). The sections were then observed and photographed using a bright-field microscope (Olympus). For the observation of mature endosperm starch granules, the cross-sections of mature caryopses were sputter-coated with gold and observed using SEM (GeminiSEM 300, ZEISS).
RNA extraction and gene expression analyses
Total RNA from various tissues and young caryopses was extracted using the Plant RNA Kit (Omega). Total RNA of mature caryopses was extracted using the RNAprep Prue Plant Plus Kit (TANGEN). HiScript II Q Select RT SuperMix (Vazyme) was used for cDNA synthesis. The expression levels of each gene were measured by RT-qPCR using the CFX Connect Real-Time PCR Detection System (BioRad) and the AceQ qPCR SYBR Green Master Mix (Vazyme). The housekeeping gene Os03g63430 was used as an internal control. Three biological replicates were performed for each experiment. The primers used for RT-qPCR are listed in Supplemental Table S3.
RNA in situ hybridization
Digoxigenin-labeled in situ hybridization was performed as previously described (Yong et al., 2003), with a minor modification. The hybridization probes were amplified using the primers listed in Supplemental Table S3 and then cloned into pEASY-T3 vector (Transgene). A Digoxigenin RNA labelling kit (Roche) was used to label the probes according to the manufacturer’s standard protocol. Hybridization was performed at 42°C overnight using a solution as previously described (Yong et al., 2003). Labeled probes were detected using alkaline phosphatase coupled to anti-DIG antibody Fab fragments and 4-nitroblue tetrazolium chloride/5-bromo-4-chloro-3-indolyl-phosphate (Roche). Sections were dehydrated in an ethanol series and cleared in xylene after staining.
GUS staining
For GUS staining, the caryopses were stained using a staining buffer [0.6-mg/mL X-Gluc, 0.05-M PBS, pH 7.0, 0.05-mM potassium ferricyanide, 0.05-mM potassium ferrocyanide, 20% {v/v} methanol, and 0.1% {v/v{ Triton X-100] at 37°C in the dark for ∼8 h. The samples were then washed and cleared with a graded ethanol series before imaging.
Transcriptome analysis
RNA isolated from 10-DAF-old WT, osyuc11-1, and osyuc11-2 caryopses was submitted for RNA sequencing. Two biological replicates for each sample were used. Each replicate consisted of ∼20 caryopses. The samples were submitted to Novogene Co. Ltd. (Tianjin) for library preparation and sequencing using the Illumina Nova 6000 platform. We used CLC Genomics Workbench 12.0 software for short reads alignment and identification of DEGs with the settings previously described (Cheng et al., 2020a). The DEGs were then submitted to AgriGO (Du et al., 2010), an online tool for GO analysis, using the default settings.
Hormone extraction and quantification
The procedures were conducted as previously described (Cheng et al., 2020b). Approximately 0.2 g of caryopsis samples were finely ground in a precooled mortar containing 5 mL of extraction buffer composed of isopropanol/hydrochloric acid. The purified product was then subjected to high-performance liquid chromatography–tandem mass spectrometry using the Agilent 1290 platform.
Yeast one-hybrid assay
The OsYUC11 promoter region (−350 to −1) was amplified and cloned into XhoI-linearized pHisi-1-Bait using the ClonExpress® II One Step Cloning Kit (Vazyme). The bait gene was then integrated into the chromosome of yeast strain YM4271. The rice TF library was constructed by OE Biotech (Shanghai) and consisted of 1530 rice TFs. Strains containing bait and prey constructs were then mated in 96-well plates and screened using the selective medium SD/-Leu/-His/3-AT (3-amino-1,2,4-triazole, 30 mM). The yeast clones that survived after selection were sequenced, and the correct clones were used in a second round of point-to-point validation. If colonies still survived on the second set of selective medium, we concluded that the TF was able to bind to the OsYUC11 promoter.
ChIP analysis
Two grams of 2-week-old shoot tissue was harvested from WT and OsNF-YB1-overexpressing plants and then cross-linked in 1% (v/v) formaldehyde for 15 min at room temperature. The cross-linking reaction was then stopped by the addition of glycine (final concentration, 0.125 M). Chromatin was extracted and fragmented using sonication with an ultrasonic disruptor (XO-1000D, Xianou-Tech). ChIP using an OsNF-YB1 polyclonal antibody was performed using a Chip Kit-Plants kit (ab117137; Abcam). Because a previous study showed that OsNF-YB1 cannot bind to the promoter and coding regions of OsNF-YB1 but can bind to the promoter of Os01g63680, these genes were used as negative and positive controls, respectively. Chromatin immunoprecipitated with OsNF-YB1 antibody was compared with chromatin immunoprecipitated by mouse IgG (ab117137; Abcam] for enrichment analysis. All primers used for ChIP-qPCR assays are listed in Supplemental Table S3.
Phylogenetic analysis and expression clustering analysis
Alignments of the YUC protein sequences of rice and Arabidopsis were performed using Clustal Omega (https://www.ebi.ac.uk/Tools/msa/clustalo). Phylogenetic analyses were conducted using MEGA 7 (Kumar et al., 2016). A neighbor joining phylogenetic tree was constructed with default settings.
The expression data of the genes encoding TFs that showed binding abilities to the OsYUC11 promoter in yeast were retrieved from RiceXPro (Sato et al., 2013). The RXP_0012 (Embryo and Endosperm gene expression profile at ripening stage) dataset was used. Cluster analysis of gene expression was conducted using TBtools (Chen et al., 2020) with the PearsonDist method.
Statistical analysis
The data were exported to Microsoft Excel for statistical analysis (mean and standard error) and for graphing. Microsoft Excel was used for Student’s t test.
Accession numbers
OsYUC9, LOC_Os01g16714; OsYUC10, LOC_Os01g16750; OsYUC11, LOC_Os12g08780; OsYUC12, LOC_Os02g17230; OsYUC13, LOC_Os11g10140; OsYUC14, LOC_Os11g10170; OsNF-YB1, LOC_Os02g49410; OsTAR1, LOC_Os05g07720; OsGH3-2, LOC_Os01g55940; OsGH3-4, LOC_Os05g42150; OsPIN5b, LOC_Os08g41720; OsFIE1, LOC_Os08g04290.
Supplemental data
The following materials are available in the online version of this article.
Supplemental Figure S1. Expression of OsYUC9, 10, 11, and 12, and OsTAR1.
Supplemental Figure S2. Agronomic traits of osyuc11 plants.
Supplemental Figure S3. Expression of several starch biosynthetic genes in the developing caryopses of the WT and osyuc11-1.
Supplemental Figure S4. The sucrose, fructose, and glucose contents in the developing caryopses of the WT and osyuc11-1.
Supplemental Figure S5. The endosperm and embryo development of osyuc11.
Supplemental Figure S6. Physical and biochemical analyses of WT and osyuc11 starch.
Supplemental Figure S7. Quantitative measurements of storage proteins and lipids in the WT and osyuc11 caryopses.
Supplemental Figure S8. Relative expression of OsYUC9, OsYUC12, and OsNF-YB1 in the 10-d-old caryopses of WT and osyuc11.
Supplemental Figure S9. In vitro analyses of binding and activation ability of OsNF-YB1 to OsYUC11.
Supplemental Figure S10. Expression of OsYUC9 in OsNF-YB1 knock-out and overexpression lines.
Supplemental Figure S11. Phenotypic characterization of osyuc12 caryopses.
Supplemental Figure S12. OsYUC7 and OsYUC8 show expression in the endosperm of rice.
Supplemental Table S1. DEGs identified from the 10-DAF-old caryopses of the WT and osyuc11 mutants.
Supplemental Table S2. TFs showed binding affinity for the OsYUC11 promoter.
Supplemental Table S3. Primers used in the present study.
Acknowledgements
We thank Dr Hong-Xuan Lin of the Shanghai Institute of Plant Physiology and Ecology (SIPPE), Chinese Academics of Sciences, for providing the ostar1 mutants. We thank Dr Jian Zhang of the China National Rice Research Institute (CNRRI) for providing the OsNF-YB1OX line and the OsNF-YB1 antibody. We thank Dr Kewei Zhang of Zhejiang Normal University for assistance with auxin measurements.
Funding
This research was supported by grants from the National Key R&D Program of China (2016YFD0100902), the National Natural Science Foundation of China (31701392), the Science Fund for Distinguished Young Scholars of Jiangsu Province (BK20180047), and the Priority Academic Development of Jiangsu Higher Education Institutions.
Conflict of interest statement. None declared.
C.C. conceived the project. C.C., B.N., X.X., and D.Z. designed the research. X.X., Z.E., D.Z., Q.Y., Y.Z., and B.N. performed the experiments and analyzed the data. C.C. and X.X. wrote the manuscript.
The author responsible for distribution of materials integral to the findings presented in this article in accordance with the policy described in the Instructions for Authors (https://dbpia.nl.go.kr/plphys) is: Chen Chen ([email protected]).
References
Author notes
Xinyu Xu, Zhiguo E and Dongping Zhang authors contributed equally to this work
Senior author.