-
PDF
- Split View
-
Views
-
Cite
Cite
Paola Punzo, Alessandra Ruggiero, Marco Possenti, Giorgio Perrella, Roberta Nurcato, Antonello Costa, Giorgio Morelli, Stefania Grillo, Giorgia Batelli, DRT111/SFPS Splicing Factor Controls Abscisic Acid Sensitivity during Seed Development and Germination , Plant Physiology, Volume 183, Issue 2, June 2020, Pages 793–807, https://doi.org/10.1104/pp.20.00037
- Share Icon Share
Abstract
RNA splicing is a fundamental mechanism contributing to the definition of the cellular protein population in any given environmental condition. DNA-DAMAGE REPAIR/TOLERATION PROTEIN111 (DRT111)/SPLICING FACTOR FOR PHYTOCHROME SIGNALING is a splicing factor previously shown to interact with phytochrome B and characterized for its role in splicing of pre-mRNAs involved in photomorphogenesis. Here, we show that DRT111 interacts with Arabidopsis (Arabidopsis thaliana) Splicing Factor1, involved in 3′ splicing site recognition. Double- and triple-mutant analysis shows that DRT111 controls splicing of ABI3 and acts upstream of the splicing factor SUPPRESSOR OF ABI3–ABI5. DRT111 is highly expressed in seeds and stomata of Arabidopsis and is induced by long-term treatments of polyethylene glycol and abscisic acid (ABA). DRT111 knock-out mutants are defective in ABA-induced stomatal closure and are hypersensitive to ABA during seed germination. Conversely, DRT111 overexpressing plants show ABA-hyposensitive seed germination. RNA-sequencing experiments show that in dry seeds, DRT111 controls expression and splicing of genes involved in osmotic-stress and ABA responses, light signaling, and mRNA splicing, including targets of ABSCISIC ACID INSENSITIVE3 (ABI3) and PHYTOCHROME INTERACTING FACTORs (PIFs). Consistently, expression of the germination inhibitor SOMNUS, induced by ABI3 and PIF1, is upregulated in imbibed seeds of drt111-2 mutants. Together, these results indicate that DRT111 controls sensitivity to ABA during seed development, germination, and stomatal movements, and integrates ABA- and light-regulated pathways to control seed germination.
The phytohormone abscisic acid (ABA) regulates physiological and developmental processes, including stress responses, seed development, and germination. Perhaps the most well-defined mechanism mediated by ABA is induction of stomatal closure. In plants subjected to hyperosmotic stress, ABA is synthesized predominantly in leaf vascular tissues and guard cells. Here, ABA activates a signaling pathway that coordinately modulates activity of membrane-located transporters, leading to efflux of solutes. The consequent reduction of guard cell turgor causes stomatal closure, thus reducing evapotranspiration under abiotic stress conditions (Qin and Zeevaart, 1999; Schroeder et al., 2001; Nambara and Marion-Poll, 2005; Bauer et al., 2013; Kuromori et al., 2018).
In seeds, ABA induces maturation, dormancy, and plays a key role during germination. Transcription factors such as LEAFY COTYLEDON1 (LEC1) and LEC2, FUSCA3, and ABSCISIC ACID INSENSITIVE3 (ABI3) are involved in reserve accumulation and inhibition of premature germination (Santos-Mendoza et al., 2008, Mönke et al., 2012; Yan and Chen, 2017). At early stages of seed maturation, LEC1, LEC2, and FUSCA3 are expressed to prevent germination of the developing embryo, whereas ABI3 expression is maintained at high levels until final maturation stages (Perruc et al., 2007). In this phase, ABI3 and LEC1 regulate expression of genes involved in storage reserve accumulation and acquisition of desiccation tolerance, such as late embryogenesis abundant proteins (Parcy et al., 1994).
In addition, ABA prevents germination by inhibiting water uptake and endosperm rupture (Finch-Savage and Leubner-Metzger, 2006). When favorable conditions are restored, ABA levels decrease, with a concomitant increase of gibberellic acid (GA) to allow embryos to expand and break the seed-covering layers (Manz et al., 2005). The endogenous levels of ABA and GA are regulated by different signaling pathways, and recent studies have highlighted the crosstalk between light and hormonal pathways in the regulation of germination (Kim et al., 2008; Lau and Deng, 2010; de Wit et al., 2016). Phytochrome A (phyA) and phyB are photoreceptors that perceive Far Red (FR) and Red (R) light, respectively. During early stages of germination, phyB signaling involves a family of basic helix–loop–helix transcription factors, namely PHYTOCHROME INTERACTING FACTORs (PIFs). After R or white-light illumination, phyB translocates to the nucleus in its active Pfr conformation, where it binds and inhibits PIF1, also known as PIF3-LIKE5 (PIL5), promoting light-induced germination (Lee et al., 2012). In the dark or in low R/FR light, when phyB is in the inactive, Pr cytosolic form, PIF1 is stabilized and represses germination. PIF1 promotes ABA biosynthesis and signaling, and represses GA signaling, inducing expression of genes such as ABI3, ABI5, REPRESSOR OF GA1-3, and DOF AFFECTING GERMINATION1 (Oh et al., 2009). Interestingly, ABI3 protein also interacts with PIF1 to activate the expression of direct targets, such as SOMNUS (SOM), a key regulator of light-dependent seed germination acting on ABA and GA biosynthetic genes (Kim et al., 2008; Park et al., 2011).
In seeds initiating germination, ABI3 expression is repressed. Perruc et al. (2007) reported that the chromatin-remodeling factor PICKLE negatively regulates ABI3 by promoting silencing of its chromatin during seed germination. ABI3 activity is also controlled by alternative splicing (AS) of the corresponding precursor mRNA (pre-mRNA), with different splice forms predominating at different seed developmental stages. This process is regulated by splicing factor SUPPRESSOR OF ABI3-5 (SUA) through the splicing of a cryptic intron in ABI3 mRNA (Sugliani et al., 2010).
AS occurs when the spliceosome differentially recognizes the splice sites. The selection of alternative 5′ or 3′ splice sites leads to an inclusion of different parts of an exon, whereas failure to recognize splicing sites causes intron retention (IR) in the mature mRNA. These alternative splice forms can produce proteins with altered domains and function (Nilsen and Graveley, 2010; Staiger and Brown, 2013; Fu and Ares, 2014; Laloum et al., 2018). In plants, this mechanism is highly induced in response to external stimuli. Recent studies report an emerging link between splicing and ABA signaling (Zhu et al., 2017; Laloum et al., 2018). For example, the transcript encoding type 2C phosphatase HYPERSENSITIVE TO ABA1 (HAB1), a negative regulator of ABA signaling, undergoes AS. In the presence of ABA, the last intron is retained, leading to a truncated protein. The two encoded proteins, HAB1-1 and HAB1-2, play opposite roles by competing for interaction with OPEN STOMATA1 during germination, which then results in switching of the ABA signaling on and off (Wang et al., 2015). Likewise, SR45, a member of the Ser/Arg-rich proteins (an important class of essential splicing factors that influence splice site selection), regulates Glc signaling through downregulation of the ABA pathway during seedling development (Carvalho et al., 2010). In addition, several splicing regulators were reported to influence ABA sensitivity, such as SAD1, ABH1, SKB1, and Sf1 (Hugouvieux et al., 2001; Xiong et al., 2001; Zhang et al., 2011; Jang et al., 2014).
In this study, we show that the splicing factor DNA-DAMAGE REPAIR/TOLERATION PROTEIN111 (DRT111), previously characterized to play a role in the control of pre-mRNA splicing in light-regulated developmental processes (Xin et al., 2017), is involved in ABA response mechanisms. Manipulation of DRT111 expression results in a modified sensitivity to ABA regarding stomatal movements and seed germination. Accordingly, DRT111 is highly expressed in stomata and seeds, and upregulated upon long-term exposure to ABA. Moreover, ABI3 alternative transcript quantification as well as analysis of double and triple mutants shows that DRT111 controls splicing of ABI3 upstream of SUA. Transcriptome analysis in drt111 dry seeds revealed extensive alteration in gene expression and splicing of genes involved in light- and ABA-dependent control of germination. Consistently, we show that expression of the germination inhibitor SOM is induced in drt111. Taken together, our results suggest that DRT111 functions in the integration of ABA and light quality stimuli for seed germination under appropriate conditions
RESULTS
DRT111 Expression Is High in Seeds and Guard Cells and Is Induced by Long-Term Stress
Using RNA transcriptome data from potato (Solanum tuberosum) cells adapted to gradually increasing concentrations of polyethylene glycol (PEG), we identified Arabidopsis orthologous genes, and functionally analyzed their role in responses to ABA and osmotic stress (Ambrosone et al., 2015, 2017; Punzo et al., 2018). Following the same rationale, we focused on a DNA-damage-repair/toleration protein coding gene (EST617924, GenBank accession no. BQ510509, corresponding to PGSC0003DMT400054608, Potato Genome Sequencing Consortium, 2011; http://solanaceae.plantbiology.msu.edu/pgsc_download.shtml), upregulated in adapted cells (Ambrosone et al., 2017). The protein deduced from PGSC0003DMT400054608 shared 64% sequence identity with Arabidopsis At1g30480, encoding a predicted splicing factor (also referred to as DRT111//SPLICING FACTOR FOR PHYTOCHROME SIGNALING [RSN2/SFPS], SPF45-related; Supplemental Fig. S1; Pang et al., 1993; Zhang et al., 2014; Xin et al., 2017; ).
To verify the expression pattern in Arabidopsis, we mined public databases (eFP platform; Winter et al., 2007), which indicated that DRT111 is ubiquitously expressed throughout development, with highest transcript abundance in dry seeds (Fig. 1A). In addition, histochemical analysis of stable Arabidopsis lines expressing β-glucuronidase (GUS) under the control of the DRT111 promoter revealed GUS activity in cells surrounding the base of trichomes and guard cells (Fig. 1B).
![DRT111 promoter activity and gene expression. A, DRT111 tissue-specific expression based on Arabidopsis microarray data in the eFP browser (http://bar.utoronto.ca). Data are normalized by the GeneChip operating software (GCOS) method, target intensity value (TGT) of 100. B, Histochemical localization of GUS in leaves of transgenic Arabidopsis adult plants expressing the GUS reporter gene driven by the DRT111 promoter (DRT111promoter::GUS). C, Relative quantification (RQ) of DRT111 expression in 7-d–old seedlings after 3-, 6-, and 9-h exposure to NaCl (120 mm) or ABA (50 μm). D, RQ of DRT111 expression in 7-d-old seedlings after 5-d exposure to NaCl (120 mm), ABA (10 μm), or PEG (35% [w/v]). Data were normalized using RNA from untreated seedlings, and the elongation factor EF1a as endogenous control. Data reported are means ± sd of three biological replicates. The asterisks indicate significant differences compared to control conditions according to Student’s t test (*P ≤ 0.05 and **P ≤ 0.01).](https://oup.silverchair-cdn.com/oup/backfile/Content_public/Journal/plphys/183/2/10.1104_pp.20.00037/2/m_plphys_v183_2_793_f1.jpeg?Expires=1750215890&Signature=3wJbTagdnnYRAHL6rgId3o3AWqH2WDhqVqiZWJhMQzk37~wSLbc2NC07Za67vJOvsRDJVuHNfBP3W2JCty8J13cp6~rcji3WQRT69hO4IC7UsmYJvBAdSf319nVSfqihXgtvIY1fMG0BHIiw8MpBONYCownjh3kvPIq~~JKlz22VAiSSmhzSm2eiXEgkUONy5Os13L-kmQxU6oDImuBOnxnrsP3nCLPwM90vb4B-LkxDq8ggo4Nk2J-D2kFugwZu~KAYP4PTQChqCz~cmaiwBhlG683Yx3G~8rWpPDJZIRQYRS1q9QIqPZENXson-~vqWz3qngMOq53Wv-8ozIdjyw__&Key-Pair-Id=APKAIE5G5CRDK6RD3PGA)
DRT111 promoter activity and gene expression. A, DRT111 tissue-specific expression based on Arabidopsis microarray data in the eFP browser (http://bar.utoronto.ca). Data are normalized by the GeneChip operating software (GCOS) method, target intensity value (TGT) of 100. B, Histochemical localization of GUS in leaves of transgenic Arabidopsis adult plants expressing the GUS reporter gene driven by the DRT111 promoter (DRT111promoter::GUS). C, Relative quantification (RQ) of DRT111 expression in 7-d–old seedlings after 3-, 6-, and 9-h exposure to NaCl (120 mm) or ABA (50 μm). D, RQ of DRT111 expression in 7-d-old seedlings after 5-d exposure to NaCl (120 mm), ABA (10 μm), or PEG (35% [w/v]). Data were normalized using RNA from untreated seedlings, and the elongation factor EF1a as endogenous control. Data reported are means ± sd of three biological replicates. The asterisks indicate significant differences compared to control conditions according to Student’s t test (*P ≤ 0.05 and **P ≤ 0.01).
When assessing responsiveness of DRT111 in seedlings exposed to short- or long-term treatments of NaCl, ABA, or PEG, we detected a significant upregulation of DRT111 only after long-term treatments, whereas 3-, 6-, or 9-h treatments did not result in major changes of DRT111 transcript abundance. In particular, an increase higher than 2-fold was observed after 5-d treatments with ABA or PEG (Fig. 1, C and D). Taken together, the results show that DRT111 is highly expressed in seeds and guard cells, and that a higher steady-state mRNA level is determined by long-term exposure to ABA or osmotic stress.
DRT111/SFPS encodes a nuclear-localized potential ortholog of human splicing factor RBM17/SPF45 (Supplemental Fig. S2; Supplemental Materials and Methods; Xin et al., 2017), shown to interact with Splicing Factor1 (SF1), a protein involved in early prespliceosome assembly (Hegele et al., 2012; Crisci et al., 2015). Therefore, we investigated whether this interaction is conserved in Arabidopsis. Using the yeast two-hybrid system, we tested different portions of SF1, and demonstrated interaction between DRT111 and the C-terminal fragment (1,398–2,415) of SF1 (Fig. 2A), whereas a fusion of the GAL4 binding domain with the SF1 N-terminal fragment (1–1,396) resulted in autoactivation when cotransformed with the empty activation domain vector in yeast. We used a split reporter system to confirm the interaction in planta. A reconstituted YFP signal was detected in nuclear speckles, indicating that DRT111 forms a complex with SF1 and may thus act at early steps of the spliceosome machinery (Fig. 2B).
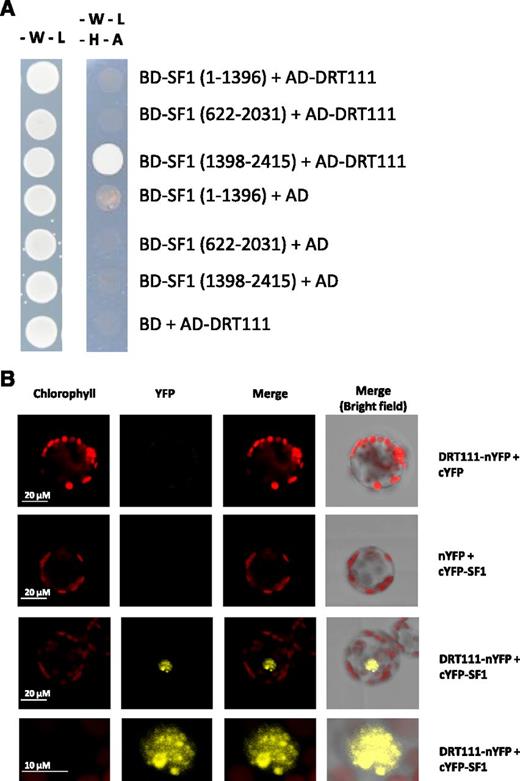
Interaction of DRT111 with SF1. A, Yeast two-hybrid assay. DRT111 in prey vector (pGADT7, activation domain) was cotransformed with the indicated fragments of SF1 cloned in the bait vector (pGBKT7, binding domain). The empty vectors pGADT7 and pGBKT7 were used as negative controls. Overnight grown yeast culture was dropped onto selective media lacking tryptophan (-W), leucine (-L), histidine (-H), and adenine (-A). Pictures were taken after 3-d incubation at 30°C. B, Bimolecular fluorescence complementation assay. Nicotiana tabacum leaf protoplasts were cotransformed with 20 μg each of plasmids encoding DRT111 fused with N terminus of YFP (nYFP) and SF1 fused with the C terminus of YFP (cYFP). nYFP and cYFP empty vectors were used as negative controls. The cells were imaged by confocal microscopy 16-h later. For the interaction, enlarged images of the nucleus are shown in the bottom row. Chlorophyll autofluorescence, YFP fluorescence, and merged images are shown.
Altered DRT111 Expression Affects Plant Growth and Stomatal Responsiveness to ABA
To analyze the involvement of DRT11 in ABA-related processes, insertion mutants were identified within The Arabidopsis Information Resource collection (https://www.arabidopsis.org/) and transgenic plants overexpressing DRT111 were produced (Supplemental Fig. S3, A–C). Three overexpressing (OX) lines carrying homozygous, single-copy transgene insertions and overexpressing the transcript were selected (Supplemental Fig. S3C). Phenotypic observations under control conditions and after ABA treatment indicated that overexpression of DRT111 caused a limited increase in primary root length (Fig. 3A), whereas lack of DRT111 expression resulted in early flowering, as previously reported (Supplemental Fig. S3D; Xin et al., 2017).
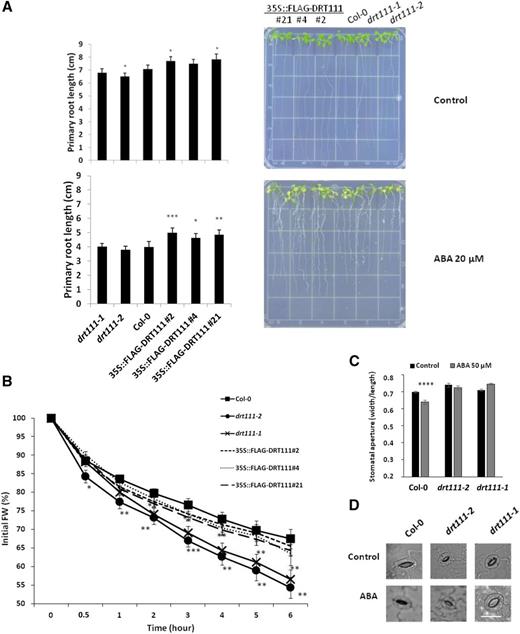
Phenotyping of DRT111 knockout mutants and OX lines. A, Primary root length of 10-d-old wild type (Col-0), drt111-1 (GABI_351E09), drt111-2 (SALK_001489), and DRT111 OX lines (35S::FLAG-DRT111 2, 4, and 21) grown on GM medium (1% Suc) or medium containing 20 μm ABA. Photos of representative plates (right), quantified as reported in graphs. B, Water loss of detached leaves of drt111 mutants, OX lines (35S::FLAG-DRT111), and wild-type (Col-0) plants. Data are averages ± se of two independent experiments (n = 5 for each line, per experiment) and reported as percentages of initial fresh weight at different time points (0.5–6 h). The asterisks indicate significant differences compared with wild type (*P ≤ 0.05, **P ≤ 0.01, and ***P ≤ 0.001, Student’s t-test). C, Stomatal aperture of drt111-1 and drt111-2 mutants and wild-type plants in response to ABA. Leaf peels harvested from 2-week-old plants were incubated for 2 h in SOS buffer under light and then treated with or without 50 μm ABA for 2.5 h. Asterisks indicate significant difference between samples with or without ABA (****P ≤ 0.0001, Student’s t-test). D, Photographs of stomata of the indicated genotypes as reported in (C). Scale bar = 25 μm.
As DRT111 is highly expressed in guard cells (Fig. 1B), we evaluated transpirational water loss and its relation to stomatal movements in plants with altered expression of DRT111. First, we measured the fresh-weight reduction of detached leaves during 6 h (Verslues et al., 2006). Whereas the OX and wild-type plants showed similar trends, we observed a significant increase in the transpirational water loss in knockout plants, with a loss of 44% and 46% of their initial fresh weight for drt111-1 and drt111-2, respectively, compared to 33% of Col-0 after 6 h (Fig. 3B).
Thus, we analyzed the stomatal movements in dtr111 plants compared to wild type after treatments with ABA, which plays a central role in stomatal closure (Fig. 3, C and D). Consistent with the water loss analysis, significant differences in stomatal pore size were observed between genotypes after 2.5-h ABA treatments (50 μm). Whereas ABA-induced stomatal closure was observed in Col-0 leaves (ratio 0.64 width/length of pore, corresponding to 8.5% reduction of the pore size), stomata of the mutants did not respond to the ABA treatment (drt111-1) or had a strikingly reduced response (drt111-2, ratio 0.72 width/length of pore, corresponding to 2.2% reduction compared to untreated stomata), suggesting that stomatal responsiveness to ABA is impaired in drt111 mutants, which causes significant water loss over time.
drt111 Seeds Are Hypersensitive to ABA during Germination
Because DRT111 is an ABA-responsive gene highly expressed in seeds (Fig. 1A), we analyzed seed germination of mutants and OX lines in the presence of ABA. As indicated in Figure 4, seeds collected from drt111-1 and drt111-2 were hypersensitive to ABA in terms of radicle emergence and cotyledon expansion, whereas OX lines displayed a rate of increased seed germination in the presence of ABA compared to wild type. In particular, after 3 d, 77% of Col-0 seeds were germinated on 0.5 μm ABA compared to 96%, 93%, and 93% of FLAG-DRT111 2, 4, and 21 seeds, and 53% and 57% of drt111-1 and drt111-2 seeds, respectively (Fig. 4, A–C). An ABA response curve of 12 months after-ripened seeds indicated that the hypersensitivity at high concentrations of ABA of the drt111 mutants was retained after long periods of dry storage (Fig. 4D). Complementation experiments of drt111 with DRT111 driven by the endogenous promoter indicated that the hypersensitivity to ABA observed in drt111-1 and drt111-2 can be reverted by introducing a functional DRT111 copy, thus confirming that the mutant phenotype is caused by the lack of functional DRT111 (Fig. 4E).
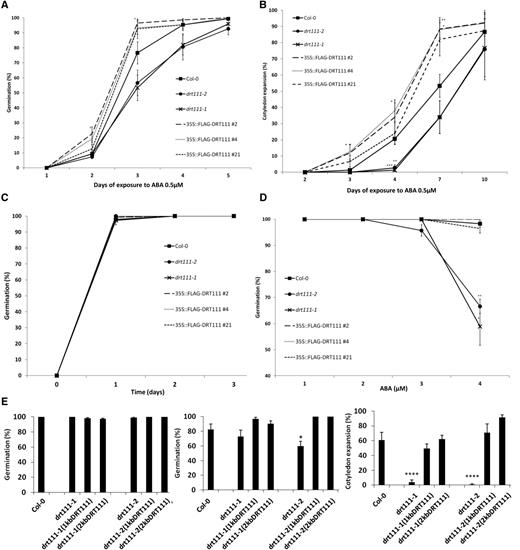
Germination analysis of DRT111 knockout mutants, DRT111 OX lines, and drt1111 mutant complemented lines. A to C, Seed germination of drt111 mutants (drt111-1, drt111-2), DRT111 OX lines (35S::FLAG-DRT111 2, 4, and 21), and wild-type (Col-0). Germination percentage of 10 d after-ripened seeds scored in terms of radicle emergence (A) and cotyledon expansion (B) in presence of 0.5 μm ABA and control media (C). Data are means ±sd (n = 150) of five biological replicates. D, Germination percentage of 1 year after-ripened seeds scored in terms of radicle emergence after 3 d in the presence of different concentrations of ABA. E, Germination analysis of drt111 mutants transformed with DRT111 genomic fragment including 1- or 2-kb upstream sequence from the translation start site (1KbDRT111; 2kbDRT111) in pDRT111::DRT111-FLAG constructs. Germination reported as percentage in terms of radicle emergence under control conditions (left) or in the presence of 0.5 μm ABA (middle), and in terms of cotyledon expansion in the presence of 0.5 μm ABA (right). In all germination tests, seeds were stratified for 2 d before incubation at 24°C. The asterisks indicate significant differences compared to Col-0 according to Student’s t-test (*P ≤ 0.05 and ****P ≤ 0.0001).
DRT111 Regulates Gene Expression and mRNA Splicing
To further characterize the role of DRT111 in seed germination, we examined the transcriptome of drt111 dry seeds. RNA-sequencing (RNA-seq) analysis highlighted a major role of DRT111 in the regulation of gene expression with >3,000 differentially expressed genes (DEGS; |log2 fold-change| > 0.21, false discovery rate [FDR] < 0.05), equally distributed among down- (1,941 genes) and upregulated (1,834) genes (Supplemental Dataset 1). Validation of a subset of genes by reverse transcription quantitative PCR (RT-qPCR) showed high correlation with the fold change detected by RNA-seq (Supplemental Fig, S4, C and D).
Consistent with the observed phenotype, gene-ontology (GO) enriched categories included seed-related processes (seed germination, embryo development ending in seed dormancy, postembryonic development), responses to abiotic stress (response to salt stress, response to cold, response to water deprivation, response to heat, regulation of stomatal movement, hyperosmotic salinity response, and response to osmotic stress), the ABA signaling pathway (response to ABA, ABA-activated signaling pathway), as well as the processing of mRNAs (mRNA processing, RNA splicing; Supplemental Dataset S1; Supplemental Fig. S4, A and B).
Among the genes differentially expressed in drt111-2, those encoding components of the light perception/signaling cascade were present, including Phytochromes and PIFs, some of which were upregulated (PhyA, PIF1/PIL5, and PIF6/PIL2) and others downregulated (PhyE, PhyD, and PIF7; Supplemental Dataset S1).
Significantly upregulated genes in drt111-2 included several members of the homeodomain Leu zipper class I TF (ATHB-1, ATHB-5, ATHB-7, and ATHB-12), which regulate abiotic stress responses.
To investigate the impact of a lack of DRT111 on pre-mRNA splicing, we explored differences in splicing events between drt111-2 and Col-0. Using the Multivariate Analysis of Transcript Splicing software (http://rnaseq-mats.sourceforge.net/), we analyzed all major types of splicing events, such as exon skipping (ES), alternative 5′ or 3′ splice site (A5SS; A3SS), mutually exclusive exon, and IR. All the analyzed events were affected in drt111-2 seeds. We identified a total of 611 differential splicing events, corresponding to 485 genes between mutant and wild type. Among these, A3SS and IR were the most represented categories, with 161 and 258 events, respectively (Fig. 5, A and B; Supplemental Dataset S2). Interestingly, gene ontology enrichment analysis (GOEA) showed that categories related to germination mechanisms; such as response to ABA, positive regulation of seed germination, ABA biosynthetic process, maintenance of seed dormancy by ABA, regulation of seed germination, and embryo sac egg cell differentiation; or to mRNA metabolism; such as mRNA splicing via spliceosome, mRNA processing, RNA splicing, and mRNA stabilization; were significantly enriched among the IR and A3SS defects in drt111-2. (Supplemental Dataset S3), suggesting that DRT11 may control the splicing of specific mRNAs in seeds. We validated the splicing events identified through RNA-seq and reported as reads mapped in gene regions in Col-0 and drt111-2 mutant (Fig. 5, C, E, G, and I) by RT-qPCR analysis (Fig. 5, D, F, H, and J).
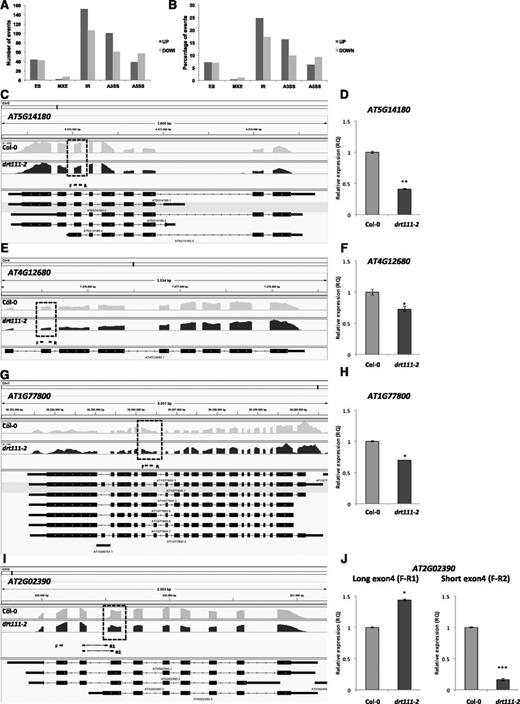
AS events altered in drt111-2. A, Number of different AS events upregulated (a greater prevalence of AS event in the mutant versus Col-0) and downregulated (a lower prevalence of AS event in the mutant versus Col-0) in drt111-2 dry seeds. B, Percentage of splicing events significantly up- and downregulated in drt111-2 with respect to the total AS events defective in drt111-2. MXE, Mutually exclusive exon; IR, Intron retaining; A3SS, Alternative 3′ splice site; A5SS, Alternative 5′ splice site. C to J, Validation of RNA-seq data by RT-qPCR. C, E, G, and I, Representation of AS differences between Col-0 and drt111-2 detected by RNA-seq using the Integrative Genomics Viewer (https://software.broadinstitute.org/software/igv/). Dashed box indicates the position of AS events: ES (AT5G14180, AT4G12680), IR (AT1G77800), and A3SS in (AT2G02390). Primers used for RT-qPCR are shown. D, F, H, and J, Validation by RT-qPCR. The elongation factor EF1α was used as endogenous control. Data reported are means ±sd of three biological replicates (n = 3). The asterisks indicate significant differences compared to control conditions according to Student’s t-test (*P ≤ 0.05 and **P ≤ 0.01).
DRT111 Regulates ABI3 Splicing
We have shown that drt111 mutants are hypersensitive to ABA in the germination process (Fig. 4). One of the key players determining sensitivity to ABA at the seed stage, and whose activity is regulated by AS, is the transcription factor ABI3 (Sugliani et al., 2010). The ABI3 locus gives rise to two alternative transcripts, ABI3-α and ABI3-β, which differ by the presence or cleavage of a cryptic intron, respectively. ABI3-α produces a full-length, functional protein and is highly expressed during seed development, whereas ABI3-β encodes a truncated protein lacking two of the four ABI3 conserved domains, and accumulates at the end of seed maturation (Sugliani et al., 2010).
Although splicing of ABI3 was not identified as affected in drt111-2 through RNA-seq, comparison of the DEGs with a list of 98 ABI3 targets (Mönke et al., 2012) showed that 51 of these genes were deregulated in drt111-2 (Supplemental Table S1), suggesting that ABI3 might be a target of DRT111.
Therefore, we used RT-qPCR to quantify the amount of ABI3-α and ABI3-β in drt111-2 dry and imbibed seeds compared to wild type using primers described by Sugliani et al. (2010). Although the level of ABI3-α is similar in dry seeds, accumulation of ABI3-β is significantly higher in drt111-2 than Col-0 (Fig. 6A). In addition, in imbibed seeds, both transcripts were upregulated in drt111-2, with ABI3-β showing a 4-fold induction compared to Col-0 (Fig. 6A), demonstrating a defective regulation of ABI3 splicing in plants lacking DRT111.
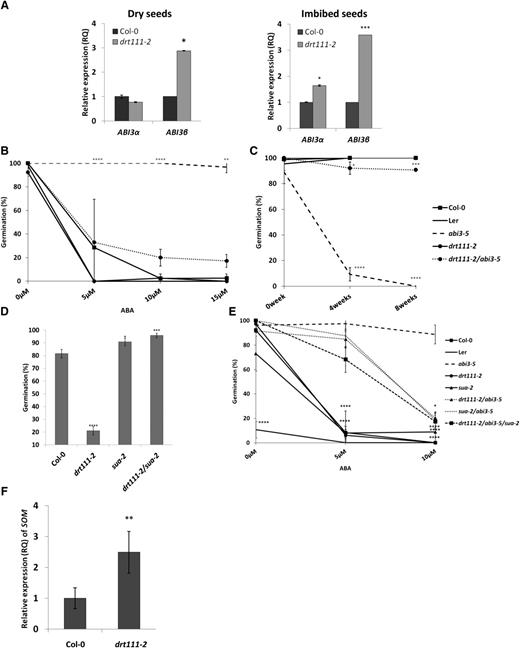
Germination analysis and relative expression of ABI3 and SOM in drt111-2 seeds. A, Relative quantification (RQ) of expression of ABI3 splicing variants (ABI3-α, ABI3-β) in Col-0 and drt111-2 dry seeds (left) or imbibed seeds (right). Data reported are means ±sd of three biological replicates (n = 3). B, Germination of freshly harvested seeds sown on media containing 0, 5, 10, or 15 μm ABA. C, Germination of seeds sown on GM medium after different periods of dry storage: 0, 4, or 8 weeks after harvest. B and C, Data were collected after 3 d and reported as means of three biological replicates (±sd). D, Germination of 14 d after-ripened seeds sown on medium containing 0.5 μm ABA. Data were collected after 3 d and reported as means of two independent experiments (±se). E, Germination of freshly harvested seeds sown on medium containing different concentrations of ABA (0, 5, or 10 μm). Data were collected after 7 d and reported as means of three biological replicates (±sd). F, Expression of SOM in Col-0 and drt111-2 imbibed seeds. In all germination tests, seeds were stratified for 2 d before incubation at 24°C. The asterisks indicate significant differences compared with wild type or abi3-5 (E) according to Student’s t-test (*P ≤ 0.05, **P ≤ 0.01, ***P ≤ 0.001, and ****P ≤ 0.0001).
To confirm this observation genetically, we took advantage of the abi3-5 mutant allele (Ooms et al., 1993). Due to a frameshift mutation, the abi3-5-α transcript contains a premature stop codon, whereas abi3-5-β encodes a functional ABI3 protein, and therefore an increase in accumulation of abi3-5-β results in a higher amount of functional ABI3 (Sugliani et al., 2010). Thus, an increased efficiency in splicing of the cryptic intron is expected to alleviate abi3-5 phenotypes, including ABA insensitivity during germination and reduced seed longevity (Bies-Etheve et al., 1999).
We thus produced double mutants drt111-2/abi3-5 to verify reversion of the abi3-5 phenotypes. Germination tests showed increased sensitivity to ABA and improved longevity of drt111-2/abi3-5 as compared to abi3-5. In the presence of 10 μm of ABA, 20% of drt111-2/abi3-5 seeds were able to germinate compared to 100% in abi3-5 (Fig. 6B). Moreover, the severe reduction of longevity observed in abi3-5 seeds was restored in drt111-2/abi3-5, with 90% of seeds able to germinate 8 weeks after harvest compared to 0% abi3-5 (Fig. 6C). Altogether, these results show that mutations in DRT111 rescue the abi3-5 mutation, with knock-out mutations showing a higher efficiency of phenotype reversion.
Because the AS of ABI3 is also controlled by the splicing factor SUA (Sugliani et al., 2010), we verified genetic interaction between DRT111 and SUA by the analysis of the double mutants drt111-2/sua-2. As shown in Figure 6, seed germination on 0.5 μm of ABA of drt111-2/sua-2 was 96% compared to 20% of drt111-2 and 91% of sua-2. Thus, these results indicate that SUA is epistatic to DRT111 and that DRT111 acts upstream of SUA (Fig. 6D).
Accordingly, germination of the drt111-2/sua-2/abi3-5 triple mutant did not show additive effects compared to drt111-2/abi3-5 or sua-2/abi3-5 double mutants. Germination rates of the triple mutant on higher ABA concentrations largely resembled that of double mutants, with 17% drt111-2/sua-2/abi3-5 seeds germinated on 10 μm of ABA compared to 20% drt111-2/abi3-5 and 18% sua-2/abi3-5, indicating that control of ABI3 splicing by DRT111 and SUA is exerted through the same pathway (Fig. 6E).
Expression of SOM Is Affected in drt111
In imbibed seeds, ABI3 and PIL5/PIF1 collaboratively activate the expression of the germination inhibitor SOM (Park et al., 2011), whereas R or white light repress it through the action of PhyB. Because DRT111 controls splicing of ABI3, and is epistatic to PIFs (Xin et al., 2017), we verified expression of SOM in imbibed seeds of drt111-2. As shown in Figure 6F, expression of SOM was 2.49-fold higher in drt111-2 compared to wild-type Col-0, indicating that a higher expression of SOM might contribute to the observed ABA hypersensitivity in drt111 seeds. Consistently, pil5/pif1 and the quadruple pif1/pif3/pif4/pif5 mutant are insensitive to ABA in seed germination (Supplemental Fig. S5, B and C; Supplemental Materials and Methods; Oh et al., 2009; Lee et al., 2012). Finally, we also established that the phyB mutant displayed hypersensitivity to ABA in the germination process (Supplemental Fig. S5A). Again, this could be partly due to the lack of negative regulation of SOM acting as a positive regulator of ABA biosynthesis (Kim et al., 2008).
DISCUSSION
AS and its regulation are involved in several adaptation processes in response to environmental stimuli and stresses (Laloum et al., 2018). Here, we have shown that the Arabidopsis DRT111 gene, encoding a protein orthologous to the human splicing factor SPF45 (Xin et al., 2017), is highly expressed in dry seeds, stomata, and in seedlings experiencing long-term osmotic stress. Functional studies in Arabidopsis established that DRT111 controls stomatal movements and seed germination in response to ABA.
The human splicing factor SPF45 forms a complex with SF1 and U2AF65 for the selection of alternative 3′ splice sites (Lallena et al., 2002). During early spliceosome assembly, the U2AF65 interact with U2AF35 and SF1 to promote U2snRNP detection of the pre-mRNA 3′ splice site (Park et al., 2017).
Here we have shown that DRT111 physically interacts with SF1, whereas in a previous independent study, interaction and colocalization with U2AF65 was reported (Xin et al., 2017). Based on homology with yeast and metazoan proteins, plant SF1 may be involved in recognition of intron branching point and assist in 3′ splice site selection (Jang et al., 2014; Lee et al., 2017). Together with the observation that the highest number of the observed splicing defects concerned IR and A3SS, the protein interactions suggest that DRT111 is also involved in the early steps of spliceosome formation, which concern intron branch point recognition and 3′ splice site selection by U2AF and SF1. However, the mode of participation of DRT111 (e.g. promotion and/or suppression) in this complex remains to be established.
A growing body of evidence indicates that in plants, components of the pre-mRNA splicing machinery modulate responses to ABA and abiotic/biotic stresses (Xiong et al., 2001; Cui et al., 2014; Carrasco-López et al., 2017). Arabidopsis sf1 mutants show several developmental defects, including dwarfism, early flowering, and hypersensitivity to ABA at the seed germination stage (Jang et al., 2014).
Previously, DRT111 and SUA were identified in a suppressor screen of snc4-1d, mutated in a receptor-like kinase involved in bacterial pathogen resistance (Zhang et al., 2014). A similar pattern of IR in SNC4 and CERK1 was reported in both sua and drt111 plants, thus suggesting that SUA and DRT111 are both required for the splicing of at least these two genes (Zhang et al., 2014).
Here, we have shown that DRT111 knock-out and OX plants are impaired in ABA seed germination responses, in analogy to sua mutants (Sugliani et al., 2010). In particular, SUA controls the activity of ABI3 by suppressing the splicing of an ABI3 cryptic intron to reduce the levels of functional ABI3 in mature seeds (Sugliani et al., 2010). Because the ABI3 cryptic intron is part of a protein-coding exon, it was subsequently classified as an exitron, an alternatively spliced internal region of a protein-coding exon (Marquez et al., 2015). Exitron splicing (EIS) is suggested to be a mechanism to increase plant proteome diversity in specific developmental stages or stress conditions, to affect protein functionality by modifying intracellular localization, presence of protein domains, and posttranslational modification sites, such as phosphorylation, sumoylation, and ubiquitylation (Marquez et al., 2015). Based on EIS patterns in sua mutants, and presence of RBM5/SUA predicted binding sites enrichment in exitrons, SUA appears to have a general role in preventing EIS (Marquez et al., 2015).
Here, we have shown that DRT111, similarly to SUA, suppresses splicing of ABI3. Accordingly, known ABI3 targets (Mönke et al., 2012) were found to be differentially expressed in drt111 compared to wild type. Interestingly, sua mutants in Columbia background are insensitive to ABA in seed germination (Sugliani et al., 2010), whereas DRT111 knock-out causes ABA hypersensitivity. The phenotype in drt111 may be explained by the observed increase in total ABI3 amount, determined in imbibed seeds by an increase of both the alpha and the beta transcripts. In particular, a 4-fold accumulation of ABI3-β, corresponding to the transcript in which the exitron is spliced out, accounts for most of ABI3 transcript in drt111-2. Therefore, the different ratio between the ABI3-α and ABI3-β transcripts, and their products thereof, may be important to define seed ABA sensitivity.
Both sua/abi3-5 or drt111-2/abi3-5 in Columbia background partially rescue seed developmental and ABA sensitivity defects of abi3-5. Thus, similarly to mammalian systems, SUA and DRT111 may control splicing of the same substrates with different timing. Further analyses will verify if DRT111 also controls the EIS mechanism.
DRT111/SFPS was recently shown to regulate development in response to light through interaction with phyB and REDUCED RED-LIGHT RESPONSES IN CRY1CRY2 BACKGROUND1 (Xin et al., 2017, 2019). In vegetative tissues, DRT111 regulates pre-mRNA splicing of genes involved in light signaling and the circadian clock and acts upstream of PIFs, a major class of phyB targets (Xin et al., 2017). Interestingly, we observed differential expression of PIF1/PIL5, PIF6/PIL2, and PIF7 in dry seeds of drt111-2. In particular, PIF1/PIL5 and PIF6/PIL2 were upregulated and PIF7 was slightly downregulated.
PIF1 inhibits GA signaling by promoting expression of DELLA repressors and, indirectly, by reducing GA levels (Oh et al., 2007; Paik et al., 2017). Indeed, upregulated expression of GIBBERELLIC ACID INSENSITIVE and REPRESSOR OF GA1-3-LIKE2 observed in drt111-2 seeds could also be dependent on increased PIF1 activity or expression (Lee et al., 2012).
In the dark, or in response to low R/FR light, PIF1 inhibits seed germination through activation of hormone-dependent, germination-inhibiting mechanisms, including the induction of ABA biosynthesis and signaling genes (Oh et al., 2009). This process is partly regulated by the action of SOM (Kim et al., 2008; Park et al., 2011), which in turn regulates MOTHER-OF-FT-AND-TFL1 (Vaistij et al., 2018). This may be achieved through induction of expression and interaction with ABI3 and ABI5, which may assist PIF1 in target site selection and activation of transcription (Kim et al., 2008, 2016; Park et al., 2011). Here, we have shown that expression of SOM is upregulated compared to wild type in drt111-2 imbibed seeds. Thus, regulation of SOM appears to be a major point of convergence between light and hormonal stimuli and DRT111 may be involved in this signal integration by exerting a regulatory function on both ABI3 and PIF1.
Because phyB-9 seeds are hypersensitive to ABA and it has been previously shown that phyB plants maintain open stomata under stress conditions, similarly to what we observed in drt111 mutants (González et al., 2012), we cannot exclude that the light perception by phyB is involved in DRT111-dependent splicing events.
Finally, the transcriptomic analysis identified several genes whose expression/splicing is affected in drt111-2, therefore, several other key factors may contribute to the observed ABA hypersensitivity in drt111. Among them, genes highly expressed in drt111-2 included several members of the homeodomain Leu zipper class I TF (ATHB-1, ATHB-5, ATHB-7, and ATHB-12), which have been largely studied for their role as regulators of abiotic stress responses. ATHB-7 and ATHB-12 are induced by water stress and ABA and control expression of several members of clade A PP2Cs, and are therefore considered negative regulators of ABA and stress responses (Arce et al., 2011; Valdés et al., 2012; Sessa et al., 2018). By contrast, ATHB-5, whose expression is positively regulated by ABI1, ABI3, and ABI5, is considered a positive regulator of ABA signaling because enhanced levels of ATHB-5 result in elevated ABA responses (Johannesson et al., 2003). ATHB-1, in particular, was shown to be regulated at the expression level by PIF1/PIL5 and is involved in hypocotyl growth regulation in short days (Capella et al., 2015). Future work will analyze the molecular details of the regulation operated by DRT111 on its targets.
AS defects observed in drt111 concerned predominantly IR and A3SS. Other splicing effectors and regulators affecting stress responses regulate these two AS classes. An increased splicing efficiency of IR-prone introns was shown to be important for acclimation to drought stress and splicing regulator HIN1 is involved in this process (Chong et al., 2019). Similarly, SAD1 splicing factor increased A3SS usage under salt-stress conditions (Cui et al., 2014). How DRT111 and components/regulators of the spliceosome, including SUA, SF1, and U2AF65, associate/compete to determine the splicing of specific transcripts will be important to establish the contribution of this layer of regulation in defining the proteome during ABA and stress responses.
In conclusion, the evidence shown here combined with that previously reported shows that DRT111 constitutes a point of integration between light- and ABA-dependent signaling by controlling expression and splicing of key factors such as ABI3 and PIF transcription factors.
MATERIALS AND METHODS
Plant Materials, Growth Conditions, and Germination Assays
The Columbia (Col-0) and Landsberg ecotypes were used as wild type. The drt111 T-DNA insertion mutants drt1111-1 (GABI_351E09) and drt111-2 (SALK_001489) were obtained from the Nottingham Arabidopsis Stock Centre. sua-2 and sua-2/abi3-5 were kindly donated by Wim Soppe (Max Planck Institute for Plant Breeding Research). abi3-5 was donated by Dr. Lucio Conti (University of Milan). Arabidopsis (Arabidopsis thaliana) plants were grown on soil in a growth chamber (14-h light/10-h dark) at 24°C. For germination tests, seeds harvested the same day from plants grown in parallel and stored for the same time were compared. Freshly harvested seeds or seeds dry stored (after-ripened) for different times as indicated in figure legends were used. Seeds were sown on germination (GM) medium (1× Murashige and Skoog salts and 0.5% [w/v] Suc, pH 5.7) or medium containing different concentrations of ABA (0.5, 2, 5, or 10 μm). After stratification treatment at 4°C for 2 d, seeds were transferred to a growth chamber (16-h light/8-h dark) at 24°C. Germination percentage was evaluated in terms of radicle emergence or fully expanded cotyledons. Gene expression analysis was carried out using 7-d–old seedlings grown on GM plates and then transferred to GM or GM containing NaCl (120 mm) and ABA (50 μm) for 3, 6, and 9 h, or NaCl (120 mm), ABA (10 μm), or PEG (35% [w/v]) for 2 and 5 d.
RNA Extraction, cDNA Synthesis, and RT-qPCR
Total RNA was isolated from 100 mg of seedlings using RNeasy Plant Mini Kit (Qiagen) according to manufacturer’s instructions. For RNA deep sequencing and RT-qPCR, total RNA was extracted from 100 mg of dry seeds or imbibed seeds (incubated in water, 24 h in darkness, 4°C) using a method reported by Oñate-Sánchez and Vicente-Carbajosa (2008). Complementary DNA (cDNA) was synthesized using the QuantiTect Reverse Transcription Kit (Qiagen), starting from 1 μg of DNase-treated RNA. For RT-qPCR, 4.5 μL of diluted (1:20) cDNA was used for each reaction, with 6.25 μL of 1× Platinum SYBR Green qPCR SuperMix (Life Technologies) and 1.75 μL of primer mix (5 μm). PCR was performed using 7900HT (Applied Biosystems). Cycling conditions were: 10 min at 95°C followed by 40 cycles of 95°C for 15 s and 60°C for 1 min. Three biological replicates, each with three technical replicates, were tested. The relative quantification of gene expression was calculated based on the 2−ƊƊCt method (Livak and Schmittgen, 2001). The elongation factor EF1α was used as endogenous reference gene and RNA isolated from control plants as calibrator sample. Primers used are listed in Supplemental Table S2.
Generation of DRT111 Transgenic Plants
Transgenic Arabidopsis plants were produced using binary vectors obtained by Gateway Technology (Life Technologies). To study promoter activity, the sequence of DRT111 promoter (2-kb upstream sequence from the start codon) was amplified from genomic DNA of Col-0 plants. To permit both N-terminal and C-terminal fusion with tags, the coding sequence of DRT111 was amplified with or without a STOP codon. For the complementation of drt111 mutants, genomic sequences of DRT111 including the upstream 1-kb or 2-kb region were amplified. Primers used are listed in Supplemental Table S2. PCR amplifications were performed using Phusion DNA polymerase (Thermo Fisher Scientific). The amplicons were cloned into pDONR207 (Life Technologies) using BP Clonase (Life Technologies) to obtain entry vectors.
Recombination with destination vectors was performed using LR Clonase (Life Technologies). pMDC164 (Curtis and Grossniklaus, 2003) was used for promoter studies, pGWB411 and pGWB412 (Nakagawa et al., 2007) was used to produce FLAG-tagged OX plants, and pEG302 (Earley et al., 2006) was used for mutant complementation. The resulting recombinant binary vectors were then introduced into the Agrobacterium tumefaciens GV3101 strain, which was then used to transform Col-0 plants or drt111 mutants using the floral dip method (Clough and Bent, 1998).
GUS Assay
Histochemical analysis of GUS activity was performed as described in Batelli et al. (2012). The tissues from transgenic Arabidopsis plants transformed with DRT111promoter::GUS construct were washed in 70% (v/v) ethanol, and cleared with chloralhydrate/glycerol solution. Samples were analyzed and photographed under an Axioscope 2 Plus microscope (Zeiss) equipped with a Coolpix 990 camera (Nikon).
Stomatal Measurements
Detached leaves from 4-week–old plants were used for stomatal measurements. For stomatal aperture assay, epidermal peels were floated in SOS solution (20 mm KCl, 1 mm CaCl2, and 5 mm MES-KOH [pH 6.15]) for 2.5 h under light to induce stomatal opening. Then, the buffer was replaced with fresh SOS containing 50 μm of ABA or fresh SOS without ABA and incubated under light for 2.5 h. One-hundred stomata were randomly observed using a DMR microscope (Leica). The widths and lengths of stomata pores were measured using the software Image J (https://imagej.net/).
Yeast Two-Hybrid Assay
For the yeast two-hybrid assay, the coding sequence of DRT111 was cloned between the BamH1 and XhoI restriction sites of pGADT7 vector (Clontech) and the cDNA fragments of SF1 were cloned between the SmaI and SalI sites of pGBKT7 (Clontech) using primers listed in Supplemental Table S2. To evaluate the interaction between DRT11 and the different SF1 fragments, the obtained constructs were cotransformed into Saccharomyces cerevisiae AH109 strain using the LiAc-mediated transformation method (Bai and Elledge, 1996) and plated on synthetic defined (SD) medium (7.5 g L−1 Yeast Nitrogen Base, 0.75 g L−1 amino acid mix, and 20 g L−1 Glc, pH 5.8) lacking Leu and Trp. Yeast cultures were grown overnight and an equal amount was dropped on SD lacking Leu and Trp medium to guarantee the presence of both vectors, and onto SD medium lacking Leu, Trp, Ade, and His to verify the protein–protein interaction (Ruggiero et al., 2019). Empty vectors pGBKT7 and pGADT7 were used as negative controls.
Bimolecular Fluorescence Complementation Assay
The coding sequences of DRT111 and SF1 were cloned by Gateway technology in the pUGW2 and pUGW0 vectors (Nakagawa et al., 2007) to guarantee the downstream fusion of the C-terminal YFP region and upstream fusion of N-terminal YFP region, respectively. Primers are listed in Supplemental Table S2. Nicotiana tabacum leaf protoplasts were prepared and transfected according to Pedrazzini et al. (1997). Forty micrograms of DNA for each construct was introduced in 1 × 106 protoplasts using PEG-mediated transfection. After a 16-h incubation in the dark at 25°C, the cells were imaged with an Inverted Z.1 microscope (Zeiss) equipped with a LSM 700 spectral confocal laser-scanning unit (Zeiss). Samples were excited with a 488-nm, 10-mW solid laser with emission split at 505 nm for YFP and excited with a 555-nm, 10-mW solid laser with emission split at 551 nm for chlorophyll detection.
RNA-Seq Analysis
For RNA deep sequencing, total RNA was extracted from dry seeds and DNAse-treated using an RNAeasy Plant Kit (Qiagen). Three biological replicates per genotype (Columbia-0 and drt111-2) were used. Library construction was performed using the TruSeq RNA Sample Preparation Kit (Illumina) before sequencing in single (2 × 100, ∼45,000,000 total reads/sample) on the platform HiSeq 2500 (Illumina). The sequencing service was provided by Genomix4life (http://www.genomix4life.com) at the Laboratory of Molecular Medicine and Genomics (University of Salerno). Raw sequences are deposited in the National Center for Biotechnology Information Sequence Read Archive, bioproject PRJNA605166. Before further analysis, a quality check was performed on the raw sequencing data by using FastQC (https://www.bioinformatics.babraham.ac.uk/projects/fastqc/), then low-quality portions of the reads were removed with BBDuk (sourceforge.net/projects/bbmap/). The minimum length of the reads after trimming was set to 35 bp and the minimum base quality score to 25. The high-quality reads were aligned against the Arabidopsis reference genome sequence (Araport11; http://www.araport.org/) with the STAR Aligner tool (v2.5.0c; Dobin et al., 2013). FeatureCounts (v1.4.6-p5; Liao et al., 2014) was used together with the most recent Arabidopsis annotation to calculate gene expression values as raw read counts. Normalized Trimmed Means of M-values and fragments per kilobase of exon model per million reads mapped values were calculated. All the statistical analyses were performed with R with the packages HTSFilter (Rau et al., 2013) and edgeR (Robinson et al., 2010). The first step was the removal of not-expressed genes and the ones showing too much variability. The software package HTSFilter was chosen for this scope, which implements a filtering procedure for replicated transcriptome sequencing data based on a Jaccard similarity index. The “Trimmed Means of M-values” normalization strategy was used. The filter was applied to the different experimental conditions to identify and remove genes that appear to generate an uninformative signal. The overall quality of the experiment was evaluated on the basis of the similarity between replicates by a principal component analysis using the normalized gene expression values as input. The differential expression analysis was performed to identify the genes that are differentially expressed in all comparisons. Only genes with |log2 fold-change| > 0.21 and FDR ≤ 0.05 were considered as DEGs.
To identify the number of different splicing events, the software rMATS (v3.2.5; Shen et al., 2014) was used. Before further analysis, the high-quality reads were aligned against the Arabidopsis genome using Araport11 as reference with the software tool STAR Aligner (v2.5.0c), with the Local Mapping option due to the restrictions in the splicing software. An FDR filter of ≤0.05 was used to detect significant differences in splicing events between Col-0 and drt111. The bioinformatics analysis was performed by Sequentia Biotech (http://www.sequentiabiotech.com). For the DEGs and significantly different splicing events, a GOEA was performed to identify the most enriched GO categories across the down- and upregulated genes (P value < 0.05 and FDR < 0.05) following the method described in Tian et al. (2017).
Accession Numbers
The genes analyzed in this study are: DRT111/SFPS (At1g30480), SUA (At3g54230), PIF1/PIL5 (At2g20180), ABI3 (At3g24650), SF1 (At5g51300), SOM (At1g03790), and phyB (At2g18790).
Supplemental Data
The following supplemental materials are available.
Supplemental Materials and Methods. Additional plant laterial and localization study.
Supplemental Figure S1. DRT111 protein alignment.
Supplemental Figure S2. Subcellular localization of DRT111 protein.
Supplemental Figure S3. Identification of DRT111 knockout mutants and OX plants.
Supplemental Figure S4. Analysis of enriched GO categories and validation of RNA-seq data.
Supplemental Figure S5. Germination of phyB, pif1/pif3/pif4/pif5, pil5-1, and pil5-3, in the presence of ABA.
Supplemental Table S1. List of DEGs in drt111-2 seeds previously identified as ABI3 targets.
Supplemental Table S2. List of primers used in this study.
Supplemental Dataset S1. Genes differentially expressed in drt111-2 seeds and GOEA.
Supplemental Dataset S2. AS events defective in drt111-2 seeds.
Supplemental Dataset S3. GEOA of AS events defective in drt111-2 seeds.
ACKNOWLEDGMENTS
We are thankful to Wim Soppe, Dr. Lucio Conti, and Dr. Eirini Kaiserli for providing seeds. We acknowledge Rosario Nocerino and Gaetano Guarino for excellent technical assistance.
LITERATURE CITED
Author notes
This work was supported by the Italian Ministry of Education, Research and Universities (grant nos. PON02_00395_3215002 and PON02_00395_3082360), the Regione Campania (POR Campania no. FSE 2014/2020 to P.P), and the Ministry of Agricultural, Food and Forestry Policies (Progetto Biotech).
Articles can be viewed without a subscription.
Senior author.
The author responsible for distribution of materials integral to the findings presented in this article in accordance with the policy described in the Instructions for Authors (www.plantphysiol.org) is: Giorgia Batelli ([email protected]).
P.P. performed most of the experiments and analyzed the data; A.R., R.N., M.P., and G.P. performed experiments, acquired, and interpreted experimental results; A.C. supervised the experiments; S.G. supervised the research and complemented the writing; G.B. and G.M. conceived the project, designed the experiments, and analyzed the data; and P.P. and G.B. wrote the article with contributions from all the authors.